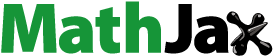
ABSTRACT
We use new paleoseismic data and lidar to reassess late Quaternary activity of the NW (northwest) Cardrona Fault, a ∼60 km long range-bounding fault in Otago. Paleoseismic investigations of the NW Cardrona Fault were conducted in the 1980s, but findings were limited by a paucity of materials suitable for dating. Here, re-exposure and re-assessment of a 1980s trench at Macdonalds Creek provide stratigraphic evidence for two surface rupturing earthquakes on the NW Cardrona Fault. Through Optically Stimulated Luminescence dating and OxCal modelling, we estimate these earthquakes occurred at (mean ± 2 standard deviations, σ) 20.5 ± 7.8 ka and 8.7 ± 6.6 ka. The timings of these earthquakes are consistent with those proposed from re-interpretation of another 1980s trench on the NW Cardrona Fault in the Kawarau River valley, ∼25 km farther south. The slip rate and recurrence interval derived from the Macdonalds Creek trench data are 0.13 ± 0.05 mm/yr and 11,800 ± 5100 years (2σ error) respectively. These estimates imply lower slip rates and longer earthquake recurrence intervals on the NW Cardrona Fault than previously assessed.
Introduction
The NW (northwest) Cardrona Fault is a major range-bounding active reverse fault in western Otago (; Beanland and Barrow-Hurlbert Citation1988; Turnbull Citation2000; Barrell Citation2019). It forms the northeastern component of the Nevis-Cardrona Fault System, a ∼120 km long array of fault strands with more than 1–1.5 km of late Cenozoic west-side-up throw, and which forms the boundary between the Otago range and basin reverse fault province and the Southern Alps tectonic domain (Beanland and Barrow-Hurlbert Citation1988; Warren-Smith et al. Citation2017; Barrell Citation2019; Eberhart-Phillips et al. Citation2022; Seebeck et al. Citation2023).
Figure 1. Map of late Cenozoic faults in the southern South Island as represented by the New Zealand Community Fault Model v1.0 (Seebeck et al. Citation2022, Citation2023), with major faults in the Otago range and basin reverse fault province named in italicised text. Inset, principal components of plate boundary in the South Island (PSZ: Puysegur subduction zone, MFS: Marlborough Fault System) and NUVEL-1a plate motion vector between the Australian (AUS) and Pacific (PAC) plate (DeMets et al. Citation2010). Image underlain by an 80 m digital elevation model.

Prior to this study, constraints on late Quaternary activity of the NW Cardrona Fault were provided by mapping, surveying, and excavation of three paleoseismic trenches in the 1980s (; Beanland Citation1984; Beanland and Fellows Citation1984; Beanland and Barrow-Hurlbert Citation1988). Up to three late Quaternary surface rupturing earthquakes on the NW Cardrona Fault were inferred from these investigations. However, the main technique for dating fault movements at this time was radiocarbon dating and preserved organic carbon is rare in near-surface sediments in much of the Otago region. Hence, rupture timings could only be directly constrained for a trench at Gibbston in the Kawarau River valley near the NW Cardrona Fault’s southern end where organic sediments were found (; Beanland Citation1985; Beanland and Barrow-Hurlbert Citation1988). Subsequently, the development of Optically Stimulated Luminescence (OSL) dating techniques, which target inorganic sandy or silty sediments, has enabled widespread dating of fault movements in the Otago range and basin reverse fault province (Litchfield and Lian Citation2004; Pace et al. Citation2005; Van Dissen et al. Citation2007; Barrell et al. Citation2020; Taylor-Silva et al. Citation2020; Griffin et al. Citation2022a).
Figure 2. Overview of the NW Cardrona Fault. A, Active fault map with previous trench sites, and the vertical separation of terrace surfaces either side of the scarp, highlighted (Beanland and Barrow-Hurlbert Citation1988; Barrell Citation2019; van den Berg Citation2020). Fault mapping and classifications follow Barrell (Citation2019). B, Simplified geological map of the same extent in A from the 1:1,000,000 Geological Map of New Zealand (GNS Science Citation2014). Both maps underlain by New Zealand 8 m digital elevation model. Aerial imagery in A, from LINZ Aerial Imagery Basemap. Coordinates are in NZTM.

In this study we re-examine and, using OSL dating, constrain the chronology of surface rupturing earthquakes on the NW Cardrona Fault from a trench previously excavated on a fault scarp at Macdonalds Creek (previously termed the Branch Creek Road trace, ; Beanland and Barrow-Hurlbert Citation1988). We then place these results in the context of geomorphic analyses from new 1 m horizontal resolution lidar coverage along much of the NW Cardrona Fault’s length (Barrell Citation2019; van den Berg Citation2020), and revised rupture timings along-strike at the Gibbston trench (). This assessment of late Quaternary surface ruptures on the NW Cardrona Fault will provide improved information for seismic hazard assessment around the nearby expanding communities and infrastructure in Queenstown and Wānaka (Mackey Citation2015). In addition, it will contribute to a growing paleoseismic dataset in the Otago range and basin reverse fault province (Van Dissen et al. Citation2007; Barrell et al. Citation2020; Taylor-Silva et al. Citation2020; Meyer Citation2021; Griffin et al. Citation2022a), which is helping to better characterise earthquake behaviour on low slip rate (<∼1 mm/yr) faults in New Zealand and globally.
The NW Cardrona Fault
Geologic setting
The NW Cardrona Fault is mapped for ∼60 km southwest from Lake Wānaka, along the northwest side of the Cardrona valley and the southeastern foot of the Crown Range to a prominent scarp in the Kawarau River valley, and then to Doolans Saddle where it is interpreted to meet the Nevis Fault ( and ; Beanland and Barrow-Hurlbert Citation1988; Turnbull Citation2000; Langridge et al. Citation2016; Barrell Citation2019; Seebeck et al. Citation2023). Within this region, basement rock comprises Mesozoic age Torlesse-affiliated Haast Schist (b; Mortimer Citation1993; Turnbull Citation2000). The contact between the schist basement and overlying Cenozoic cover strata is marked by the Late Cretaceous-early Cenozoic Waipounamu Erosion Surface, an initially flat surface that provides a useful marker for late Cenozoic regional deformation (Stirling Citation1990; Landis et al. Citation2008; Barrell Citation2019).
In the Cardrona valley, Miocene quartz-rich fluvial and lacustrine sedimentary strata of the Manuherikia Group are conformably overlain by a locally-sourced schist gravel formation (Tuohys Breccia; McDonnell and Craw Citation2003). This formation has stratigraphic correlatives in the Nevis (Dell Sandstone and Schoolhouse Fanglomerate; Williams Citation1974; Youngson et al. Citation2002) and Manuherikia valleys (Wedderburn Formation; Youngson et al. Citation1998). The locally sourced angular clasts indicate that these formations were deposited during the Late Miocene-Early Pliocene onset of reverse faulting and uplift of fault-bounded ranges in Otago, including the Nevis-Cardrona Fault System (Beanland and Barrow-Hurlbert Citation1988; Youngson et al. Citation1998; McDonnell and Craw Citation2003).
The Tuohys Breccia is conformably overlain by the Pliocene-age Waiorau Conglomerate. This formation contains well-rounded greywacke clasts whose geochemistry implies an unmetamorphosed Torlesse Terrane source (McDonnell and Craw Citation2003). Quaternary-age poorly consolidated predominantly fluvial sediments unconformably overlie the Waiorau Conglomerate and other older geologic units under the margins and floor of the Cardrona valley (; Beanland and Barrow-Hurlbert Citation1988; Turnbull Citation2000; McDonnell and Craw Citation2003). At the Macdonalds Creek trench site described in this paper, local bedrock comprises Manuherikia Group and/or Tuohys Breccia, overlain by a veneer of Quaternary sediments (Turnbull Citation2000).
Regional neotectonic setting
The NW Cardrona Fault is one of a series of reverse faults that collectively define the Otago range and basin reverse fault province (; McSaveney and Stirling Citation1992). The range and basin topography of this reverse fault province reflects late Cenozoic crustal shortening across a 200-km-wide outboard zone of the Australia-Pacific plate boundary (Beanland and Berryman Citation1989; Norris et al. Citation1990). Geodetic measurements indicate that 1–3 mm/yr of Australian-Pacific plate motion is accommodated within this reverse fault province, with most (70–90%) of the plate motion accommodated to the west on the Alpine Fault (Norris and Cooper Citation2001; Beavan et al. Citation2016; Denys et al. Citation2016). This is consistent with low rates of seismicity in Otago (Warren-Smith et al. Citation2017; Todd et al. Citation2020; Eberhart-Phillips et al. Citation2022) and estimated slip rates on individual faults on the order of 0.1–1 mm/yr (Litchfield and Norris Citation2000; Barrell Citation2019; Taylor-Silva et al. Citation2020; Seebeck et al. Citation2022; Griffin et al. Citation2022a, Citation2022b).
Based on the difference in elevation between basement rock under the Cardrona valley and at the summits of the ranges to the northwest of the NW Cardrona Fault, the late Cenozoic fault throw is at least 1–1.5 km. Along the southeastern side of the Cardrona valley is the Paddys Ridge Fault (previously termed the SE Cardrona Fault) which, together with the Pisa Fault farther southeast, has uplifted the Pisa Range through a combination of anticlinal folding and faulting ( and ; Beanland and Berryman Citation1989). The Paddys Ridge Fault has no known evidence for late Quaternary displacement (; Beanland and Barrow-Hurlbert Citation1988; Barrell Citation2019).
Like other range-bounding faults in the Otago range and basin reverse fault province, the NW Cardrona Fault comprises multiple strands (Beanland et al. Citation1986; Beanland and Barrow-Hurlbert Citation1988; Beanland and Berryman Citation1989; Barrell Citation2019). At the base of its hanging wall ranges is a northwest-dipping master fault strand that is presumed to have accommodated the majority of displacement. Basinward of this master strand, subsidiary NW Cardrona Fault strands are identified from discontinuous (< 1 km long) basin-facing (i.e. synthetic) or range-facing (i.e. antithetic) scarps on mid-level alluvial fans and fluvial terrace surfaces (; Beanland and Barrow-Hurlbert Citation1988; Barrell Citation2019; van den Berg Citation2020). These include the antithetic scarp at the Macdonalds Creek trench site, which is part of an aligned series of discontinuous antithetic scarps extending from Boundary Creek northeast for ∼10 km to Spotts Creek (Barrell Citation2019). These were attributed to a fault strand identified as the ‘NW Cardrona Fault – backfault’ by Barrell (Citation2019). Here, we propose a more concise name, the Branch Burn strand, after the name of the catchment that includes Macdonalds Creek.
From their interpretation of a dextrally-offset terrace riser north of Blackmans Creek, Beanland and Barrow-Hurlbert (Citation1988) inferred the NW Cardrona Fault also accommodates a component of strike-slip displacement. However, lidar indicates that this interpretation is non-unique as it is plausible that fluvial erosion has caused the change in the terrace riser’s trend at the location of the fault, which has created an impression of a lateral offset (Barrell Citation2019). Since there is no other evidence for lateral offsets along the NW Cardrona Fault, it is interpreted here to exhibit reverse slip.
Previous paleoseismic investigations
Paleoseismic trenches were excavated at three locations along the NW Cardrona Fault in 1984 for seismic hazard assessment of proposed hydroelectric developments along the Kawarau and Clutha rivers. The trenches were excavated by bulldozers (‘dozer cuts’ – DC), and were originally named DC12, DC11 and DC10. However, for descriptive simplicity, we refer to them here as the Gibbston, Māori Gully, and Macdonalds Creek trenches (A). We also note that the NW Cardrona fault scarp at the Macdonalds Creek trench locality was previously referred to as the ‘Branch Creek Road’ trace; however, since this name no longer appears on topographic maps, we have renamed it as the Macdonalds Creek scarp. Trenching results from 1984 were initially described in three preliminary unpublished reports (Beanland Citation1984, Citation1985; Beanland and Fellows Citation1984) and then later synthesised together with paleoseimic investigations on the Nevis Fault, to provide an assessment of late Quaternary activity along the Nevis-Cardrona Fault System (Beanland and Barrow-Hurlbert Citation1988).
Beanland and Barrow-Hurlbert (Citation1988) identified evidence for three surface rupturing earthquakes in the Gibbston trench exposure, with their timings constrained by two radiocarbon dates from organic sediments in the trench exposure. These dates were originally reported as conventional radiocarbon ages; however, we report them here after calibration to SHCal20 (; Hogg et al. Citation2020). This implies an antepenultimate rupture sometime before 24,695 ± 2460 calendar years before present (BP), a penultimate rupture between 24,695 ± 2460 and 11,234 ± 1499 calendar years BP, and a subsequent rupture sometime after 11,234 ± 1499 calendar years BP.
Table 1. Radiocarbon ages for samples collected from the Gibbston trench across the NW Cardrona Fault (Beanland Citation1985; Beanland and Barrow-Hurlbert Citation1988).
From the trench across the Branch Burn strand at Macdonalds Creek (Figure S1), Beanland and Barrow-Hurlbert (Citation1988) inferred evidence for three surface ruptures based on differing degrees of deformation of sediments exposed within the trench. The Māori Gully trench ( and S2) revealed a northwest-dipping reverse fault that vertically offset an alluvial fan surface by 2–4 m. The number of ruptures responsible for the observed throw could not be discerned, however, it is likely to be ≥2 (Beanland Citation1984). For both the Māori Gully and Macdonalds Creek trenches, the lack of suitable organic material for radiocarbon dating meant that a rupture chronology could not be determined. Nevertheless, an upper bound for the most recent NW Cardrona Fault surface rupture in the Cardrona valley was inferred by correlating the youngest faulted surface with the youngest fluvial aggradation surface (‘Hawea’ – inferred 18 ka age), which is regarded as widespread throughout the upper Clutha catchment (Beanland Citation1984; Beanland and Barrow-Hurlbert Citation1988; Barrell Citation2019).
Methods
Re-exposure and re-assessment of the Macdonalds Creek trench (44° 49′ 47.63″ S/169° 01′ 33.07″ E) was carried out in February 2019 (van den Berg Citation2020). The trench excavated in 1984 was not subsequently backfilled, however, its walls had become obscured by soil and vegetation in the succeeding 35 years (van den Berg Citation2020). The re-exposure described here therefore involved digging ∼1 m south of what would have been the south wall of the original trench to reveal a new 30 m long exposure (C). It was not practical or safe to re-expose the north wall. The south wall was re-exposed from the ground surface down to the late Cenozoic local bedrock on the hanging wall, a depth of ∼4 m. A smaller pit was excavated in the trench floor to partially re-expose the lower part of the footwall and allow sample collection for dating. The original trench was excavated to ∼6 m depth and exposed the top 1–3 m of the late Cenozoic bedrock. To aid the 2019 trench interpretation, we placed the (scaled) 1984 south wall trench log (Figure S1) on top of the 2019 log and incorporated the lower unit descriptions and extents from the original log into the new log.
The re-exposed trench wall was scraped clean, and unit descriptions and unit boundaries were assessed through a combination of fieldwork and a geometrically rectified (orthomosaic) image of the trench wall. To generate the orthomosaic, multiple photos of the trench were imported into Agisoft Metashape photogrammetry software, where they were aligned, and used to build a 3D polygonal mesh of the trench wall surface onto which an orthomosaic image could be projected. To georeference the orthomosaic, the position of the re-exposed trench wall was surveyed using a Trimble R8s GNSS (Global Navigation Satellite System) receiver. The receiver was set up in RTK (Real-Time Kinematic) mode and data were collected relative to the nearest reference station (ALXD; Alexandra). The markers (corner points of the trench with their associated GPS data) were then manually added to their corresponding location in the 3D mesh in Metashape, which allowed the orthomosaic to be georeferenced (Reitman et al. Citation2015). We then imported the orthomosaic into ArcGIS, which allowed projection of a 1 m2 grid over the trench wall orthomosaic.
To constrain the timing of surface ruptures, nine OSL samples from key stratigraphic units in the Macdonalds Creek trench were submitted to the Victoria University of Wellington Luminescence Dating Laboratory for dating. Samples were prepared using the fine grain (4–11 μm) technique and luminescence ages determined by the Single Aliquot Regenerative (SAR) method. The equivalent dose (paleodose) was calculated using the measurement of broadband luminescence from feldspar when subjected to infrared stimulation. The dose rate was determined based on gamma spectrometry measurements.
The ages and associated uncertainties obtained from the OSL samples were then used to inform Bayesian statistical modelling of surface rupture timings at Macdonalds Creek using OxCal v4.4 (Bronk Ramsey Citation2009; Lienkaemper and Bronk Ramsey Citation2009). In addition, we incorporated the stratigraphic information and radiocarbon ages from the Gibbston trench into OxCal to reassess rupture timings there. In this framework, a ‘sequence’ is developed in which the timings of surface rupturing earthquakes are undated events separated by dating constraints. Except for the penultimate rupture recorded in the Gibbston trench, we do not make any inferences between rupture timing and deposition of the units from which the constraining dates were obtained. We discuss modelling the Gibbston trench’s penultimate rupture timing further in the section ‘A revised interpretation for the Gibbston trench’. OxCal codes are also provided in Supplementary Materials S2.
Macdonalds Creek paleoseismic trench results
Trench site description
The Macdonalds Creek trench was excavated across a well-expressed ∼200 m long NNE-trending range-facing scarp that crosses a fluvial terrace at a high angle immediately south of Macdonalds Creek (‘main terrace’ in ). This scarp is associated with the ∼10 km long east dipping antithetic Branch Burn fault strand, lying about 0.5 km east of the NW Cardrona Fault’s master strand. The main terrace is 30 m higher than the inset modern valley floor of Macdonalds Creek. At the southeast margin of the main terrace is a 20-m riser up to a dissected fluvial terrace remnant (‘high terrace’, ), while to the southwest is dissected hill slope terrain that rises up to ∼70 m above the main terrace. An apron of small colluvial fans lies along the foot of the main terrace’s southern margin (C and ).
Figure 3. Field context of fault trenching site at Macdonalds Creek. A, Northwest-facing photograph looking from the Criffel Range across the Cardrona valley. We highlight here the contrast between the irregular terrain on schist basement that have been uplifted on the NW Cardrona Fault master strand’s hanging wall (background), and the more subdued landscape of the Cardrona valley floor that is developed on Cenozoic cover rock and Quaternary sediments (foreground). B, The Macdonalds Creek fault scarp across the main terrace. View to the north. C, Macdonalds Creek trench looking towards the southeast margin of the main terrace and the riser up to the high terrace with colluvial fans near the foot of the riser. View to the southeast.

Figure 4. Macdonalds Creek trench site in A, unannotated lidar hillshade digital elevation model, and B, interpretive geomorphic map of the main landform elements, and profile locations in . Coordinates in A, are in NZTM 2000 and map extent also shown in A. Topographic contour intervals in B are 1 m, with indicated elevations also in metres.

Figure 5. Vertically exaggerated topographic profiles from lidar data along A, Macdonalds Creek active channel and B–E, the main terrace on which the trenches was excavated on (B). The profiles in B–E were constructed by considering a B–D 20 m, or E 10 m, wide swath of elevation data centred on the profile lines in B, with the black line and grey shading respectively indicating the mean and standard deviation of topography across the swath (Schwanghart and Scherler Citation2014). In B–D, the dashed blue lines are regression surfaces representing the terrace gradient either side of the scarp. The vertical separation of the terrace surfaces is measured from the projected offset of the regression surfaces at the midpoint of the scarp. The surfaces either side of the scarp in E, do not have the same gradient. The scarp height, as indicated by the red horizontal regression lines, is therefore measured instead.

In detail, the main terrace is a composite surface separated by two subtle ∼1 m high terrace risers ( and ). These minor surfaces on the main terrace step up to the south. Topographic profiles constructed using lidar data indicate that the vertical separation of the main terrace across the scarp (3.5–3.9 m) is approximately the same across each minor surface (B–D). All three terrace surfaces on the footwall have uniform gradients all the way to the scarp indicating that there has been minimal accumulation of sediment adjacent to the scarp (). The trench is located on the highest (and morphologically oldest) surface, near the base of the main terrace’s southern margin (C and ). Colluvial fan sediments are therefore widespread around the trench site, where they have preferentially accumulated in the lee of the scarp and have obscured some of the fault displacement (scarp height ∼2 m at the trench site vs 3–5 m to the north, ). In places, the ground near the trench has also been slightly modified by anthropogenic activity.
Topographic profiles indicate that the terrace gradient, expressed as slope angle, on the downstream side/hanging wall of the scarp (2.2° ± 0.1°) is steeper than on the upstream/footwall side (1.6°) and the active channel of Macdonalds Creek (1.4°, ). Assuming that the terrace gradient at the time of formation was approximately the same as the Macdonalds Creek’s modern channel, this suggests that the scarp’s hanging wall has been tilted to the east.
Trench stratigraphy
The oldest unit present in the 2019 Macdonalds Creek trench wall re-exposure is a massive, clayey silt with some gravel (Unit 4, and ). The 1984 trench revealed a greater extent of Unit 4 (Figure S1), where it was documented to comprise a sequence of steeply-dipping interbedded clay and gravels with angular schist clasts. Beanland and Barrow-Hurlbert (Citation1988) inferred this unit to be the Manuherikia Group; however, the presence of schist clasts in Unit 4 is more in accordance with the Tuohys Breccia of McDonnell and Craw (Citation2003).
Figure 6. Geometrically rectified orthomosaic image of the 2019 Macdonalds Creek trench south wall exposure, with key unit boundaries and faults superimposed. The interpreted trench log, supplemented with the full extent of the original trench wall as logged in 1984, is shown in .

Figure 7. Log of the Macdonalds Creek trench’s south wall, incorporating observations from the 2019 trench (upper units, ) and 1984 trench (lower units with fainter colours from Figure S1; Beanland Citation1984; Beanland and Barrow-Hurlbert Citation1988).

Resting on Unit 4 is a dark grey-blue coarse gravel with pebble to large-cobble-sized clasts (Unit 3c, and ). Above these gravels is a sequence of alternating dark to light brown laminar sand and silt (Unit 3b) and yellow-grey moderately interbedded silty gravel to gravelly silt with pebble-sized clasts (Unit 3a). Collectively, we interpret Unit 3 to represent stream alluvium deposited by Macdonalds Creek.
The overlying Unit 2 contains at its base a mottled grey-orange silt (Unit 2c) and is overlain by a pale grey-brown silt with pebble-sized clasts (Unit 2b). There is brown staining within the base of footwall Unit 2c; however, this is not observed in the hanging wall (). We consider this a consequence of it forming as a scarp draping unit (see next section), and we are confident the correlation of Unit 2c across the fault is robust. Above Unit 2b is a similar unit composed of pale-grey-brown silt but with cobble-sized clasts (Unit 2a, and ). We interpret Units 2c-b as colluvial fan deposits sourced from the adjacent slope (C and ). Unit 2a is interpreted to include scarp-derived colluvium, and further colluvial fan deposits from the slope behind. In comparison to the 1984 trench logs, the 2019 trench re-exposure shows a greater Unit 2 thickness ( and S1). This is indicative of the fan deposits thinning down-dip to the north, particularly in the hanging wall.
The Unit 2 sequence is capped by organic silt (Unit 1, and ) which is uniformly 0.4 m thick across the fault and interpreted as topsoil. It is yellow-grey to the east of the fault and brownish-grey to the west (). This variation is attributed to different moisture conditions and soil development at the foot of the scarp. Preserved locally on the footwall above Unit 1 is a structureless pale silt (Unit 0, and ). This unit is interpreted to be human modified or disturbed ground. A zone of structureless gravels is also observed along the fault (Mixed Zone, MZ; and ). Its position localised and adjacent to the main fault indicates it is sourced from adjacent sediments that were reworked and entrained along the fault during rupture.
Surface rupture interpretation
We interpret the sequence of surface rupturing earthquakes that is represented in the Macdonalds Creek stratigraphic record by considering a simple retro-deformation model (). In this schematic model, which combines observations from both the 1984 and 2019 trench exposures, the unit thicknesses, terrace surface dip, fault dip, and incremental offsets are scaled, but small-scale lateral variations in unit thickness are not included. A key constraint for this model is that all three minor surfaces that constitute the main terrace that the Macdonalds Creek trench was excavated on exhibit approximately the same vertical separation across the scarp (3.5–4.0 m, and , see Section ‘Trench Site Description’). This implies that no surface rupture occurred during the formation of the main terrace surface. This is consistent with minimal sediment accumulation at the foot of the upstream-facing scarp. Furthermore, in the trench exposure, Unit 3 exhibits a relatively uniform thickness on either side of the fault, and the unit’s vertical offset (∼3.5–4 m, , ) is broadly consistent with the scarp’s vertical separation of the main terrace surface. Unit 3c has a minor difference (<0.5 m) in thickness on either side of the fault, but we suggest that it is readily explained as a bar to channel fluvial relief on the paleo-bed of the stream. We therefore consider that no surface rupture occurred at the trench locality during Unit 3 alluvial deposition, and that the scarp must have formed after stream action on the terrace had ceased (A).
Figure 8. Schematic retro-deformation model for the sequence of surface rupturing earthquakes at the Macdonalds Creek trench. Unit thicknesses, offsets, and the dips of the fault and units are scaled. However, some small-scale lateral variation in unit thicknesses are not included. Syn-rupture deformation associated with the most recent surface rupture is shown progressively in two steps in parts D and E, MZ; mixed zone.

Table 2. Stratigraphic unit thicknesses from the Macdonalds Creek trench.
Stratigraphic evidence from the trench indicates that the scarp is the product of more than one surface rupture (). The interpretation of a penultimate rupture is based on lateral variations in the thickness of Unit 2c silt, which is 0.7 ± 0.3 m and 1.65 ± 0.15 m thick in its hanging wall and footwall respectively (reported uncertainties represent lateral variations in unit thickness and measurement uncertainties; ). In addition, the higher degree of folding in the footwall of Unit 3 relative to Unit 2c is consistent with the former having accommodated more fault displacement ( and ). We therefore interpret the hanging wall thinning of Unit 2c across the fault as depositional onlap of fan colluvium against a scarp that formed between deposition of Units 3a and 2c (B&C), with the onlapping Unit 2c sediments sourced from the slope that lies immediately south of the trench (C). The thickness variations in Unit 2c either side of the fault indicates that vertical offset in this rupture was 0.95 ± 0.5 m (). While the variation in Unit 2c thickness could reflect lateral thinning of the deposit near the distal margin of the fan, we consider that the scarp’s proximity to Unit 2c thinning makes sediment onlap against the fault scarp a more likely explanation.
In our retro-deformation model, the remaining fault deformation is accounted for by one subsequent rupture that occurred between deposition of Units 2b and 2a. We interpret that during this rupture, sediments were relatively cohesive, so that as hanging wall sediments were uplifted, they were synchronously folding and rolling over onto the footwall (D&E). This formed a 2.8 m long sub-horizontal contact between the hanging wall and footwall. The mixed zone unit (MZ) is interpreted as a lozenge of disintegrated gravelly sediment that was emplaced as different units of cohesive sediments were juxtaposed and amalgamated together at the ground surface.
The vertical offset for this most recent rupture is estimated to be 3.1 m, based on the position of the upper surface of Unit 2c either side of the fault (, E). We interpret that minor scarp degradation following this rupture partly truncated Units 2b, 2c and 3a in the folded hanging wall and resulted in deposition of colluvial Unit 2a (F). We critically discuss this rupture sequence interpretation further in the Section ‘Surface rupture characteristics of the NW Cardrona Fault at Macdonalds Creek’.
OSL dating results and surface rupture timing
Our observations suggest two surface rupturing earthquakes can be inferred in the Macdonalds Creek trench wall from stratigraphic and structural relationships. To estimate the timings of these ruptures, we incorporated the stratigraphic constraints and OSL dates presented in and into OxCal version 4.4 to develop a probabilistic rupture chronology at this site using Bayesian statistics (Bronk Ramsey Citation2009; Lienkaemper and Bronk Ramsey Citation2009; see also ‘Methods’ section). For each OSL sample, we also describe its stratigraphic context and provide a qualitative assessment of our confidence that the sample returned a reliable age for the stratigraphic unit’s deposition (). In particular, we note that the hanging wall OSL dates from Units 3a and 2c (MC02 and MC03) are younger than their footwall dates (MC07 and MC08, and ). We consider that this reflects partial resetting of the luminesce signatures when the hanging wall was pushed close to the surface during the most recent rupture and subsequent erosional truncation of the hanging wall (D and E, ), and not that the stratigraphic correlations across the fault are incorrect. OSL dates MC02 and MC03 were therefore not included in the OxCal model.
Table 3. Optically Stimulated Luminescence (OSL) data and ages from the Macdonalds Creek trench.
Table 4. Confidence in Optically Stimulated Luminescence (OSL) ages from the Macdonalds Creek trench.
The penultimate surface rupture at Macdonalds Creek (MC-eq2) is interpreted to have occurred between the deposition of Units 3a and 2c, and so is bounded by OSL dates (reported as modelled mean age ± 2 standard deviations, σ, ) MC07 (26.7 ± 4.1 ka) and MC08 (14.2 ± 2.7 ka). Incorporating these constraints into the OxCal model indicates that MC-eq2 occurred at 20.5 ± 7.8 ka (mean age ± 2σ, ).
Figure 9. OxCal model for the Macdonalds Creek trench chronology. Light and dark grey are the probability distributions for the OSL ages original and posterior distributions respectively. Posterior distributions for the timings of NW Cardrona Fault surface rupturing earthquake (MC-eq2 and MC-eq1) are shown in red. White circles represent mean age of distributions, horizontal bars bracket 95.4% of the posterior distribution.

The most recent surface rupture at Macdonalds Creek (MC-eq1) is interpreted to have occurred between deposition of faulted Unit 2b and unfaulted Unit 2a. Given our concerns with the reliability of OSL dates MC02 and MC03, the timing for MC-eq1 is most confidently bounded by OSL dates MC08 (14.2 ± 1.4 ka) and MC12 in Unit 1 (3.3 ± 0.6 ka). Our OxCal model indicates that MC-eq1 occurred at 8.7 ± 6.6 ka ().
A revised interpretation for the Gibbston trench
The Gibbston trench was excavated across a 400 m long, 4–5 m high, N-S-trending NW Cardrona Fault scarp that crosses a fluvial terrace in the Kawarau River valley, ∼25 km south of the Macdonalds Creek trench ( and ; Beanland and Fellows Citation1984; Beanland and Barrow-Hurlbert Citation1988). This scarp is interpreted to represent the master west-dipping strand of the NW Cardrona Fault in the Kawarau River valley. At the trench locality, the fluvial terrace stands ∼55 m above present river level and is onlapped by alluvial and colluvial fans from gullies on the south side of the valley (B).
Figure 10. A, Unannotated and B, annotated lidar hillshade digital elevation model of the NW Cardrona Fault scarp at Gibbston in the valley of the east flowing Kawarau River. Contour intervals in B are 1 m. Coordinates in NZTM. C, Profile across the fault scarp with horizontal lines shown used to measure scarp height. The profile is generated by considering the mean (black line) and standard deviation (grey shading) of elevation extracted from the lidar data in a 50 m wide swathed centred on the profile line in B (Schwanghart and Scherler Citation2014). The westward ground slope illustrates that on both sides of the scarp, the river terrace is overlain by an alluvial fan (as indicated in b). Dashed red lines indicate the horizontal lines used to measure scarp height; the terrace’s vertical separation across the scarp cannot be measure as the terrace slope gradient is not equal either side of the scarp.

The trench log indicates schist bedrock (hanging wall only) overlain by ∼2.5 m of Kawarau River gravels (). These are capped by up to ∼2 m (locally thinned by erosion across the scarp crest) of what was originally described as overbank sands and silts (C; Beanland and Barrow-Hurlbert Citation1988). However, from 1 m-interval elevation contours around the trench site (B), and in particular the upstream sloping terrace surface either side of the scarp (C), we infer that an alluvial fan landform is present across the fault scarp. In this context, we re-interpret the upper fluvial deposits as fan alluvium (C).
Figure 11. A,B, Photos and C, log of the trench excavated at this locality from Beanland and Fellows (Citation1984); also see Beanland and Barrow-Hurlbert (Citation1988). The log has been annotated with our revised stratigraphy for the trench exposure (see text for details).

On the downthrown side of the fault, a sand-silt deposit, interpreted as loess (Beanland and Barrow-Hurlbert Citation1988) contains two organic paleosols (carbonaceous sediments, referred to as buried peaty soils by Beanland Citation1985), one at the base and one in the middle of the sand-silt deposit (C). Organic paleosols are rarely found in South Island loess; paleosol horizons in loess are typically marked instead by greater weathering and distinctive pedogenetic textures within the clastic sediments (Raeside Citation1964; Eden and Hammond Citation2003). This leads us to re-interpret the sand-silt deposit as distal alluvial fan deposits rather than loess.
Beanland and Barrow-Hurlbert (Citation1988) considered that three surface ruptures are represented in the trench exposure. In their event sequence, the antepenultimate rupture formed a scarp against which the lower paleosol accumulated. In contrast, our interpretation that alluvial fan deposits are more widespread than previously thought, with their maximum preserved thickness about the same on either side of the scarp, leads us to propose that the lower paleosol was already widely developed across the fan surface. In this context, the initial rupture created accommodation space in the footwall that allowed the paleosol to be buried and preserved beneath silt accumulation (i.e. the paleosol was deposited before, not after this rupture). The preserved thickness of distal alluvial fan sediments over the buried soil on the footwall (∼2 m, C) implies a ∼2 m high scarp was formed by this rupture.
A penultimate rupture was inferred by Beanland and Barrow-Hurlbert (Citation1988) from the offset of the lower paleosol, and in order to create accommodation space for the deposition of the upper paleosol. An expected result of this proposed rupture is that the lower distal fan unit, which is between the lower and upper paleosol, should be more tightly folded than the upper distal fan unit. However, the trench log shows no discernible difference in folding between these units (C). This result could be accounted for by: (1) deformation is localised within the hanging wall units, or (2) that there was no rupture to form the upper paleosol. We tentatively prefer the latter interpretation as we do not consider the presence of the upper paleosol alone as sufficient evidence for the penultimate rupture proposed by Beanland and Barrow-Hurlbert (Citation1988). This implies that only one subsequent rupture is required to explain the emplacement and folding of the hanging wall units over the footwall distal fan units and paleosols, which was then followed by only minor subsequent colluvial deposition. This deformation style is analogous to our interpretation for the most recent rupture at the Macdonalds Creek trench (D–E).
Using our revised interpretation of two surface rupturing earthquakes, and the two calibrated radiocarbon dates (G01: 24,695 ± 2460 calendar years BP, and G02: 11,234 ± 1,499 calendar years BP, ) obtained from sampling the lower and upper paleosol (bulk samples analysed; Beanland Citation1985; Beanland and Barrow-Hurlbert Citation1988), we developed an OxCal model for the Gibbston Trench (Figure S3). In this sequence, the timing of the penultimate surface rupture (G-eq2) can be constrained by the calibrated radiocarbon dates G01 and G02. However, since we infer that burial and preservation of the lower paleosol was only possible because of this rupture, sample G01 provides a close maximum for its timing. To represent this interpretation in our OxCal model, we applied a zero boundary to positively skew the rupture’s timing probability distribution function (PDF, Figure S3; DuRoss et al. Citation2011). This results in a modelled mean ± 2σ uncertainty timing for G-eq2 of ka ( and Figure S3). The timing for the most recent surface rupturing earthquake (G-eq1) is constrained in our OxCal model by the radiocarbon date from the upper paleosol (G02) and a boundary at 1840 CE to signify that it must have occurred before written historical records began in southern New Zealand This results in a modelled mean and ± 2σ uncertainty for the timing of G-eq1 of
ka (Figure S3). In this case, the rupture timing’s asymmetric PDF is indicative of the historical constraint used to bound its younger age, which biases the timing towards this constraint in OxCal.
Discussion
Significance of the NW Cardrona Fault Branch Burn strand
The Macdonalds Creek trench site lies on the 10 km long Branch Burn antithetic fault strand. Adjacent to this fault strand, no other late Quaternary scarps have been identified on the NW Cardrona Fault, either on the presumed master fault 0.5-2 km across-strike from the Branch Burn strand, or to the west in the Cardrona valley (; Barrell Citation2019; van den Berg Citation2020). Antithetic fault scarps are a common feature of reverse faults in the Otago range and basin reverse fault province, however, unlike the Branch Burn strand, they are typically paired with a synthetic scarp that lies basinward of the master strand (Beanland et al. Citation1986; Beanland and Barrow-Hurlbert Citation1988; Beanland and Berryman Citation1989; Barrell Citation2019).
To account for this observation, Beanland and Barrow-Hurlbert (Citation1988) proposed that coseismic slip in this area had been localised onto bedding planes within the steeply-dipping limb of synclinally-folded late Cenozoic strata under the Cardrona valley. In that context, the Branch Burn strand has formed via triggered slip from ruptures on the NW Cardrona Fault master strand. Conversely, Barrell (Citation2019) suggested that the Branch Burn strand represents a hard-linked ‘pop-up’ over an area where the tip of a basinward synthetic strand of the NW Cardrona Fault has not yet ruptured to the surface (i.e. a blind fault). In either case, (1) the master fault at depth must rupture at least as often as the Branch Burn antithetic strand, and (2) given the available evidence that no scarps have been identified west or east of the Branch Burn strand, we can assume for the remainder of this discussion that the Macdonalds Creek trench site provides a complete paleoseismic record for the most recent ruptures on this section of the NW Cardrona Fault.
Surface rupture characteristics of the NW Cardrona Fault at Macdonalds Creek
Our interpreted deformation history indicates that two late Quaternary surface ruptures are recorded in the Macdonalds Creek trench. This interpretation is supported by a schematic retro-deformation model, which to the first-order, accounts for the relative positions, offsets, and folding of the main stratigraphic units (). Small inconsistencies do exist between the trench log and deformation model; for example, the thicknesses of Unit 2c and 2b in the hanging wall, and the modelled position of the footwall contact between Units 3a and 2c is not in the same place as on the trench log (). However, these may be explained by small (∼0.1–0.4 m) lateral thickness variations in Units 3 and 2 that were not incorporated into .
Surface rupture on steeply-dipping reverse faults, like that exposed in the Macdonalds Creek trench (dip 66°; Beanland and Barrow-Hurlbert Citation1988), typically results in an overhanging scarp and subsequent block collapse (Carretier et al. Citation2002; McCalpin and Carver Citation2009; Chiama et al. Citation2023). However, our deformation history interpretation implies that rather than experiencing block collapse, the hanging wall sediments were able to buckle over the footwall during the most recent rupture (D & E). Indeed, mesoscopic-scale, fault-proximal folding of near-surface sediments is common on New Zealand reverse faults (Barrell et al. Citation2009; Citation2020; Citation2023).
We note that hanging wall mesoscopic anticlinal folding may be expected to form tensional features such as axial grabens and infilled fissures; however, no such features were identified in the Macdonalds Creek trench exposure. Nevertheless, grabens or fissure fills in mesoscopic anticlinally-folded sediments are by no means ubiquitous (e.g. Barrell et al. Citation2023). For example, if the sediments were moist at the time of surface rupture, then it is conceivable that they will be more likely to fold without fracturing than dry sediments. The tendency for the sediments exposed in the Macdonalds Creek trench to deform with negligible fracturing is also illustrated in the south wall of the 1984 trench, where fan alluvium sediment layers (our Unit 3c) in the footwall have been synclinally folded and smeared along the fault (Figure S1a).
Our interpretation that two surface ruptures are represented at Macdonalds Creek trench contrasts with the original three-rupture interpretation for this site (Beanland Citation1984; Beanland and Barrow-Hurlbert Citation1988). To explore this discrepancy, we first highlight that the relative (i.e. stratigraphically-defined) timing of the antepenultimate and penultimate ruptures inferred by Beanland and Barrow-Hurlbert (Citation1988) are consistent with our two rupture interpretation. In particular, Beanland and Barrow-Hurlbert (Citation1988) used differences in the degree of folding between their lower (our Units 3a-b) and upper mixed alluvium (our Unit 2c) units to differentiate an earlier rupture that deformed the lower unit and then a later rupture that deformed, by a lesser amount, the upper unit (Figure S1). The higher degree of footwall folding in Unit 3 relative to Unit 2c is also evident in the 2019 trench ( and ). We also associate this earlier rupture with footwall thickening of Unit 2c (B & C), which is visible in the equivalent upper mixed alluvium in the 1984 trench log too (Figure S1). However, this was not noted by Beanland and Barrow-Hurlbert (Citation1988). This may reflect that their stratigraphic framework did not distinguish between the Unit 3 alluvium and Unit 2 colluvium.
The main difference between our interpretations and those of Beanland and Barrow-Hurlbert (Citation1988) is that they proposed the scarp-derived colluvium/Unit 2a has been faulted in a subsequent surface rupture. This is based on their log of the 1984 trench’s north wall (Figure S1b & c) as there is no evidence for a fault offsetting Unit 2a in the south wall (, , and S1d). The north wall was not re-exposed in 2019, and so we cannot entirely exclude the possibility that there was an additional rupture that faulted scarp-derived colluvium/Unit 2a. However, we note the following problems with this interpretation: (1) there is only a subtle difference between the scarp-derived colluvium (Unit 2a) and the underlying fan colluvium (Unit 2b, Figure S1), and so it is possible that the ‘deformed scarp-derived colluvium’ in the north wall is actually fan colluvium, and (2) though the Beanland (Citation1984) north wall trench log indicates that deformed scarp-derived colluvium is locally preserved in a channel cut into the fan alluvium/Unit 3c hanging wall (Figure S1b&c), we regard it as possible that these sediments instead represent Unit 2 fan colluvium. The interpretation for two ruptures since Unit 2b deposition also implicitly assumes progressive deformation across the hanging wall fold. However, it is generally observed that during subsequent surface ruptures, reverse faults truncate previously formed hanging wall folds and propagate to a higher stratigraphic layer (McCalpin and Carver Citation2009).
In summary, there are challenges to reconstructing the sequence of surface ruptures at Macdonalds Creek as the down-dip thinning of Unit 2 colluvial fan sediments across the trench site leads to considerable variations in the north and south wall’s stratigraphy ( and Figure S1). These challenges are further compounded by scarp degradation and hanging wall erosion, which make it difficult to correlate Unit 2 sediments across the fault. For the reasons outlined above, our preferred interpretation is that two events are recorded in the Macdonalds Creek stratigraphy; however, we do not entirely rule out the original three-event interpretation presented in Beanland and Barrow-Hurlbert (Citation1988).
Constraints on NW Cardrona Fault activity at Macdonalds Creek
The deformation history in implies that the interevent displacement variability in the Macdonalds Creek trench (two surface ruptures with 1 and 3 m throw) is higher than observed in other paleoseismic studies from the Otago range and basin reverse fault province (Taylor-Silva et al. Citation2020; Griffin et al. Citation2022a) and elsewhere (Schwartz and Coppersmith Citation1984; Hecker et al. Citation2013). However, we do not attach much significance to this inference, as with only two recorded ruptures from one site on the NW Cardrona Fault, we cannot exclude the possibility that this represents stochastic variability in surface rupture displacement at a single point. Furthermore, the vertical separation of scarps across the lowest (i.e. geomorphically youngest) terrace surfaces elsewhere in the Cardrona valley is typically ∼ 2 m (e.g. Pringles Creek, Back Creek, Spotts Creek; Barrell Citation2019; van den Berg Citation2020), which places a maximum constraint on the throw of the most recent NW Cardrona Fault surface rupture at these localities. In this context, the 3 m throw interpreted for the most recent surface rupture at Macdonalds Creek is not necessarily representative of the causative earthquake’s surface offset.
Projecting the 4.0 m offset of the Unit 3–4 contact through a near-surface 66° fault dip (Beanland and Barrow-Hurlbert Citation1988) gives a total dip-slip displacement at the Macdonalds Creek trench site of 4.4 m. Taking the maximum (OSL date MC04: 49.6 ± 3.8 ka) and minimum (OSL date MC07: 26.7 ± 2.1 ka) age for the units that have experienced both of the two ruptures represented in the trench (and hence the 4.4 m of displacement) we obtain an indicative late Quaternary average dip-slip rate for the Macdonalds Creek site of 0.13 ± 0.05 mm/yr. We consider this to be a representative net slip rate for the NW Cardrona Fault in the Cardrona valley under the assumptions that it accommodates pure dip-slip and that on this portion of the fault, the Branch Burn strand has localised the most recent surface deformation.
For comparison, the slip rate of a moderately dipping (44°) NW Cardrona Fault synthetic strand, as obtained from the Gibbston trench, is ∼0.2 mm/yr (Barrell Citation2019). Notably, the previous estimated net slip rate for the NW Cardrona Fault in the Cardrona valley was mm/yr (Stirling et al. Citation2012; Litchfield et al. Citation2013; Citation2014; Barrell Citation2019; Seebeck et al. Citation2022, Citation2023). The lower slip rates we obtain in this study reflect that: (1) previous net slip rate estimates incorporated a strike-slip component on the NW Cardrona Fault, while we regard that the evidence for lateral offsets on this fault is equivocal (Barrell Citation2019), and (2) previous estimates had assumed an 18 ka surface age for the main terrace at Macdonalds Creek. Conversely, the OSL dates from Unit 3 alluvial sediments and OxCal model imply that this terrace was abandoned prior to the 20.5 ± 7.8 ka timing for the penultimate surface rupture (MC-eq2, ).
Following Griffin et al. (Citation2022a), we use the event timing probability distribution functions for the two surface rupturing earthquakes from our OxCal model (20.5 ± 7.8 ka and 8.7 ± 6.6 ka, ) to simulate 10,000 random earthquake chronologies at Macdonalds Creek. From these simulations, we obtain an estimate of 11,800 ± 5,100 years (2σ error) for the one closed recurrence interval at this locality. This is longer than previous estimates of the NW Cardrona Fault recurrence interval (∼5,500–6,500 years; Beanland and Barrow-Hurlbert Citation1988; Stirling et al. Citation2012; Barrell Citation2019), which were based on three earthquakes being inferred at this locality.
Comparison of surface rupturing earthquake timings on the NW Cardrona Fault from the Macdonalds Creek and Gibbston trench records
The trench records from Macdonalds Creek and Gibbston provide an opportunity to assess if surface rupturing earthquakes on the NW Cardrona Fault have been able to propagate between these sites. We do this using the Combine function in OxCal, so that for all possible rupture timing PDF combinations between the two trenches, we derive: (1) a chi-square statistic (χ2) that the two PDFs are statistically distinct (at a 95% significance level), and (2) the agreement indices (Acomb) for the posterior individual and combined PDF (Bronk Ramsey Citation2009; DuRoss et al. Citation2011; Howarth et al. Citation2021). This analysis is performed for the two rupture interpretations at both the Macdonalds Creek and Gibbston trenches ( and S3). While acknowledging a possibility of three-rupture interpretations at both trenches, which would introduce other combinations of inter-trench rupture correlations, we consider that the two-rupture scenario best accommodates the available trench stratigraphic evidence.
From this analysis, only rupture pairs MC-eq2 - G-eq2, and MC-eq1 - G-eq1 can be correlated (, ). It is also possible that ruptures at these trench localities occurred independently or were distinct but temporally clustered events. Nevertheless, our preferred interpretation is that ruptures between the trench localities correlate with each other since (1) there are no surface geometrical discontinuities (e.g. bends and steps) along the NW Cardrona Fault between the Gibbston and Macdonalds Creek localities () that may act as rupture barriers (Biasi and Wesnousky Citation2016; Biasi and Wesnousky Citation2017), and (2) the single event displacement estimates at each trench (1-3 m) imply rupture lengths (∼30-100 km using the shallow crust reverse fault scaling of Thingbaijam et al. Citation2017) that are consistent with coeval rupture at the two sites. If true, then from combining the rupture timing PDFs from both sites, the mean ages (± 2σ) of the penultimate and most recent ruptures on the NW Cardrona Fault are ka and
ka respectively.
Figure 12. Probability distributions for the timings of surface rupturing earthquakes on the NW Cardrona Fault from OxCal modelling of stratigraphy and dating constraints in A, the Gibbston trench (Figure S3; Beanland and Barrow-Hurlbert Citation1988) and B, the Macdonalds Creek trench ().

Table 5. Statistical tests to determine if the timings of surface rupturing earthquakes from the Macdonalds Creek () OxCal model can be correlated with the ages from the Gibbston OxCal model (Figure S3).
Conclusions
Through re-exposure and re-assessment of a paleoseismic trench at Macdonalds Creek, OSL dating, and OxCal modelling, we provide improved chronostratigraphic constraints for late Quaternary surface rupturing earthquakes on the NW Cardrona Fault in the Otago range and basin reverse fault province. The trench site at Macdonalds Creek is located on the east-dipping antithetic Branch Burn strand of the NW Cardrona Fault, and an absence of identified late Quaternary scarps east or west of this strand suggests it has accommodated the most recent NW Cardrona Fault surface ruptures.
At the Macdonalds Creek trench site, we document evidence for two surface ruptures, a penultimate rupture with ∼1 m throw at 20.5 ± 7.8 ka (mean ±2σ), and the most recent rupture with ∼3 m throw at 8.7 ± 6.6 ka. Our trench interpretation implies a NW Cardrona Fault slip rate of 0.13 ± 0.05 mm/yr and a recurrence interval for surface rupturing earthquakes of 11,800 ± 5,100 years (2σ error), albeit we have only constrained rupture timings for one closed recurrence interval.
Our new data and revised trench interpretations imply lesser fault slip rates and longer earthquake recurrence intervals along the NW Cardrona Fault than earlier studies (Beanland and Barrow-Hurlbert Citation1988), where the lack of available dating techniques precluded the ability to constrain rupture timings in the Cardrona valley and a three-event interpretation for the trench was proposed. Given our two-event interpretation, the timing of the penultimate and most recent surface ruptures at Macdonalds Creek can be correlated to rupture timings 25 km along-strike to the south at Gibbston in the Kawarau River valley.
Acknowledgements
We would like to express our gratitude to Ray Anderson and Hamish and Anna Mackay for allowing land access for fieldwork. Stephen Read kindly assisted with the use of ArcGIS and Agisoft Metashape software assistance. Contact Energy Ltd. is thanked for logistical support, digger hire, and for providing a one-year stipend for the lead author’s thesis, which is the basis for this paper. A University of Otago publishing bursary enabled the first author to prepare this paper, and the university also supported some of the OSL dating costs. Jonathan Griffin publishes with the permission of the CEO, Geoscience Australia. Two anonymous reviewers are thanked for their constructive comments which helped us to improve the manuscript.
Disclosure statement
No potential conflict of interest was reported by the author(s).
Data availability statement
Supplemental files S1 (Figures S1-S3) and S2 (Macdonalds Creek and Gibbston OxCal model code) are available at https://doi.org/10.5281/zenodo.10403626. The Cardrona valley lidar (https://data.linz.govt.nz/layer/99123-otago-lidar-1m-dem-2016/ last accessed August 11th 2023) in , , 10, and S2, New Zealand 8 m digital elevation model in (https://data.linz.govt.nz/layer/51768-nz-8m-digital-elevation-model-2012 last accessed August 11th 2023), and the aerial imagery in (https://www.linz.govt.nz/data/linz-data/aerial-imagery last accessed August 11th 2023) were sourced from Toitū Te Whenua Land Information New Zealand, and licensed by Toitū Te Whenua Land Information New Zealand for re-use under the Creative Commons Attribution 4.0 International licence. The New Zealand 80 m digital elevation model in was sourced from Koordinates (https://koordinates.com/layer/1418-nz-80m-digital-elevation-model/ last accessed August 11th 2023) and licenced under a Creative Commons Attribution 3.0 International licence. A laboratory report on the Macdonalds Creek trench OSL samples and a high-resolution unannotated version of are included in the digital appendices of van den Berg (Citation2020) (http://hdl.handle.net/10523/10474 last accessed August 11th 2023).
Correction Statement
This article has been corrected with minor changes. These changes do not impact the academic content of the article.
Additional information
Funding
References
- Barrell DJA. 2019. General distribution and characteristics of active faults and folds in the Queenstown Lakes and Central Otago districts, Otago. Lower Hutt (NZ). GNS Science Consultancy Report 2018/207. 99 p. https://www.orc.govt.nz/media/6621/gns_cr2018-207_queenstown-lakes-and-central-otago_active-faults.pdf.
- Barrell DJA, Litchfield NJ, Van Dissen RJ, Wang N, Taylor-Silva BI, Hornblow S, Stirling MW. 2020. Investigation of past earthquakes on the Titri Fault, coastal Otago, New Zealand, Lower Hutt (NZ). GNS Science Report 2017/35. 66 p. doi:10.21420/G2TW6S.
- Barrell DJA, Read SAL, Van Dissen RJ, Macfarlane DF, Walker J, Rieser U. 2009. Aviemore: a dam of two halves. In: Geological Society of New Zealand & New Zealand Geophysical Society Joint Annual Conference: Field Trip Guides. Geological Society of New Zealand Miscellaneous Publication 128B.
- Barrell DJA, Stirling MW, Williams JN, Sauer KM, van den Berg EJ. 2023. Hundalee Fault, North Canterbury, New Zealand: late quaternary activity and regional tectonics. New Zealand Journal of Geology and Geophysics. 66(2):293–316. doi:10.1080/00288306.2022.2153877.
- Beanland S. 1984. Late quaternary faulting in the cardrona valley, central otago. Lower Hutt (NZ), GNS Science, New Zealand Geological Survey Immediate Report EDS 84/017 (unpublished file report).
- Beanland S. 1985. Reassessment of late Quaternary fault history using new radiocarbon dates, Kawarau trace, Central Otago. GNS Science, Lower Hutt, (NZ) New Zealand Geological Survey Immediate Report EDS 85/007 (unpublished file report).
- Beanland S, Barrow-Hurlbert SA. 1988. The Nevis-Cardrona Fault system, central Otago, New Zealand: late quaternary tectonics and structural development. New Zealand Journal of Geology and Geophysics. 31(3):337–352. doi:10.1080/00288306.1988.10417780.
- Beanland S, Berryman KR. 1989. Style and episodicity of late quaternary activity on the Pisa-grandview fault zone, central Otago, New Zealand. New Zealand Journal of Geology and Geophysics. 32(4):451–461. doi:10.1080/00288306.1989.10427553.
- Beanland S, Berryman KR, Hull AG, Wood PR. 1986. Late quaternary deformation at the Dunstan Fault, central Otago, New Zealand. Royal Society of New Zealand Bulletin. 24:293–306.
- Beanland S, Fellows DL. 1984. Late quaternary tectonic deformation in the Kawarau river area, central Otago. GNS Science, Lower Hutt, (NZ) New Zealand Geological Survey Immediate Report EDS 84/019 (unpublished file report).
- Beavan J, Wallace LM, Palmer N, Denys P, Ellis S, Fournier N, Hreinsdottir S, Pearson C, Denham M. 2016. New Zealand GPS velocity field: 1995–2013. New Zealand Journal of Geology and Geophysics. 59(1):5–14. doi:10.1080/00288306.2015.1112817.
- Biasi GP, Wesnousky SG. 2016. Steps and gaps in ground ruptures: empirical bounds on rupture propagation. Bulletin of the Seismological Society of America. 106(3):1110–1124. doi:10.1785/0120150175.
- Biasi GP, Wesnousky SG. 2017. Bends and ends of surface ruptures. Bulletin of the Seismological Society of America. 107(6):2543–2560. doi:10.1785/0120160292.
- Bronk Ramsey C. 2009. Bayesian analysis of radiocarbon dates. Radiocarbon. 51(1):337–360. doi:10.1017/S0033822200033865.
- Carretier S, Ritz JF, Jackson J, Bayasgalan A. 2002. Morphological dating of cumulative reverse fault scarps: examples from the Gurvan Bogd Fault system, Mongolia. Geophysical Journal International. 148(2):256–277. doi:10.1046/j.1365-246X.2002.01599.x.
- Chiama K, Chauvin B, Plesch A, Moss R, Shaw JH. 2023. Geomechanical modeling of ground surface deformation associated with thrust and reverse-fault earthquakes: a distinct element approach. Bulletin of the Seismological Society of America. 113(4):1702–1723. doi:10.1785/0120220264.
- DeMets C, Gordon RG, Argus DF. 2010. Geologically current plate motions. Geophysical Journal International. 181(1):1–80. doi:10.1111/j.1365-246X.2009.04491.x.
- Denys P, Pearson C, Norris R, Denham M. 2016. A geodetic study of Otago: results of the central Otago deformation network 2004–2014. New Zealand Journal of Geology and Geophysics. 59(1):147–156. doi:10.1080/00288306.2015.1134592.
- DuRoss CB, Personius SF, Crone AJ, Olig SS, Lund WR. 2011. Integration of paleoseismic data from multiple sites to develop an objective earthquake chronology: application to the Weber segment of the Wasatch Fault zone, Utah. Bulletin of the Seismological Society of America. 101(6):2765–2781. doi:10.1785/0120110102.
- Eberhart-Phillips D, Upton P, Reyners M, Barrell DJA, Fry B, Bourguignon S, Warren-Smith E. 2022. The influence of basement terranes on tectonic deformation: joint earthquake travel-time and ambient noise tomography of the southern of the Southern South Island, New Zealand. Tectonics. 41(4):e2021TC007006. doi:10.1029/2021TC007006.
- Eden DN, Hammond AP. 2003. Dust accumulation in the New Zealand region since the last glacial maximum. Quaternary Science Reviews. 22:2037–2052. doi:10.1016/S0277-3791(03)00168-9.
- GNS Science. 2014. Geological map of New Zealand 1:1,000,000, 2nd ed. [Data set]. Lower Hutt, (NZ): GNS Science. doi:10.21420/VRX8-CD71.
- Griffin JD, Stirling MW, Barrell DJA, van den Berg EJ, Todd EK, Nicolls R, Wang N. 2022a. Paleoseismology of the Hyde Fault, Otago, New Zealand. New Zealand Journal of Geology and Geophysics. 65(4):613–637. doi:10.1080/00288306.2021.1995007.
- Griffin JD, Stirling MW, Wilcken KM, Barrell DJA. 2022b. Late Quaternary slip rates for the Hyde and Dunstan Faults, southern New Zealand: implications for strain migration in a slowly deforming continental plate margin. Tectonics. 41(9):e2022TC007250. doi:10.1029/2022TC007250.
- Hecker S, Abrahamson NA, Wooddell KE. 2013. Variability of displacement at a point: implications for earthquake-size distribution and rupture hazard on faults. Bulletin of the Seismological Society of America. 103(2 A):651–674. doi:10.1785/0120120159.
- Hogg AG, Heaton TJ, Hua Q, Palmer JG, Turney CSM, Southon J, Bayliss A, Blackwell PG, Boswijk G, Bronk Ramsey C, et al. 2020. SHCal20 Southern Hemisphere Calibration, 0-55,000 years cal BP. Radiocarbon. 62(4):759–778. doi:10.1017/RDC.2020.59.
- Howarth JD, Barth NC, Fitzsimons SJ, Richards-Dinger K, Clark KJ, Biasi GP, Cochran UA, Langridge RM, Berryman KR, Sutherland R. 2021. Spatiotemporal clustering of great earthquakes on a transform fault controlled by geometry. Nature Geoscience. 14(5):314–320. doi:10.1038/s41561-021-00721-4.
- Landis CA, Campbell HJ, Begg JG, Mildenhall DC, Paterson AM, Trewick SA. 2008. The Waipounamu erosion surface: questioning the antiquity of the New Zealand land surface and terrestrial fauna and flora. Geological Magazine. 145(2):173–197. doi:10.1017/S0016756807004268.
- Langridge RM, Ries WF, Litchfield NJ, Villamor P, Van Dissen RJ, Barrell DJA, Rattenbury MS, Heron DW, Haubrock S, Townsend DB, et al. 2016. The New Zealand active faults database. New Zealand Journal of Geology and Geophysics. 59(1):86–96. doi:10.1080/00288306.2015.1112818.
- Lienkaemper JJ, Bronk Ramsey C. 2009. OxCal: versatile tool for developing paleoearthquake chronologies- a primer. Seismological Research Letters. 80(3):431–434. doi:10.1785/gssrl.80.3.431.
- Litchfield NJ, Lian O. 2004. Luminescence age estimates of Pleistocene marine terrace and alluvial fan sediments associated with tectonic activity along coastal Otago, New Zealand. New Zealand Journal of Geology and Geophysics. 47(1):29–37. doi:10.1080/00288306.2004.9515035.
- Litchfield NJ, Norris RJ. 2000. Holocene motion on the Akatore Fault, south Otago coast, New Zealand. New Zealand Journal of Geology and Geophysics. 43(3):405–418. doi:10.1080/00288306.2000.9514897.
- Litchfield NJ, Van Dissen R, Sutherland R, Barnes PM, Cox SC, Norris R, Beavan RJ, Langridge R, Villamor P, Berryman K, et al. 2014. A model of active faulting in New Zealand. New Zealand Journal of Geology and Geophysics. 57:32–56. doi:10.1080/00288306.2013.854256.
- Litchfield NJ, Van Dissen RJ, Sutherland R, Barnes PM, Cox SC, Norris RJ, Beavan RJ, Langridge RM, Villamor P, Berryman KR, et al. 2013. A model of active faulting in New Zealand: fault zone parameter descriptions. Lower Hutt, N.Z: GNS Science. GNS Science report 2012/19. p. 120.
- Mackey B. 2015. Seismic hazard in the Queenstown Lakes district. Dunedin, NZ: Otago Regional Council. p. 98. https://www.orc.govt.nz/media/2924/2015-seismic-hazard-queenstown-lakes.pdf.
- McCalpin JP, Carver GA. 2009. Paleoseismology of compressional tectonic environments. International Geophysics. 95:315–419. doi:10.1016/S0074-6142(09)95005-7.
- McDonnell M, Craw D. 2003. Stratigraphy and provenance of Pliocene greywacke-bearing conglomerate, Cardrona valley, Otago, New Zealand. New Zealand Journal of Geology and Geophysics. 46(3):425–436. doi:10.1080/00288306.2003.9515018.
- McSaveney MJ, Stirling MW. 1992. Central Otago: basin and range country. In: Soons JM, Selby MJ, editors. Landforms of New Zealand. Auckland: Longman Paul; p. 482–504.
- Meyer AFT. 2021. A paleoseismic investigation of the long valley fault, Central Otago. Dunedin, NZ: University of Otago. BSC(Hons) Thesis.
- Mortimer N. 1993. Jurassic tectonic history of the Otago Schist, New Zealand. Tectonics. 12(1):237–244. doi:10.1029/92TC01563.
- Norris RJ, Cooper AF. 2001. Late Quaternary slip rates and slip-partitioning on the Alpine Fault, New Zealand. Journal of Structural Geology. 23(2000):507–520. doi:10.1016/S0191-8141(00)00122-X.
- Norris RJ, Koons PO, Cooper AF. 1990. The obliquely-convergent plate boundary in the South Island of New Zealand: implications for ancient collision zones. Journal of Structural Geology. 12(5–6):715–725. doi:10.1016/0191-8141(90)90084-C.
- Pace B, Stirling MW, Litchfield NJ, Rieser U. 2005. New active fault data and seismic hazard estimates for west Otago, New Zealand. New Zealand Journal of Geology and Geophysics. 48(1):75–83. doi:10.1080/00288306.2005.9515099.
- Raeside JD. 1964. Loess deposits of the south island, New Zealand, and soils formed on them. New Zealand Journal of Geology and Geophysics. 7:811–838. doi:10.1080/00288306.1964.10428132.
- Reitman NG, Bennett SEK, Gold RD, Briggs RW, DuRoss CB. 2015. High-resolution trench photomosaics from image-based modeling: workflow and error analysis. Bulletin of the Seismological Society of America. 105(5):2354–2366. doi:https://doi.org/10.1785/0120150041.
- Schwanghart W, Scherler D. 2014. Short Communication: TopoToolbox 2 - MATLAB-based software for topographic analysis and modeling in Earth surface sciences. Earth Surface Dynamics. 2(1):1–7. doi:10.5194/esurf-2-1-2014.
- Schwartz DP, Coppersmith KJ. 1984. Fault behavior and characteristic earthquakes: examples from the Wasatch and San Andreas Fault zones. Journal of Geophysical Research: Solid Earth. 89(B7):5681–5698. doi:10.1029/JB089iB07p05681.
- Seebeck H, Van Dissen RJ, Litchfield N, Barnes PM, Nicol A, Langridge R, Barrell DJA, Villamor P, Ellis S, Rattenbury M, et al. 2023. The New Zealand Community Fault Model–version 1.0: an improved geological foundation for seismic hazard modelling. New Zealand Journal of Geology and Geophysics. 1–21. doi:10.1080/00288306.2023.2181362.
- Seebeck H, Van Dissen RJ, Litchfield NJ, Barnes PM, Nicol A, Langridge RM, Barrell DJA, Villamor P, Ellis SM, Rattenbury MS, et al. 2022. New Zealand community Fault model – version 1.0. Lower Hutt, NZ: GNS Science. GNS Science Report 2021/57. p. 97. doi:10.21420/GA7S-BS61.
- Stirling M, McVerry G, Gerstenberger M, Litchfield N, Van Dissen R, Berryman K, Barnes P, Wallace L, Villamor P, Langridge R, et al. 2012. National seismic hazard model for New Zealand: 2010 update. Bulletin of the Seismological Society of America. 102(4):1514–1542. doi:10.1785/0120110170.
- Stirling MW. 1990. The Old Man range and Garvie mountains: tectonic geomorphology of the central Otago Peneplain, New Zealand. New Zealand Journal of Geology and Geophysics. 33(2):233–243. doi:10.1080/00288306.1990.10425681.
- Taylor-Silva BI, Stirling MW, Litchfield NJ, Griffin JD, van den Berg EJ, Wang N. 2020. Paleoseismology of the Akatore Fault, Otago, New Zealand. New Zealand Journal of Geology and Geophysics. 63(2):151–167. doi:10.1080/00288306.2019.1645706.
- Thingbaijam KKS, Mai PM, Goda K. 2017. New empirical earthquake source-scaling laws. Bulletin of the Seismological Society of America. 107(5):2225–2246. doi:10.1785/0120170017.
- Todd EK, Stirling MW, Fry B, Salichon J, Villamor P. 2020. Characterising microseismicity in a low seismicity region: applications of short-term broadband seismic arrays in Dunedin, New Zealand, New Zealand. New Zealand Journal of Geology and Geophysics. 63(3):331–341. doi:10.1080/00288306.2019.1707238.
- Turnbull IM. 2000. Geology of the Wakatipu area. Institute of Geological & Nuclear Sciences 1:250,000 Geological Map 18. 72 p. and map (1 sheet). Lower Hutt (NZ): GNS Science.
- van den Berg E. 2020. Paleoseismology of the NW Cardrona Fault, Central Otago. University of Otago, Dunedin (NZ). MSC Thesis. http://hdl.handle.net/10523/10474.
- Van Dissen R, Barrell D, Langridge R, Litchfield N, Villamor P, Tonkin P. 2007. Reassessment of seismic hazard at the Clyde Dam, Central Otago: Earthquake geology field investigations and determination of Dunstan Fault rupture characteristics. Lower Hutt (NZ). GNS Science Consultancy Report 2006/147.
- Warren-Smith E, Lamb S, Stern TA, Smith E. 2017. Microseismicity in southern South Island, New Zealand: implications for the mechanism of crustal deformation adjacent to a major continental transform. Journal of Geophysical Research: Solid Earth. 122(11):9208–9227. doi:10.1002/2017JB014732.
- Williams GJ. 1974. Cenozoic geology of the lower Nevis basin, with special reference to shale deposits. Wellington (NZ): Department of Scientific and Industrial Research. DSIR Bulletin. 212:36. and 1 folded map.
- Youngson JH, Craw D, Landis CA, Schmitt KR. 1998. Redefinition and interpretation of late Miocene-Pleistocene terrestrial stratigraphy, Central Otago, New Zealand. New Zealand Journal of Geology and Geophysics. 41(1):51–68. doi:10.1080/00288306.1998.9514790.
- Youngson JH, Wopereis P, Kerr LC, Craw D. 2002. Au-Ag-Hg and Au-Ag alloys in Nokomai and Nevis valley placers, northern Southland and Central Otago, New Zealand, and their implications for placer-source relationships. New Zealand Journal of Geology and Geophysics. 45(1):53–69. doi:10.1080/00288306.2002.9514959.