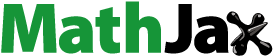
ABSTRACT
Dryland calcicolous plants are drought-tolerant species that are adapted to survive on alkaline low-nutrient bare substrates derived from limestone around the world. Outcrops of the Otekaike Limestone of North Otago host some rare low-growing (<10 cm) endemic calcicoles, and conservation reserves have been created at some localities. The calcicoles occupy microhabitats in solution cavities in limestone outcrops, growing in thin (<10 cm) limestone debris accumulations, including detrital sand and silt. Calcicoles also grow in coarse and fine disaggregated limestone talus deposits at tops and bottoms of outcrops, commonly with the low-growing exotic hawkweed but the calcicoles are displaced by taller species, especially grasses. Calcicole substrates have pH 8 to 9, with a balance between calcite dissolution and calcite re-precipitation. Electrical conductivity is typically <500 µS/cm, but can be as high as 7 mS/cm with evaporative salts and salt weathering enhancing porosity. The limestone contains variably oxidised authigenic glaucony and pyrite, which provide some plant nutrients (K, Mg, Fe, S, N) as roots penetrate porous limestone, with apatite providing phosphate. Persistence of these rare calcicolous communities in soil-free settings is strongly affected by the underlying mineralogy and geochemistry, and some similar microhabitats may occur on other rock types.
Introduction
Alkaline low-nutrient rocky surfaces around the world commonly host calcicolous plants (calcicoles) that are adapted to survive in these chemically challenging environments (Lee Citation1998; Zohlen and Tyler Citation2000; Lauchli and Grattan Citation2012; Wala et al. Citation2020). Many calcicoles, grow on limestone and other calcareous rocks in settings where there is little or no soil, and the plants get their nutrients directly from underlying rock debris (Lee Citation1998; Paul et al. Citation2009; Estrada-Medina et al. Citation2013; Schwinning Citation2013). New Zealand has some endemic calcicoles, and many of these grow on Cenozoic limestones of the South Island (Heenan and Molloy Citation2006, Citation2019; Rogers et al. Citation2018; Heenan and Rogers Citation2019). Several such communities occur on Otekaike Limestone in the drylands of inland Waitaki valley of North Otago (A,B; Heenan and Molloy Citation2006, Citation2019; Rogers et al. Citation2018; Heenan and Rogers Citation2019). These endemic plants are small (<10 cm high) and typically can be found on soil-free substrates or substrates with only primitive soil (herein called proto-soil, with low-moderate organic content), although this may not be their only historical distribution. Their survival is threatened by incursion of taller plants, especially exotic pasture grasses and weeds, and several reserves have been created for their preservation by the New Zealand Department of Conservation (DOC) on public land, and on private land facilitated by Queen Elizabeth II National Trust (QE II), a non-government organisation for conservation.
Figure 1. Location maps and setting for the Otekaike Limestone in the Waitaki valley. A, Digital elevation model of topography (from Geographx. co.nz) of the study region. Inset shows the study region in relation to Otago Schist basement. B, Present exposures of limestones in the region (from Forsyth Citation2001), with the locations of study sites. C, Summary annual rainfall map for the region (adapted from NIWA Citation2023), showing the low-rainfall corridor down the Waitaki valley. D, Oblique view from southeast of the region, contoured for solar irradiation variations (from Solargis; http://globalsolaratlas.info), showing the corridor of high irradiation down the Waitaki valley.
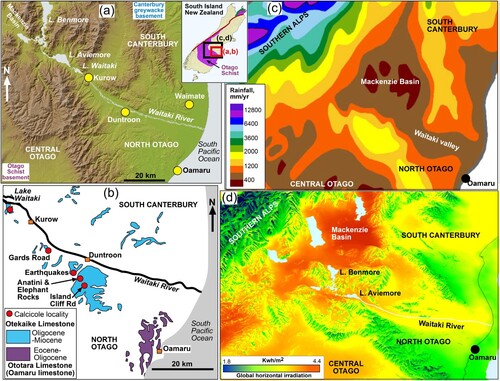
The Otekaike Limestone (Late Oligocene to Early Miocene) is one of the most prominent geological features of North Otago, in the southeastern South Island of New Zealand (A). The limestone forms spectacular steep outcrops throughout the lowlands of the Waitaki valley (A,B; Gage Citation1957; Forsyth Citation2001). In addition, the limestone is well-known for its rich fossil assemblages (e.g. Gage Citation1957; Ayress Citation1993; Graham et al. Citation2000; Tanaka and Fordyce Citation2014). The limestone includes the type section for the formally defined New Zealand Waitakian Stage and the lower part includes the boundary with the underlying Duntroonian Stage (Ayress Citation1993; Graham et al. Citation2000). Preliminary geoecological work at one Otekaike Limestone site, near Lake Waitaki, has been described by Craw et al. (Citation2022) and Rufaut et al. (Citation2023) where the site is named after Kurow, the nearest town (B). The present study extends that work in a more regional context. The limestone, its fossils, and its landforms are principal components of the popular Vanished World tourist attraction (www.vanishedworld.co.nz) within the recently established UNESCO Waitaki Whitestone Geopark (www.whitestonegeopark.nz).
Geological and botanical research projects on various aspects of the Otekaike Limestone have proceeded largely independently of each other over the past 30 years. In this paper, we bring results from these two scientific disciplines closer together. We do this by documenting the sites in which the plants live (microhabitats) in terms of the geological and mineralogical nature of the material (substrates) in which the plants grow. We attempt to answer two specific questions: (a) What is the geological and mineralogical nature of the microhabitats and underlying substrates that host the calcicoles; and (b) how do these calcicoles obtain nutrients and moisture to survive in bare dryland soil-free substrates? This information will provide better context for future conservation-oriented site management. In addition, our study will help to integrate these important and unique calcicolous plant communities into the wider geological setting of the UNESCO Waitaki Whitestone Geopark.
General setting
Geology, landscape and climate
The Otekaike Limestone is part of a regionally extensive Cenozoic marine rock sequence, remnants of which occur in the eastern parts of North Otago and South Canterbury (A,B; Gage Citation1957; Forsyth Citation2001). Pliocene-Holocene uplift and erosion have removed most of this sequence from the mountains and foothills farther inland, where basement rocks are now exposed to form complex ranges and foothills (A; Forsyth Citation2001). The Otekaike Limestone is younger than, and occurs farther inland than, the Ototara Limestone (commonly called Oamaru limestone; B; Gage Citation1957; Forsyth Citation2001). These limestone units are separated stratigraphically by the Oligocene Kokoamu Greensand immediately beneath the Otekaike Limestone (A). The Otekaike Limestone is overlain by marine mudstone, the Waioura Marl (A; Gage Citation1957; Forsyth Citation2001). However, neither the Kokoamu Greensand nor the Waioura Marl are visible in natural outcrops at most Otekaike Limestone localities (e.g. A).
Figure 2. Structure and stratigraphy of the Otekaike Limestone in the Waitaki valley study region (localities in B). A, Sketch cross section through the Anatini site. B, Cliffs at Gards Road site, showing topographic and outcrop distinctions between calcite-rich limestone (bottom) and sand and silt rich limestone (top). C, Sketch cross section through the limestone locality near Lake Waitaki. Legend as in 2a. D, Outcrop appearance with variable sand contents at Lake Waitaki site. E, Lower part of limestone cliff at the Earthquakes site, showing a rare exposure of underlying Kokoamu Greensand.
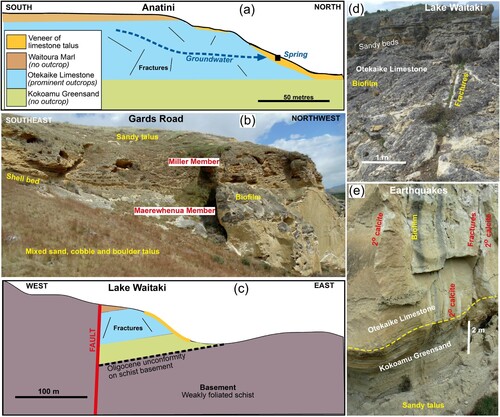
Basement in the area that forms higher topographic areas is Mesozoic Otago Schist on the south side of the Waitaki valley, and Mesozoic greywacke on the north side (A; Forsyth Citation2001). Basement is not exposed beneath the easternmost outcrops of Otekaike Limestone in Waitaki valley where the Cenozoic sequence is flat-lying or has gentle dips (B; A,B). However, farther to the northwest the limestone occurs only as structural and erosional outliers that are isolated by surrounding uplifted basement and Pleistocene fluvial sediments (B; C,D; Forsyth Citation2001). All Otekaike Limestone outcrops have Pleistocene-Holocene erosional talus deposits that coat the steep surrounding slopes (A–C). A major (100 m scale) Pleistocene-Holocene landslide emanated from an Otekaike Limestone scarp at Earthquakes, exposing even steeper cliffs (E).
The area lies in a prominent rain-shadow to the east of the Southern Alps (C). There is a corridor of especially low rainfall and high solar irradiation extending down the Waitaki valley from the more extreme rain shadow of the Mackenzie Basin (C,D). This topographic and climatic setting leads to strongly seasonal climate and weather patterns, although rainfall is generally uniformly distributed throughout the year (Supplementary Figure 1). The lower Waitaki valley receives between 400 and 800 mm/year of rain (C; NIWA Citation2023; Supplementary Figure 1). Summers in the region have high potential evaporation of 5–10 mm/day, with well-defined low-evaporation periods in the winters (Supplementary Figure 1). The climate is cool-temperate, with mean annual temperature of ∼12°C, but summers can be hot, at times with temperatures exceeding 30°C (Supplementary Figure 1). Winter temperatures include some frosts as low as −10°C and winter days are typically cool (5–10°C; Supplementary Figure 1). Summers commonly include periods of drought where high temperatures and high solar radiation leads to high evaporation that exceeds incoming rainfall (Supplementary Figure 1). Early winters (May to June) in many years have low rainfall compared to wetter early summers (December to January; Supplementary Figure 1). Consequently, drought that is driven by the high evaporation in summer and autumn can be extended by this period of low rainfall in early winter.
Calcicolous plants
The calcicolous plants on limestone in the Waitaki valley are apparently adapted to these seasonal extremes and periodic drought conditions. Many of the calcicoles can grow in full sunlight in exposed settings on outcrops and adjacent talus deposits, but most can also tolerate shady locations (Heenan and Rogers Citation2019). Geological isolation of limestone outcrops during Pliocene-Holocene uplift and erosion has led to some botanical speciation between limestone localities (Heenan and McGlone Citation2013; Heenan and Rogers Citation2019). These plant genetic divergences were driven by similar geological uplift processes, on similar time scales, to genetic divergence and speciation of native galaxiid fish in the same Waitaki valley area (Waters and Craw Citation2007). Here, we focus on the overall geoecology of calcicolous plants in general, in their extant microhabitats. Taxonomic and botanical details at the species and sub-species level are covered by e.g. Heenan and Rogers (Citation2019) and references cited therein. We provide species names for the most common calcicoles when they first appear in figures, and genera thereafter.
The chemical reasons why New Zealand calcicoles have apparent preferences for limestone substrates are not known. Calcicole-related chemistry has been investigated more intensively internationally, and some common results are summarised in . For Otekaike Limestone substrates, each calcicolous nutrient parameter in this table is equated with a mineral that occurs within the limestone as a potential controller of that nutrient effect or availability. While these nutrient correlations are speculative without further detailed research on individual species, these general links provide a useful context for a geoecological study of the calcicolous plants on the Otekaike Limestone and may be relevant for other New Zealand Cenozoic limestones which host calcicolous plants (e.g. Heenan and Rogers Citation2019). From these perspectives, this study is the first attempt to link limestone substrate geology and nutrient sources to the endemic plants on limestone in general.
Table 1. Summary of some international observations on substrate chemistry adaptations by calcicoles that facilitate their establishment at the expense of other plants.
Methods
This study reports observations from six limestone sites with calcicolous plants in the Waitaki valley (B). Most observations were made at the Anatini site (A), which is a QE II covenant on private land near Duntroon (B). Elephant Rocks site occurs on adjoining private land. Both these sites have provision for public access as part of the UNESCO Geopark, with focus on limestone landforms and contained fossils. The nearby Island Cliff Road site (B) is a small roadside limestone outcrop managed by Waitaki District Council via a QE II covenant for preservation of calcicoles. Earthquakes site is a DOC reserve, primarily for the landforms associated with the large landslide but also for calcicoles. Gards Road site is a DOC reserve for the contained calcicoles, with active management of calcicole habitat. The Gards Road site is known in the geological literature as ‘Trig Z’ section (B) and is the type locality for the Waitakian Stage, with the Duntroonian-Waitakian stage boundary (Gage Citation1957; Ayress Citation1993; Graham et al. Citation2000). A limestone outlier in hills immediately south of Lake Waitaki (B; C,D) is a QE II covenant on private land dedicated specifically for calcicole and limestone landscape preservation.
Because of the delicacy and ecologically threatened status of the endemic calcicoles, most of our observations were made on surficial features of their substrates, without disturbance. Minor disturbance of substrates, to gain deeper views, was done near to calcicoles in talus at the Lake Waitaki (Craw et al. Citation2022; Rufaut et al. Citation2023) and at the Anatini site. At Anatini, a set of ten substrate samples was extracted from the immediate vicinity of abundant calcicoles (Chaerophyllum) on talus deposits immediately above a limestone outcrop. Care was taken during sampling to avoid individual calcicoles, but the samples taken were representative of the material in which the plants were established nearby. These substrate samples were extracted immediately below (∼10 cm) any surficial vegetation that was mainly scattered Pilosella hawkweed (previously called Hieracium) and associated proto-soil (limestone-rich surficial material with minor organic material). To broaden the range of substrates, samples of turf (organic-rich soil with only minor limestone material) were obtained for comparison to the talus substrates. One turf sample was extracted from a grass-rich zone near a calcicole, Geranium scolateum, from the Elephant Rocks area adjacent to the Anatini covenant.
The ten substrate samples were collected in early summer (December 2023) and all samples contained some natural moisture. Moisture-loss experiments were conducted on these samples by adding sufficient water to further saturate them, and they were then allowed to dry progressively. The aim was to test the absolute and relative times and extents of water loss from the substrates in which calcicoles are most commonly found. About 400–500 grams of each sample were placed in a clear plastic open-topped container, 8 × 8 cm square. This resulted in a substrate depth of c. 6 cm on the bottom of the container, to be representative of a typical surficial layer of bare substrate. To imitate a prolonged rain event, 110 millilitres of water were added to each container to saturate the substrates. The added water soaked into pore spaces, but no extra pooling of water occurred at the surfaces. The gross weight of each container was then weighed to ±0.1 grams. The containers were lined up along a sunny interior windowsill and reweighed daily (with one final two-day exception) for eight weeks until only minor (∼1 g) weight losses were occurring.
Substrate samples were examined with a stereoscopic microscope to observe mineralogy and surficial textures. General chemical characterisation was done on a laboratory bench to determine pH, and also electrical conductivity (EC) as a proxy for salinity. Slurries (20 ml solids, 30 ml distilled water) were agitated into a uniform paste, as in recent studies of halophyte habitats (Rufaut et al. Citation2018, Citation2023; Craw et al. Citation2022). Two slurries were made from each sample, and three from sample Ant 6. Measurements were made with a portable Oakton PC450 meter with separate pH and EC probes, calibrated in laboratory conditions (19°C) with standard solutions (pH 4, 7, 10; EC 1000 µS/cm). Additional information on limestone petrography, mineralogy and geochemistry was extracted from Mortimer and Strong (Citation2014) and historical data therein, mostly from Morgan (Citation1919) and Kitt (Citation1962). Geochemical data on plant nutrient contents of South Island glaucony were summarised from Oze et al. (Citation2019). Other data on plant nutrients for glaucony elsewhere in the world were obtained from Hall and Buckley (Citation1991) and Srodon et al. (Citation2023).
Three centimetre-scale limestone chips from Anatini site were mounted on aluminium stubs and examined by scanning electron microscopy (SEM). One of these chips was from the surface of a limestone outcrop; the other two were from the plant substrate samples (above). SEM observations were made with a Zeiss Sigma VP (variable pressure) FEG-SEM fitted with an Oxford Instruments X-Max 20 mm silicon drift detector at the Otago Micro and Nanoscale Imaging (OMNI), University of Otago. Backscatter electron imaging (BSE) and energy dispersive spectrometry (EDS) analyses were performed at 20 kV accelerating voltage. Samples were not carbon-coated. Instead they were examined in a low-pressure N2 atmosphere to limit electron charging. Semi-quantitative elemental compositions were obtained by spot analyses (∼5–10 µm), with detection limits of ∼0.5 wt%. Rough surfaces on specimens precluded more precise measurements.
Relative element distributions were mapped with automated EDS. Brighter areas on these maps presented herein represent relatively high element concentrations, and sparsely coloured areas are background and dispersed signals near to the detection limit. The darkest areas are pore spaces and surface relief facing away from the electron source. Map colours are arbitrary and are merely a way of distinguishing different elements in the figures.
Results
Limestone outcrops
The limestone forms steep cliffs as a consequence of high calcite contents and cementation by calcite, compared to more friable rock units above and below (A–E; A). The principal constituents of the limestone in addition to calcite are detrital silicates (mostly quartz, albite, and subordinate muscovite) and authigenic glaucony (; B–E) that together make up 2–30 volume% of the rocks (Supplementary Figure 2). The most calcite-rich portions of the limestone have smooth massive faces (B,E). Elsewhere, variable amounts of detrital silicate sand and silt, and authigenic glaucony, contribute to differential weathering that accentuates original bedding (B,D,E). Glaucony content is generally higher towards the base of the limestone, where there is a steep upward transition from greensand into limestone (E; A), and glaucony contents in limestone vary from 2–30 volume % (Supplementary Figure 2). However, scattered glaucony grains occur throughout the limestone (C).
Figure 3. Outcrop appearances of Otekaike Limestone. A, Glaucony (dark) and calcite (pale) in greensand in transition to the base of Otekaike Limestone at the Earthquakes site (D). Inset summarises glaucony mineralogy. B, Backscatter electron image (BEI) of outcrop limestone from the Anatini site, showing the interior textures and mineralogy of the limestone dominated by calcite shell material (Cc) coated with cement which is mainly 2° calcite with traces of Mg, Al, Si, S and P. C, BEI view of limestone cement with P-rich glaucony. D, BEI view of a section through the outer margin of outcrop limestone from the Anatini site, with biofilm (right, dark) showing textural zonation. E, EDS element maps of the indicated area in e, showing relative distributions of Si, Mg Fe, and Al. Brighter patches in each map are well-defined mineral grains; paler zones are indications of dispersed elemental concentrations near to background levels (∼0.5 wt%).
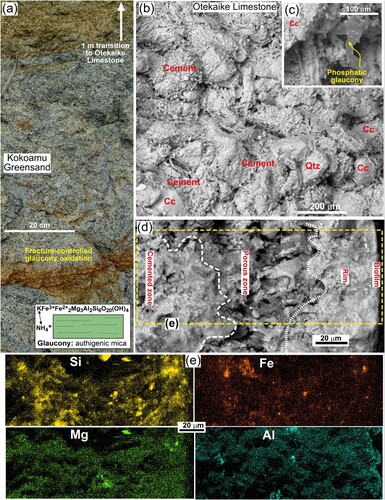
Beds with detrital silicate sand and silt are most abundant in the upper parts of the limestone (B), some of which show cross-bedding features on the 1–10 m scale. There is a general trend of increasing detrital sediment in limestone outcrops towards the northwest. The limestone at Anatini and Elephant Rocks sites has only minor sandy components, the limestone at the westernmost Lake Waitaki site has many sandy beds (D), and the intermediate Gards Road site has two distinct members with the uppermost Miller Member containing abundant detrital sand and silt (B).
Limestone outcrops with low sand and silt contents have smooth surfaces with coatings of grey biofilm. Outcrops with higher proportions of detrital silicates erode more quickly, so that the biofilm is less prominent (B). All outcrops are cut by spaced fractures (metre scale) with a range of orientations (D,E), and these fractures control water ingress and formation of some solution cavities. Uncemented pore spaces in the limestone (B–E) also permit some water passage. Irregular distributions of pore spaces, calcite cement, glaucony, and detrital silicates in the limestone facilitate development of solution cavities with a wide variety of shapes on limestone surfaces. This surficial dissolution forms irregular depressions and ridges on the 10 cm to 2 m scales, and bedding-controlled overhangs in cliffs on the metre scale (B,D,E). Many fractures and overhangs are coated with secondary calcite deposited by evaporating groundwater (E).
At sub-millimetre scales, the limestone is highly variable in mineralogy, chemistry, and textures (B–E). Fragments of calcite, mostly shell material, are variably cemented by calcite (B–D). This calcite cement typically contains minor scattered fine-grained glaucony (Mg, K, Fe, Si, Al) and dispersed phosphatic material (C–E), as well as traces of S and Cl. Most calcite fragments and matrix also have detectable Mg (>0.5 wt%), although distinguishing carbonate Mg from dispersed glaucony is difficult even at the 10 µm scale (E). The phosphate component varies widely, as well-defined apatite grains and as dispersed phosphate within glauconitic cement (C–E; Supplementary Figures 3 and 4). Scattered silicates are mostly detrital grains, and Mg-rich grains are coarse authigenic glaucony (C,E). Irregular distribution of Fe (E) includes detrital Fe-Ti oxides and redistributed in situ oxidation products. Fresh glaucony has variable K and Mg contents (Supplementary Figure 2), and weathered glaucony sits in patches of brown Fe oxyhydroxide. Fresh glaucony has minor trace metal contents such as Cu (20–50 mg/kg) and Zn (100–200 mg/kg) (Supplementary Figure 2). Glaucony may also have minor (0.01 to 0.5 wt%) nitrogen occurring as substitution of K+ sites by NH4+ (Supplementary Figure 2).
The exterior outcrop surface of the limestone shows distinct textural zonation at the 50 µm scale, as the cemented interior grades into a porous zone that lies immediately inside a re-cemented rim that hosts the biofilm (D,E; Supplementary Figure 3). The secondary calcite cement forms delicate vermiform networks in pore spaces (Supplementary Figure 3). The rim zone and adjacent porous zone has some biological material, probably lichen hyphae, penetrating through the cement (Supplementary Figure 3). Minor amorphous patches of evaporative salts (mostly NaCl, KCl, and gypsum; Supplementary Figure 5) occur on the examined broken surface, concentrated by a microfracture that controlled the specimen breakage.
Limestone disaggregation debris
Weathering of the limestone yields fragmental material, from metre-scale boulders to fine sand and silt that falls from the outcrops and accumulates in solution cavities and as talus deposits below the outcrops (A–E). Most boulders roll to the base of the talus. Finer debris accumulates on and around the outcrops in deposits from millimetres to metres thick. The debris consists of fragments of calcite-rich limestone, liberated detrital silicates (especially sand), and irregular combinations of these end-members, plus variable amounts of organic matter (A–D). The most common talus material is a mixture of cm-scale limestone chips, limestone and detrital silicate sand, in a matrix of silt (A). There have been variable degrees of oxidation of iron-bearing minerals, especially glaucony and associated authigenic pyrite (), on disaggregating outcrop surfaces and in the resultant debris (A,B). Consequently, most debris is pale tan, reflecting formation and redistribution of ferric iron oxyhydroxide (A,B). Detrital silicates are commonly coated by Fe-oxyhydroxide as well. Secondary calcite precipitates are common on exterior surfaces and fractures within clasts of debris at the cm to micron scales (A). Evaporative salts, predominantly NaCl and gypsum, also form locally from groundwater under overhangs.
Figure 4. Samples of calcicole substrates, with paste pH (blue) and generalised electrical conductivity values (EC, red, µS/cm). A, Samples of disaggregated limestone obtained in the vicinity of calcicoles (Chaerophyllum) at the Anatini site. Samples are arranged in order of increasing organic content and decreasing general particle size, from clean limestone chips (top left) to proto-soil (bottom). Whitest patches on chips are secondary calcite. B, Naturally eroded section through disaggregated limestone substrate in vicinity of samples in 4A. C, Calcicole patch (Geranium socolateum) in a broad fracture-controlled solution cavity at the Elephant Rocks site. D, Sample of organic-rich turf with sandy limestone debris obtained from below exotic plants near native calcicoles in 4C.
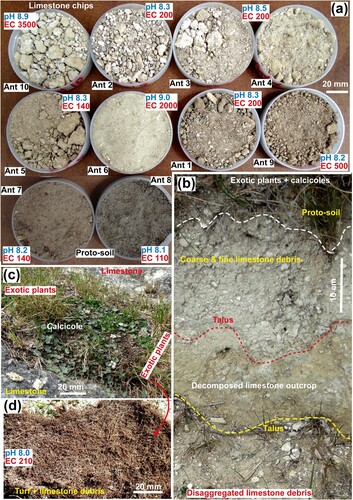
In detail, the chips within the debris retain some of the textures of outcrop limestone (A,B). The chips are crossed by zones of porous residue containing fine-grained calcite, detrital silicates, and amorphous material that includes variably degraded glaucony where some of the calcite has been dissolved (A,B; Supplementary Figure 4). The residue is highly variable chemically, but commonly contains Mg, Fe, Al, Si, K, S and minor Cl (Supplementary Figure 4). Degraded glaucony contains less K in relation to Si and Al, and the Fe is also highly variable, so the residue chemically resembles Mg-Al clay. Phosphorus occurs as discrete grains of apatite, and the porous residue also has patches with traces of P near to EDS detection limit of ∼0.5 wt% (B,C; Supplementary Figure 4).
Figure 5. SEM views of disaggregated limestone chips from the Anatini site (A). A, BEI view of chip surface showing the textural contrasts between remnants of cemented limestone and intervening zones where calcite dissolution has left porous residues. B, Close view of porous residue material, showing detrital quartz (Qtz), albite (Ab) and muscovite (Musc), and a large grain of apatite. C, EDS P element map showing scattered fine phosphatic material (bright, in white ellipses) and one bright grain of apatite. More dispersed P-enriched areas also occur, with variable slightly brighter patches near to the analytical detection limit (∼0.5 wt%). D, BEI view of fully calcified plant rootlets (possibly Chaerophyllum) and intervening needles of calcite (see also Supplementary Figures 4 and 5).
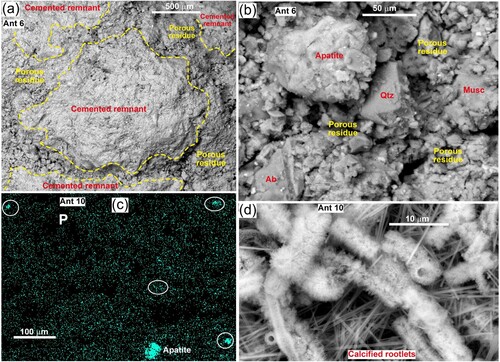
Parts of the white secondary calcite patches that are common on chip surfaces consist of variably calcified remnants of plant rootlets (A; D; Supplementary Figures 5 and 6). The imaged rootlets in D and Supplementary Figures 5 and 6 were probably derived from endemic calcicole Chaerophyllum (see Discussion below) and were obtained from below sparsely vegetated talus proto-soil near to living individuals of these species (samples Ant 1, Ant 10; A). Some organic material remained in some rootlets, but all rootlets were coated in fine secondary calcite (Supplementary Figure 6). Fully calcified roots consisted of 5–10 µm wide hollow nanocrystalline tubes with numerous micron-scale exterior needles (D). The fine scale of this material precluded detailed analysis, but spot analyses showed that they were mainly calcite with minor dispersed Mg, S and Cl. The fully calcified rootlets were fragmentary (D; Supplementary Figures 5 and 6), suggesting that they were no longer occupied by flexible organic matter, and were fully mineralised. Carbonate-relevant element maps are presented in Supplementary Figure 5.
Calcicole microhabitats on limestone outcrops
Small (10–50 cm) solution cavities on limestone outcrops are important microhabitats for calcicoles (A–D). These cavities are commonly steeply incised into the outcrops, and irregularly distributed over outcrop surfaces. The cavities accumulate limestone erosional debris as residual material, and also via water and wind transport across the outcrops. The debris deposits are typically only 1–10 cm thick (B–D). Fractures in the limestone outcrops control linear solution cavities and traps for debris as well (D). Exposed locations allow erosion of some this debris by wind and rain (B,C) and some cavities remain, or have become, empty of debris (C).
Figure 6. Calcicole microhabitats in solution cavities on limestone outcrop surfaces at the Anatini site. Grey-black surfaces represent variable thicknesses of biofilm coating limestone. A, Honeycomb weathering cavities on the vertical side of a large boulder, with Geranium socolateum calcicole. B, Flat outcrop surface with cavity that is partially filled with debris (hosting Chaerophyllum novae-zelandiae calcicoles), after wind and rain erosion has removed the upper debris layer, exposing white limestone surface. Inset shows enlarged view of calcicole microhabitat on porous limestone and debris. C, Chaerophyllum calcicoles in partially filled cavities, with an adjacent empty cavity. D, Elongate cavity is controlled by a steep fracture in outcrop and hosts Geranium and Chaerophyllum calcicoles.
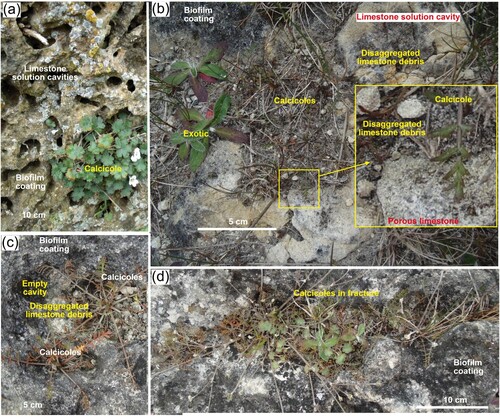
Calcicoles can become established in the thin disaggregated limestone debris deposits (B–D). Their roots extend into fractures, and may also extend into pore spaces of disaggregating solid limestone, although details of this were not examined because of the delicacy of the plants. The calcicole substrates are largely soil-free, and consist mainly of mm-scale limestone chips, coarse and fine sand, and a matrix of silt (B–D), in the microhabitats described above. Minor organic material can accumulate with the disaggregated limestone, but this encourages incursion of exotic species with consequent displacement of the endemic calcicoles. Nevertheless, some calcicoles can survive in organic-rich turf with only minor limestone debris (C,D).
Calcicoles were also observed at the top of outcrops where a thin veneer of limestone disaggregation debris accumulates in surficial iregularities (A,B). Surficial irregularities are well-developed in fractured rock and in sandier limestone (B). However, these exposed outcrop tops are also vulnerable to incursion by exotic species (A,B). Overhangs in outcrops, especially at the base of prominent cliffs, are also favourable microhabitats for calcicoles to become established in disaggregated limestone debris accumulations (C,D). Most of these sheltered sites are relatively shady, which helps to limit incursion of exotic species (C,D), and many are dry. The Island Cliff Road site (B) consists entirely of this microhabitat, albeit with minor groundwater seepages, and hosts a calcicolous grass, Simplicia sp. in its shadiest recesses (C).
Figure 7. Calcicole microhabitats on top and bottom of limestone outcrops. A, Thin debris on top of outcrop at the Earthquakes site hosts mainly exotic species with minor calcicole establishment along the debris edge. Inset shows calcicoles (Geranium) with hawkweed. B, Calcicoles (Chaerophyllum basicola) and exotic species in sandy debris on top of fractured sandy limestone at the Lake Waitaki site. C, Calcicole grass (Simplicia felix) with exotic grasses in fallen debris under an overhang at the base of an Island Cliffs limestone outcrop. D, Calcicoles (Geranium microphyllum) and exotic grass under an overhang at base of cliff at the Anatini site.
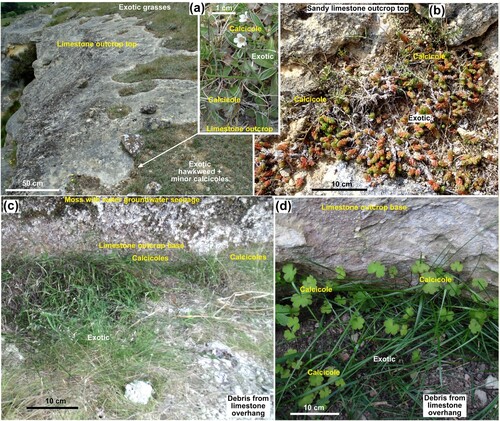
Calcicole microhabitats on talus deposits
Talus deposits of poorly sorted angular disaggregated calcite-rich limestone debris accumulate on slopes below outcrops and coat areas in between outcrops (A–D; A–E). Calcicoles occur where these talus deposits are thin (<20 cm) and typically have at least some bare soil-free substrate (A-E). Calcicoles can grow amongst coarse (10 cm scale) debris provided there is a finer matrix (A,B). However, most calcicoles occur in coarse to fine sand with larger (cm scale) chips of limestone and a matrix of silt (C–E). Talus derived from sandy and silty limestone has a higher proportion of sand, with fine-grained matrix, and fewer coarse limestone chips (A–D). This debris is readily moved by wind and rain, and is especially abundant at the bases of outcrops (A,C). Some calcicole substrates have minor components of organic matter, in a transition to proto-soil (A,D,E). The talus substrate samples in A are arranged in order of increasing organic content, and show the progression of development of proto-soil on variably vegetated talus.
Figure 8. Calcicole (Chaerophyllum) microhabitats in limestone talus at the Anatini site. A, Bare and sparsely vegetated talus, locally with proto-soil, hosting scattered calcicoles. B, Calcicoles (arrowed) in bare soil-free coarse talus. C, Calcicoles (arrowed) in proto-soil with exotic plants in talus extending from the base of outcrop. D, E, Partially bare fine talus with calcicoles (arrowed) and scattered hawkweed.
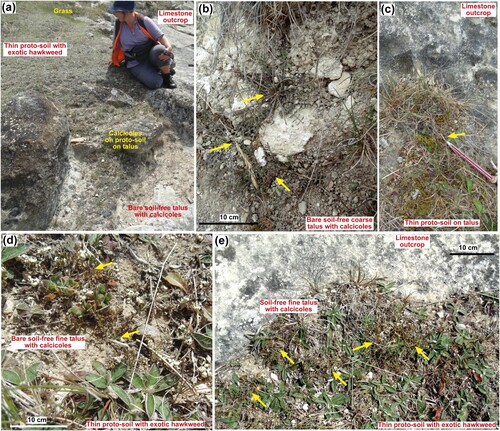
Figure 9. Calcicole microhabitats on soil-free talus associated with sandy limestone. A, Base of sandy limestone outcrop at the Gards Road, showing differential weathering of bedding, and formation of sandy talus below. B, Calcicoles (Chaerophyllum) and exotic plants in sandy talus at the Gards Road site. C, Sandy talus below outcrop at the Lake Waitaki site, with evaporative salts on surface in background, yielding localised high EC values. D, Calcicoles (Carmichaelia sp.) growing in sandy talus with limestone chips at the Lake Waitaki site.
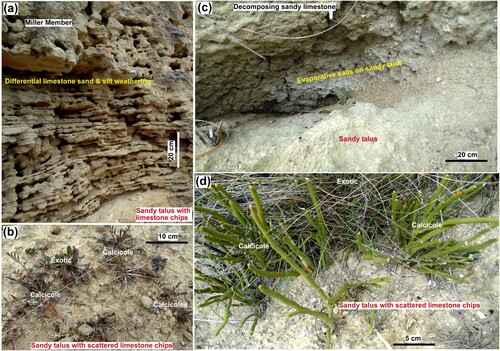
Proto-soil on talus supports some exotic plants, but these are typically not abundant and vigorous with complete ground cover (C–E). The most common accompanying exotic plant is mat-forming herb mouse-eared hawkweed which can survive in the thin dry talus but is typically <2 cm high and does not shade the calcicoles (A,D,E). The most organic-rich samples in A were extracted from beneath hawkweed patches near to substrates with a combination of hawkweed and calcicoles (A). Where talus is thicker than ∼20 cm, grasses with more advanced soil development become established, organic turf substrates dominate, and calcicoles are excluded (e.g. A; A).
Geochemical environments for calcicoles
Direct measurement of chemical parameters at the roots of the calcicoles has not been possible because of the delicacy of the plants, so proxy data are used herein to provide general characterisation of their geochemical environment. Measurements of pH and EC on slurries made with substrate samples (A,B; A–C) provide a rapid indication of two of the most important parameters. Most significantly, the calcicoles have adapted to survive in alkaline environments () as the pH range indicated for talus substrates at the Anatini site indicates (A; A). The pH in these substrates is as high as 9, and even the most organic-rich calcicole substrates have pH near 8 (A,B; A). Two nearby samples of organic-rich turf with no calcicoles, that were collected for comparison, have pH of 7.6 and 7.7 (A). The abundant calcite in all these substrates readily maintains alkaline environments (A,B). At the Lake Waitaki site, sandy talus substrates also typically have pH >8, but some organic-rich portions have pH as low as 7 (C).
Figure 10. Paste pH, EC, and experimental moisture loss data for the samples in A,B. A, The pH variations with increasing organic content, for samples organised as in A,B. B, EC values for samples as in (A). C, Plot of pH versus EC for samples in A,B, compared to North Otago groundwater (Craw et al. Citation2022) and the Lake Waitaki site disaggregated limestone substrates (Rufaut et al. Citation2023). D, Summary of results of moisture retention experiment on substrates from the Anatini site (A). Labelled curves with daily measurements are for the substrate with most moisture loss (Ant 1), the least loss (Ant 6), and the most organic-rich proto-soil (Ant 8). Data for other substrates are listed in Supplementary Table 1. E, Comparison between moisture loss rates (for substrate Ant 1 in D) and number of consecutive rain-free days (droughts of indicated lengths) in 15-year period at Waimate (Supplementary ).
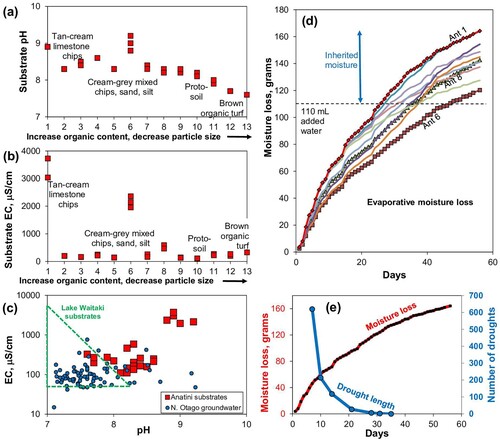
The electrical conductivities (EC) of the Anatini substrates, which reflects the amount of available dissolved ions in solution, are generally low (<300 µS/cm; B). Most of the Lake Waitaki sandy substrates had similarly low EC (C). However, some calcicole substrates have strongly elevated EC values, as high as ∼8 mS/cm at Lake Waitaki site, and 2–4 mS/cm at the Anatini site (A; B,C). These high EC values reflect the presence of evaporative salts in some substrates, and these salts are sufficiently abundant to be visible on surfaces under overhangs (C).
Geochemical environments that prevail within limestones can be examined using analyses of leachates derived by soaking talus debris in water, as has been done for Lake Waitaki sandy talus (A–F; Craw et al. Citation2022; Rufaut et al. Citation2023). More general indications of these geochemical environments can be obtained using the proxy of analyses of groundwater extracted from boreholes in the limestone-bearing rock sequence of North Otago (A–F; ORC Citation1993). All these water analyses have elevated Ca and alkalinity, and many have substantial dissolved Mg as well (A-C). The Lake Waitaki leachates have distinctly elevated K contents (D), which may reflect interaction with glaucony and detrital muscovite in the sandy talus (). These leachate K contents have an entirely different trend from that shown by marine aerosols in the North Otago groundwaters which controls the dissolved Na and Cl contents in most of these waters (D,E). Sulphate concentrations in many of the groundwaters are higher than expected from marine aerosols (F), but sulphate is relatively low in the Lake Waitaki talus leachates (F).
Figure 11. Groundwater compositions in the parts of the North Otago sedimentary sequence that are dominated by limestone (data from ORC Citation1993). Data for leachates from limestone debris at the Lake Waitaki site (Craw et al. Citation2022). Typical schist basement waters are also compared (purple boxes; Craw et al. Citation2022). All alkalinity is assumed to be bicarbonate. A–C, Dissolved Ca, Mg, and bicarbonate, in relation to carbonate dissolution lines (see text). D, E, Dissolved Na, K and Cl in relation to typical seawater (via marine aerosols). F, Sulphate versus Cl, showing excess sulphate in relation to marine aerosol contributions.
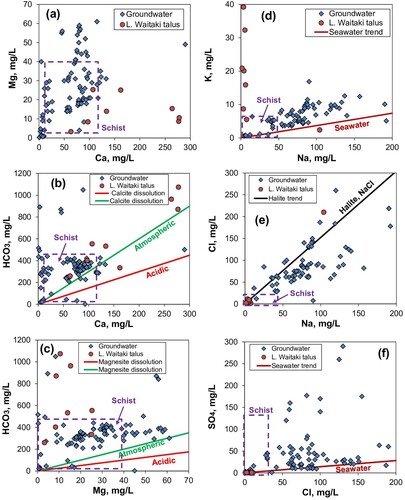
Water penetration and moisture retention
Despite the partial cementation of the limestone, there is up to 30% pore space in Otekaike Limestone outcrops (B,C; Supplementary Figure 2), and porosity is highest immediately below the biofilm-bearing rim (E). This porosity, combined with crosscutting fractures (A–E; 6d), allows water to partially saturate the limestone. Porosity is enhanced by calcite dissolution, and disaggregated limestone debris particles are locally highly porous in zones with residual minerals (A,B). This internal porosity adds to the inherent external porosity of the angular and poorly sorted detrital fragments that constitute the debris deposits (A). Consequently, limestone outcrops and their associated debris that host the calcicoles can hold and retain water that enhances the plant survival prospects during dry periods, especially in summer when evaporation can greatly exceed incoming rain (Supplementary Figure 1).
Experimental results show that these substrates can retain moisture for at least eight weeks (D). The rates of moisture loss decreased over time, with small steps in the curves reflecting hotter days (D). The pattern of moisture loss rates and changes of rates was similar for all the substrates, with some substrates apparently containing different inherited moisture levels at time of collection (D; Supplementary Table 1). The most abundant moisture loss was displayed by a sample with abundant coarse-grained limestone chips (sample Ant 1; A; D). The least moisture loss was displayed by a sample of fine limestone chips (sample Ant 6; A), which was apparently almost dry when it was collected (D). The sample with the most organic matter and consisting of abundant fine-grained limestone debris (sample Ant 8; A) had an intermediate moisture loss trajectory and apparently had a moderate moisture content when collected (D).
The high evaporation rates in summer (Supplementary Figure 1) may limit effective replenishment of substrate moisture after some of the rain events that did occur. Nevertheless, the rarity of month-long droughts compared to the longevity of moisture retention in the disaggregated limestone debris (D,E) confirms that this debris is an effective host for the dryland calcicoles.
Discussion
Limestone dissolution
Calcite in outcrops is progressively dissolved by rain water, and groundwater derived from that rain water, with assistance of dissolved atmospheric CO2 via the common reaction (Langmuir Citation1997):
(1)
(1) This reaction yields dissolved Ca and bicarbonate in 1:2 molar ratio (B). Additional calcite may be dissolved by acid generated by e.g. oxidation of pyrite in the limestone (Langmuir Citation1997; ):
(2)
(2) This reaction yields dissolved Ca and bicarbonate in 1:1 molar ratio. The very high alkalinity of the North Otago groundwaters and leachates from Lake Waitati limestone talus (B) suggest that EquationEquation (1)
(1)
(1) predominates. The very high alkalinity, combined with substantial dissolved Mg in many waters, implies that some dissolution of Mg-bearing carbonate also occurs via the Mg-carbonate equivalent of EquationEquation (1)
(1)
(1) (C). Interaction with oxidising pyrite in the sedimentary sequence is the most likely source of elevated sulphate (F; ), and low levels of S are detectable throughout the porous residue and secondary calcite in limestone chips (A,B). However, the leachates from sandy talus at the Lake Waitaki site all have low sulphate concentrations (F), so if pyrite was present in these rocks, it has now been fully oxidised.
Dissolution of exposed limestone calcite enhances incipient depressions, thereby focussing more water pooling and channelling (A,B) with a positive feedback effect. Early stages of depression formation involve localised surficial scale development and fragmentation as calcite dissolution leads to formation of a weakened porous residue (B,C). The resultant depressions trap more surface water and encourage further dissolution (B). Limestone disaggregation debris accumulates in these depressions (A,B), and the enhanced porosity of this debris further assists in water retention (D), facilitating on-going and even more localised calcite dissolution (B,C). The end result is numerous depressions, some of which are small and deep, and these are the most common microhabitats for calcicoles (A-D).
Figure 12. Summary of physical and chemical microhabitats for calcicoles. A, Otekaike Limestone outcrop at Elephant Rocks, showing sites of incipient water passage and pooling (white) across the biofilm-coated limestone. B, Otekaike Limestone outcrop at Elephant Rocks, showing three stages in evolution of surficial solution cavities. Initial decomposition of the surface crust and surface scale fragmentation (yellow, lower right) creates enhanced water pools. Further dissolution of calcite makes larger depressions with accumulated debris (red, centre and top). Localised water pools become deepened as even more calcite is dissolved and more debris accumulates (white, centre and top). C, Cartoon cross section (not to scale) of substrates hosting calcicoles, with principal chemical reactions relevant to geochemical evolution of the plant growth environments.
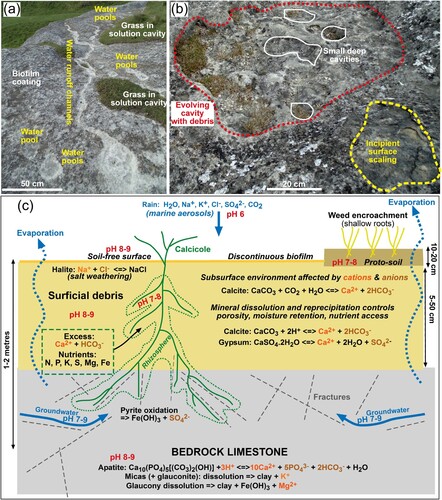
The above limestone dissolution processes are further controlled by variable contents of detrital silicate material at the scale of limestone bedding, especially the sand component (B,D; A,C). Sandy beds decompose in a different way from limestones with low sand contents, yielding overhangs and honeycomb weathering patterns that form different microhabitats for calcicoles (A; C,D). These distinctions of limestone types based on sand content are probably relevant to other New Zealand limestone substrates for calcicoles, although direct comparisons have yet to be made. The Ototara Limestone near Oamaru (B) is typically less sandy than the Otekaike Limestone, but the wetter and less evaporative coastal climatic setting for Ototara Limestone outcrops (A–D) apparently precludes establishment of dryland calcicoles.
Evaporative minerals
Biologically mediated precipitation of secondary calcite commonly occurs around plant rootlets in the rhizosphere in evaporative dryland settings (Estrada-Medina et al. Citation2013; Zamanian et al. Citation2016). These processes occur via reversal of the above carbonate dissolution reactions, and this reversal is enhanced by the periodic evaporation (Zamanian et al. Citation2016). Hence, evaporation can drive calcification of rootlets and formation of needle calcite (Verrecchia and Verrecchia Citation1994; Zamanian et al. Citation2016), both of which features have developed in the North Otago limestone debris (D; C; Supplementary Figures 5 and 6). As further debris accumulated, plants became established at the new surface and deeper debris layers were abandoned (e.g. B). Older rootlets became calcified with partial, and ultimately complete, replacement by secondary calcite (D; Supplementary Figures 5 and 6). This secondary calcification is a slow process, taking centuries (Zamanian et al. Citation2016), so it is likely that the calcified rootlets were endemic calcicoles as the hawkweed that currently accompanies the calcicoles is a relatively recent arrival (Johnstone et al. Citation1999).
Evaporation of moisture from limestone surfaces and internal porosity can raise the salinity (EC) of residual waters that originally contained marine aerosols, leading to precipitation of NaCl-rich salts in addition to the widespread secondary calcite (C; B,C; C; Supplementary Figure 5). Evaporative salts typically include gypsum, as S is detected in most precipitates, and gypsum is the dominant evaporative salt on non-limestone bare substrates near Lake Aviemore (A; Craw et al. Citation2022). Limestone porosity can be enhanced by salt weathering, which is notorious for causing decomposition of limestone building stones on human time scales (Nicholson Citation2001; Cardell et al. Citation2008; Scrivano and Gaggero Citation2020). In North Otago, these decomposition processes probably help to create extra porosity in the disaggregated limestone debris that hosts the calcicoles (A,B; A–C). Salt weathering is also likely to be active where limestone bedding features create overhangs that limit rain incursion and encourage salt accumulation (B,D,E; A,C), and some of these overhangs host calcicoles (C,D).
Establishment of calcicoles
Calcite dissolution leading to creation of disaggregated limestone fragmental material, especially fragments with porous residues (A; A–D), allows establishment of calcicoles. The calcicoles can obtain nutrients from these soil-free substrates on outcrops and in talus deposits (; Misra and Tyler Citation1999) by growing roots into the debris and possibly into the underlying outcrops (A–D; C; Supplementary Figures 3–6). Phosphorus, in particular, is a macronutrient that becomes more readily available as the limestone substrates become more porous (D; C,D; Supplementary Figure 4). Ferric iron has very low solubility at pH 8–9 (Langmuir Citation1997), but calcicoles can apparently overcome this barrier (; Lee Citation1998; Zohlen and Tyler Citation2000; Paul et al. Citation2009). In Otekaike Limestone debris, Fe is available as dispersed ferric oxyhydroxide (e.g. E) in addition to grains of glaucony (A,C; Supplementary Figure 4). Other important nutrients such as K, Mg, and S are dispersed through the variably oxidised substrate components, especially glaucony-bearing cement (e.g. C,E; C; Supplementary Figure 2, 4). Minor N may be available from glaucony and/or detrital muscovite (A; ; Craw and Rufaut Citation2017; Supplementary Figure 2), but this important macronutrient is probably the limiting nutrient factor for plant establishment. Biologically mediated availability of atmospheric nitrogen may be facilitated by the biofilm or by symbiotic bacterial activity in the root zone (rhizosphere; C), although this aspect is purely speculative at this stage of research. Important trace metals such as Zn and Cu are present in glaucony (Oze et al. Citation2019; Supplementary Figure 2), and Mo is generally enriched in marine authigenic pyrite (Bennett and Canfield Citation2020) that occurs with glaucony in Otekaike Limestone ().
Plant survival and the geochemical balance of calcite dissolution and reprecipitation in the rhizosphere are affected by periodic evaporation of scarce moisture that is retained by the porous residues of the disaggregated limestone at the millimetre scale (D; C). Our evaporation experiments show that moisture retention by porous debris occurs on time scales of weeks to months (D,E), and this is an important component of calcicole survival in the North Otago dry climate (Supplementary Figure 1). Similar, but larger scale (metres), long-term storage of water in the rhizosphere of drought-prone porous limestone substrates is described by Estrada-Medina et al. (Citation2013) and Schwinning (Citation2013).
Physical versus geochemical controls on plant establishment on bare ground
Limestone and disaggregated limestone debris are not chemically toxic for most plants, native or exotic, and the main controls on plant establishment are linked to physical access to nutrients and moisture (A–C). In particular, the endemic dryland calcicoles described in this study can apparently extend roots into the micron to millimetre scale pore spaces in the limestone substrates to obtain nutrients and access moisture for the long dry periods in which moisture is retained in these pore spaces (A–D; D; Supplementary Figures 3–6).
The physical controls on calcicole establishment described above are distinctly different from geochemical controls driven by toxicity of bare substrates elsewhere. For example, the saline sites of Central Otago have elevated EC (>1–50 mS/cm) as a result of extreme accumulations of evaporative salts (Rufaut et al. Citation2018; Craw et al. Citation2022, Citation2024; Rufaut et al. Citation2023). These saline sites are toxic to most plants because of the elevated salinity, especially NaCl that contributes to high sodicity (Craw et al. Citation2022; Rufaut et al. Citation2023). Some sites become extremely sodic and have very high alkalinity (up to pH 11) that can drive Al toxicity (Craw et al. Citation2022, Citation2024; Rufaut et al. Citation2023). Endemic halophytic plants can tolerate and even thrive in these extreme geochemical environments (Craw et al. Citation2022, Citation2024; Rufaut et al. Citation2023). Weed incursion occurs where the exotic halophyte Plantago coronopus has deposited sufficient organic debris to provide a less toxic surficial proto-soil layer (Craw et al. Citation2024). Weed incursion can also be facilitated where loess and other sediments form a protective surficial layer on top of the toxic saline substrates (Rufaut et al. Citation2018, Citation2023; Craw et al. Citation2024). Similar weed-incursion processes affect the calcicoles, which can be progressively excluded by grassy turf development (C,D; C; Supplementary Figure 7). Most encroaching weeds form a surficial mat of roots and associated organic matter, unlike the calcicoles and halophytes that extend roots into the bare soil-free substrates (Supplementary Figure 5–7).
Conclusions
Calcicoles live on soil-free disaggregated Otekaike Limestone debris in microhabitats that are strongly controlled by the geology and mineralogy of the limestone. Differential calcite dissolution during weathering of the limestone creates the microhabitats, with porosity locally enhanced by evaporative salt weathering. The calcicolous plants survive in an environmental balance between calcite dissolution and calcite re-precipitation. The plants are adapted to strongly alkaline chemical environments (pH 8–9) with elevated dissolved Ca, Mg, and bicarbonate, with variable salinity. The calcicoles obtain their nutrients directly from variably oxidised and altered minerals within the limestone, especially glaucony and associated phosphatic glaucony-bearing cement dispersed through the limestone (C). Nutrients become more readily available to these calcicoles as calcite dissolution creates porous residues that facilitate root access and help to retain moisture during up to month-long dry periods that characterise summer and autumn.
The drought tolerance and ability to extract nutrients directly from soil-free limestone debris under alkaline conditions allows these calcicoles to persist without competition from taller plants. However, accumulation of organic matter on and in the substrates can lower the pH and allow incursion of other plants, especially exotic grasses, to the detriment of the calcicolous communities. At least some calcicoles appear to be able to co-exist on bare ground and incipient proto-soil with the common exotic hawkweed (A; D,E; B), despite this weed being highly invasive in drylands (e.g. Johnstone et al. Citation1999; Day and Buckley Citation2011).
Acknowledgements
This project was initiated by personnel from Queen Elizabeth II National Trust, and we are grateful for the on-going enthusiasm and input from Aalbert Rebergen. Discussions with Clement Lagrue (DOC) and Scott Jarvie (Otago Regional Council) were helpful in developing this research project. We appreciate the ready access to the QE II covenant sites granted by the landowners, and Anatini owner Grant Neale kindly accompanied us in the field. Electron microscopy of substrate material was conducted at the Otago Micro and Nanoscale Imaging (OMNI) facility, University of Otago. Constructive comments on the manuscript by Nick Mortimer, an anonymous referee, and the associate editor substantially improved the presentation of the final version.
Disclosure statement
No potential conflict of interest was reported by the author(s).
Data availability statement
Most data directly relevant to this study are included within this paper or within cited references. Additional data are tabulated, along with additional figures mentioned in the text, in a Supplementary file available at figshare.com: doi: 10.6084/m9.figshare.25582794
.Additional information
Funding
References
- Armstrong RA. 1990. The influence of calcium and magnesium on the growth of the lichens Parmelia saxatilis and Xanthoria parietina on slate substrates. Environmental and Experimental Botany. 30:51–57. doi:10.1016/0098-8472(90)90008-R.
- Ayress MA. 1993. Ostracod biostratigraphy and paleoecology of the Kokoamu Greensand and Otekaike Limestone (late Oligocene to early Miocene), North Otago and South Canterbury, New Zealand. Alcheringa. 17:125–151.
- Bennett WW, Canfield DE. 2020. Redox-sensitive trace metals as paleoredox proxies: a review and analysis of data from modern sediments. Earth-Science Reviews. 204:103175. doi:10.1016/j.earscirev.2020.103175.
- Brautigan DJ, Rengasamey P, Chittleborough DJ. 2012. Aluminium speciation and phytotoxicity in alkaline soils. Plant and Soil. 360:187–196. doi:10.1007/s11104-012-1232-5.
- Cardell C, Benavente D, Rodríguez-Gordillo J. 2008. Weathering of limestone building material by mixed sulfate solutions. Characterization of stone microstructure, reaction products and decay forms. Materials Characterization. 59:1371–1385. doi:10.1016/j.matchar.2007.12.003.
- Craw D, Rufaut C. 2017. Geochemical and mineralogical controls on mine tailings rehabilitation and vegetation, Otago Schist, New Zealand. New Zealand Journal of Geology and Geophysics. 60:176–187. doi:10.1080/00288306.2017.1323765.
- Craw D, Rufaut C, Pillai D. 2022. Geological controls on evolution of evaporative precipitates on soil-free substrates and ecosystems, southern New Zealand. Science of the Total Environment. 849:157792. doi:10.1016/j.scitotenv.2022.157792.
- Craw D, Rufaut C, Read S, Pillai D. 2024. Hydrogeological controls on formation of Patearoa saline site in Central Otago, New Zealand and definition of geoecological salt lines. New Zealand Journal of Geology and Geophysics. doi:10.1080/00288306.2023.2246916.
- Day NJ, Buckley HL. 2011. Invasion patterns across multiple scales by Hieracium species over 25 years in tussock grasslands of New Zealand’s South Island. Austral Ecology. 36:559–570. doi:10.1111/j.1442-9993.2010.02191.x.
- Estrada-Medina H, Graham R, Allen M, Jiminez-Osornio J, Robles-Casolco S. 2013. The importance of limestone bedrock and dissolution karst features on tree root distribution in northern Yucatán, México. Plant and Soil. 362:37–50. doi:10.1007/s11104-012-1175-x.
- Forsyth PJ. 2001. Geology of the Waitaki area. Institute of Geological and Nuclear Sciences 1:250 000 Geological Map 19. 1 sheet + 64 pp.
- Gage M. 1957. The geology of Waitaki subdivision. New Zealand Geological Survey Bulletin. 55:1–135.
- Gao JW, Ranathunge KF, Arruda AJ, Cawthray GR, Clode PL, He X, Leopold M, Roessner U, Rupasinghe T, Zhong H, Lambers HL. 2020. Edaphic niche characterization of four Proteaceae reveals unique calcicole physiology linked to hyper-endemism of Grevillea thelemanniana. New Phytologist. 228:869–883. doi:10.1111/nph.16833.
- Graham IJ, Morgans HEG, Waghorn DB, Trotter JA, Whitford DJ. 2000. Strontium isotope stratigraphy of the Oligocene-Miocene Otekaike Limestone (Trig Z section) in southern New Zealand: age of the Duntroonian/Waitakian Stage boundary. New Zealand Journal of Geology and Geophysics. 43:335–347. doi:10.1080/00288306.2000.9514891.
- Hall A, Buckley HA. 1991. Ammonium in glauconite and celadonite. Mineralogical Magazine. 55:280–282. doi:10.1180/minmag.1991.055.379.17.
- Heenan PB, McGlone MS. 2013. Evolution of New Zealand alpine and open-habitat plant species during the late Cenozoic. New Zealand Journal of Ecology. 37:105–113.
- Heenan PB, Molloy BP. 2006. A new species of Oreomyrrhis (Apiaceae) from southern South Island, New Zealand, and comparison of its limestone and ultramafic habitats. New Zealand Journal of Botany. 44:99–106. doi:10.1080/0028825X.2006.9513009.
- Heenan PB, Molloy BP. 2019. Five new and nationally threatened taxa of Brachyscome, Cardamine, Convolvulus, Geranium and Ranunculus obligate to vulnerable limestone habitats, eastern South Island, New Zealand. Phytotaxa. 415:32–48. doi:10.11646/phytotaxa.415.1.2.
- Heenan PB, Rogers GM. 2019. Conserving the plants of eastern South Island limestone. Christchurch: Canterbury Botanical Society. 224 pp.
- Johnstone PD, Wilson JB, Bremner AG. 1999. Change in Hieracium populations in eastern Otago over the period 1982–1992. New Zealand Journal of Ecology. 23:31–38.
- Kitt W. 1962. Survey of limestone analyses 1917–1961. Wellington: Dominion Laboratory Report DL 2060.
- Kuster TM, Arend M, Bleuler P, Gunthardt-Goerg MS, Schulin R. 2013. Water regime and growth of young oak stands subjected to air-warming and drought on two different forest soils in a model ecosystem experiment. Plant Biology. 15:138–147. doi:10.1111/j.1438-8677.2011.00552.x.
- Lambers H, Oliviera RS. 2019. Plant physiological ecology. 3rd ed. Cham: Springer Nature. 735 pp. ISBN 978-3-030-29638-4.
- Langmuir D. 1997. Aqueous environmental geochemistry. Upper Saddle River, NJ: Prentice Hall.
- Lauchli A, Grattan SR. 2012. Soil pH extremes. In: S. Shabala, editor. Plant stress physiology. Wallingford: CAB International; p. 194–209. Chapter 9. doi:10.1079/9781845939953.0194.
- Lee JA. 1998. The calcicole – calcifuge problem revisited. Advances in Botanical Research. 29:1–30. doi:10.1016/S0065-2296(08)60306-7.
- Liao JX, Liang DY, Jiang QW, Mo L, Pu GZ, Zhang D. 2020. Growth performance and element concentrations reveal the calcicole – calcifuge behavior of three Adiantum species. BMC Plant Biology. 20:327. doi:10.1186/s12870-020-02538-6.
- Martensson L-M, Olsson PA. 2010. Soil chemistry of local vegetation gradients in sandy calcareous grasslands. Plant Ecology. 206:127–138. doi:10.1007/s11258-009-9629-9.
- Misra A, Tyler G. 1999. Influence of soil moisture on soil solution chemistry and concentrations of minerals in the calcicoles Phleum phleoides and Veronica spicata grown on a limestone soil. Annals of Botany. 81:401–410. doi:10.1006/anbo.1999.0941.
- Morgan PG. 1919. The limestone and phosphate resources of New Zealand (considered principally in relation to agriculture). Part 1, Limestone. New Zealand Geological Survey Bulletin 22.
- Mortimer N, Strong DT. 2014. New Zealand limestone purity. New Zealand Journal of Geology and Geophysics. 57:209–218. doi:10.1080/00288306.2014.901230.
- Nicholson DT. 2001. Pore properties as indicators of breakdown mechanisms in experimentally weathered limestones. Earth Surface Processes and Landforms. 26:819–838. doi:10.1002/esp.228.
- NIWA. 2023. National Institute and Water and Atmospheric Research websites: https://www.niwa.co.nz/climate; https://www.cliflo.niwa.co.nz.
- ORC. 1993. North Otago Groundwater Investigation. March 1993 prepared by IRRICON Ltd for Otago Regional Council. Dunedin. ISBN 0-908922-06-X.
- Oze C, Smaill JB, Reid CM, Palin M. 2019. Potassium and metal release related to glaucony dissolution in soils. Soil Systems. 3:70. doi:10.3390/soilsystems3040070.
- Paul A, Hauk M, Leuschner C. 2009. Iron and phosphate uptake explains the calcifuge calcicole behavior of the terricolous lichens Cladonia furcata subsp. furcata and C. rangiformis. Plant and Soil. 319:49–56. doi:10.1007/s11104-008-9848-1.
- Rogers GM, Courtney SP, Heenan PB. 2018. The calcicolous vascular flora of New Zealand. Wellington: Department of Conservation. Science for Conservation 331, 37 pp. ISBN 978–1–98–851469–7.
- Rufaut C, Pillai D, Craw D. 2023. Enhancement of alkaline saline soil-free bare substrates and specialist ecosystems, southern New Zealand. Environmental Earth Science. 82:440. doi:10.1007/s12665-023-11145-1.
- Rufaut CG, Craw D, Law S, Druzbicka J. 2018. Conservation of saline patches in Central Otago needs better recognition of physical processes to secure future habitats. New Zealand Journal of Botany. 56:115–126. doi:10.1080/0028825X.2017.1418755.
- Schwinning S. 2013. Do we need new rhizosphere models for rock-dominated landscapes? Plant and Soil. 362:25–31. doi:10.1007/s11104-012-1482-2.
- Scrivano S, Gaggero L. 2020. An experimental investigation into the salt-weathering susceptibility of building limestones. Rock Mechanics and Rock Engineering. 53:5329–5343. doi:10.1007/s00603-020-02208-x.
- Srodon J, Williams L, Szczerba M, Zaitseva T, Bojanowski MJ, Marciniak-Maliszewska B, Kuligiewicz A, Starzec K, Ciesielska Z, Paszkowski M. 2023. Mechanism of late diagenetic alteration of glauconite and implications for geochronology. Geochimica et Cosmochimica Acta. 353:157–174. doi:10.1016/j.gca.2023.05.010.
- Tanaka Y, Fordyce RE. 2014. Fossil dolphin Otekaikea marplesi (Latest Oligocene, New Zealand) expands the morphological and taxonomic diversity of Oligocene cetaceans. PLoS One. 9(9):e107972. doi:10.1371/journal.pone.0107972.
- Verrecchia EP, Verrecchia KE. 1994. Needle-fiber calcite: a critical review and a proposed classification. Journal of Sedimentary Research. 64:650–664.
- Wala M, Kolodziejek J, Mazur J, Patykowski J. 2020. Differences in iron acquisition strategies of calcicole plant species from xerothermic grasslands. Geoderma. 377:114572. doi:10.1016/j.geoderma.2020.114572.
- Waters JM, Craw D. 2007. Evolution and biogeography of New Zealand’s longjaw galaxiids (Osmeriformes: Galaxiidae): the genetic effects of glaciation and mountain building. Freshwater Biology. 53:521–534. doi:10.1111/j.1365-2427.2007.01917.x.
- Zamanian K, Pustovoytov K, Kuzyakov Y. 2016. Pedogenic carbonates: forms and formation processes. Earth-Science Reviews. 157:1–17. doi:10.1016/j.earscirev.2016.03.003.
- Zohlen A, Tyler G. 2000. Immobilization of tissue iron on calcareous soil: differences between calcicole and calcifuge plants. Oikos. 89:95–106. doi:10.1034/j.1600-0706.2000.890110.x.