Figures & data
Figure 1. (A) Standard iMQC pulse sequence. The top pulse sequence shows the initial CRAZED sequence, while the bottom figure includes a spin echo detection to compensate for dephasing. (B) Energy level diagram for 2-spin system. The iMQC transitions are 2-spin transitions in which both spins flip in the same way (αα to ββ, or up-up to down-down) to create an iDQC, or in opposite directions (αβ to βα or up-down to down-up) to create an iZQC. (C) Refocusing effect of the dipolar field created by the gradient and the mixing pulse on the dephased transverse magnetisation. The 90° pulse puts the magnetisation into the plane. The gradient spatially modulates the transverse magnetisation, and the mixing pulse tilts some portion of the modulated magnetisation back along the z-axis. The modulated longitudinal magnetisation interacts with the transverse magnetisation causing a signal refocusing at a later time.
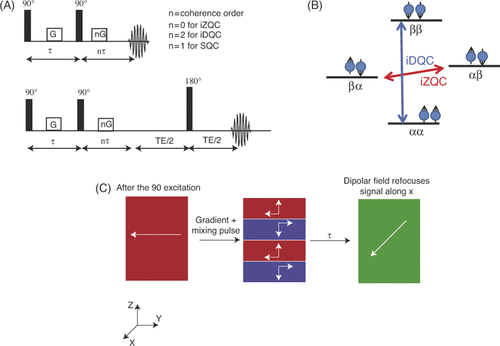
Table I. Phase cycling for two-window HOT.
Figure 2. (A) HOT pulse sequence Citation[32], Citation[51]. The HOT sequence is used to detect temperatures using iMQCs. Two coherence pathways are preserved, in which the initial evolution is iDQC which is converted to iZQC, and the second is one in which the magnetisation is initially iZQC and is converted to iDQC. The separate pathways evolve for different amounts of time allowing for fast acquisition of iMQC temperatre maps. (B) Left: Representative signal evolution obtained during a HOT experiment. In this case, t1 was incremented 48 times. As t1 changes, the phase of the signal changes, and the rate of that change can be used to determine the iZQC frequency. In this figure, each vertex represents the acquisition of a t1 increment, and the evolution of the phase of the signal is shown. Right: If the phase evolution shown on the left is unwrapped and plotted versus t1 (time) the slope of the resulting line can be used to extract the iZQC frequency. The lines on the right show the phase for every pixel in an HOT temperature experiment. The slope of the line is determined using a linear least squares fitting of the t1 time versus phase data points.
![Figure 2. (A) HOT pulse sequence Citation[32], Citation[51]. The HOT sequence is used to detect temperatures using iMQCs. Two coherence pathways are preserved, in which the initial evolution is iDQC which is converted to iZQC, and the second is one in which the magnetisation is initially iZQC and is converted to iDQC. The separate pathways evolve for different amounts of time allowing for fast acquisition of iMQC temperatre maps. (B) Left: Representative signal evolution obtained during a HOT experiment. In this case, t1 was incremented 48 times. As t1 changes, the phase of the signal changes, and the rate of that change can be used to determine the iZQC frequency. In this figure, each vertex represents the acquisition of a t1 increment, and the evolution of the phase of the signal is shown. Right: If the phase evolution shown on the left is unwrapped and plotted versus t1 (time) the slope of the resulting line can be used to extract the iZQC frequency. The lines on the right show the phase for every pixel in an HOT temperature experiment. The slope of the line is determined using a linear least squares fitting of the t1 time versus phase data points.](/cms/asset/79419a78-5546-4ac2-9e72-d013b1c8b34c/ihyt_a_499527_f0002_b.gif)
Figure 3. Demonstration of HOT sequence in cream phantom at three temperatures Citation[32]. The conventional maps were taken by monitoring the changes in phase of the water signal in a phantom of cream (homogeneous mixture of water and fat) as the sample was heated. Large distortions in the detected temperature were observed (due both to shimming imperfections and to susceptibility gradients created during heating), complicating the temperature detection. The images collected using the HOT sequence show only one temperature across the images, demonstrating the clean temperature detection of the HOT sequence. Next to both sets of images are the 90% confidence intervals showing the quality of the fit of each voxel. TE (echo time) = 60 ms, TR (repetition time) = 5 s, τ = 2.67 ms, t1 = 3 to 11 ms, indirect spectral width = 5000 Hz, correlation distance = 140 µm, voxel size = 0.0625 cm3.
![Figure 3. Demonstration of HOT sequence in cream phantom at three temperatures Citation[32]. The conventional maps were taken by monitoring the changes in phase of the water signal in a phantom of cream (homogeneous mixture of water and fat) as the sample was heated. Large distortions in the detected temperature were observed (due both to shimming imperfections and to susceptibility gradients created during heating), complicating the temperature detection. The images collected using the HOT sequence show only one temperature across the images, demonstrating the clean temperature detection of the HOT sequence. Next to both sets of images are the 90% confidence intervals showing the quality of the fit of each voxel. TE (echo time) = 60 ms, TR (repetition time) = 5 s, τ = 2.67 ms, t1 = 3 to 11 ms, indirect spectral width = 5000 Hz, correlation distance = 140 µm, voxel size = 0.0625 cm3.](/cms/asset/5d25dced-bf0c-4988-82fc-688e3ad1ce14/ihyt_a_499527_f0003_b.gif)
Figure 4. In vivo demonstration of the HOT sequence Citation[32]. (A) shows the overlay of a 2-minute HOT temperature maps on an anatomical image of a mouse. The uniformity of the voxels is as expected for the natural temperature distribution of a mouse. (B) is of a mouse with a tube of water next to it. The water is heated over the course of the experiment and the heating is observed in the temperature images. TE = 40 ms, TR = 2 s, τ = 10.66 ms, t1 = 3–13 ms, indirect spectral width = 4000 Hz, correlation distance = 0.0945 mm, voxel size = 0.25 cm3.
![Figure 4. In vivo demonstration of the HOT sequence Citation[32]. (A) shows the overlay of a 2-minute HOT temperature maps on an anatomical image of a mouse. The uniformity of the voxels is as expected for the natural temperature distribution of a mouse. (B) is of a mouse with a tube of water next to it. The water is heated over the course of the experiment and the heating is observed in the temperature images. TE = 40 ms, TR = 2 s, τ = 10.66 ms, t1 = 3–13 ms, indirect spectral width = 4000 Hz, correlation distance = 0.0945 mm, voxel size = 0.25 cm3.](/cms/asset/c87b816c-7fc4-483f-a1ae-a13eb246fb1a/ihyt_a_499527_f0004_b.gif)