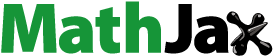
ABSTRACT
The propensity to accumulate high-value metabolites under specific conditions makes microalgae promising candidates for commercial applications. Here, the freshwater microalga Cyanophora paradoxa CCAP 981/1 was cultivated under varying conditions (control, nitrogen limitation, NaCl supplementation and blue light exposure) and the biological activity of the corresponding biomass extracts was assessed against human breast (MCF-7) and lung (A549) cancer cell lines. The stress treatments hindered the growth of C. paradoxa, the control displaying the highest specific growth rate (0.16 d−1 ± 0.01). Biomass screening by GCMS and HPLC identified diverse bioactive compounds including pigments (chlorophylls, xanthophylls, carotenes) and fatty acids (e.g., EPA). The control cultivation conditions returned the highest yields of biomass (724 mg), zeaxanthin (6.8 mg g−1), EPA (1.1 mg g−1) and phycocyanin (113.9 mg g−1), while the blue light treatment enhanced the proportions of unsaturated forms of C18 (34.3%). Extracts of dried biomass obtained by cold maceration using solvents of increasing polarity were used in the treatment of MCF-7 and A549 cancer cells. Ethyl acetate extracts from nitrogen-limited cultures reduced MCF-7 viability (~70%) after 72 h. However, the diethyl ether and methanol extracts showed no impact on MCF-7 and A549 cell viability. Furthermore, acridine orange staining revealed no significant DNA damage in these cell lines upon exposure to C. paradoxa extracts. Overall, the minimal impacts on cell viability observed here supports considering C. paradoxa as a promising source of natural bioactive compounds with applications in various biotechnological sectors.
INTRODUCTION
Microalgae constitute a polyphyletic group of eukaryotic and prokaryotic photosynthetic microorganisms that can synthesise a variety of valuable compounds (Fatemeh et al. Citation2021). They can accumulate a range of bioactive constituents such as carotenoids, proteins or fatty acids, which are of interest to the cosmetic, nutraceutical and pharmaceutical sectors (Koyande et al. Citation2019; Fatemeh et al. Citation2021). Their mass cultivation in an industrial setting is possible because of their relatively simple requirements for growth, which can be controlled in photobioreactors, enabling the optimisation of the production of specific compounds of interest (Fabris et al. Citation2020; Sarma et al. Citation2021).
Environmental fluctuations such as light, macronutrient or salinity levels have been established as important to improving cellular productivity and tailoring the biochemical profiles of microalgae (Sang et al. Citation2012; Shetty et al. Citation2019; McGee et al. Citation2020). For instance, in response to exposure to stress factors, such as light and salinity, microalgae can undergo compositional changes, including increased fatty acid and pigment content (Chainapong et al. Citation2012; Sang et al. Citation2012; Salama et al. Citation2014; Shin et al. Citation2018; McGee et al. Citation2019, Maltsev et al. Citation2021). Nitrogen limitation has also been shown to affect growth and lipid yields (Chen et al. Citation2017). However, modification of the cultivation conditions can lead to decreases in the overall biomass yield, which also needs to be considered in conjunction with the quantities of targeted compounds produced (Parkes et al. Citation2022).
The growing demand for novel substances of natural origins with potential for the treatment of human diseases, such as cancer, has led to various bioprospecting studies for the discovery of new bioactive compounds (Hamed et al. Citation2015; Talero et al. Citation2015; Saadaoui et al. Citation2020). Microalgae are regarded as a promising reservoir for such molecules (Gürlek et al. Citation2020; Eze et al. Citation2023). Indeed, several marine-derived compounds have been recognised as having various biological activities. Pigments including β-carotene, zeaxanthin, fucoxanthin or astaxanthin have been reported to exert antiproliferative activity and to induce apoptosis in a range of human cancer cell lines (e.g. breast, gastric, ovarian or liver) (Sheng et al. Citation2020; Tan et al. Citation2021; Antunes et al. Citation2022; Zhang et al. Citation2023). Phycobiliproteins, such as phycocyanin, have also shown antiproliferative effects in lung cancer cells (Hao et al. Citation2019), while polyunsaturated fatty acid rich extracts have inhibited the proliferation of various cancer cell lines, including lung (A549) and breast (MCF-7) cell lines (Vilakazi et al. Citation2021).
Ongoing research in microalgal biotechnology has acknowledged species such as Chlorella sp., Arthrospira sp., Dunaliella sp. or Scenedesmus sp. as potential sources of bioactive compounds with applications in a range of industries (Rizwan et al. Citation2018; Mutanda et al. Citation2020). However, some microalgal phyla have been understudied and warrant further scrutiny. The Glaucophyta, which includes Cyanophora paradoxa Korshikov, possesses several traits of interest, including the presence of phycobiliproteins, in addition to the carotenoids β-carotene and zeaxanthin, as well as the high-value long-chain omega-3 fatty acid EPA (Picciotto et al. Citation2021). Previous research has shown that C. paradoxa extracts do exert antiproliferative activity against some cancer cell lines (lung A549, breast MCF-7 and melanoma A-2058) (Baudelet et al. Citation2013).
The present study aimed to determine whether modifications of the cultivation conditions (sodium chloride (NaCl) supplementation, exposure to blue light and nitrogen limitation) of C. paradoxa CCAP 981/1 could modulate the profile of high-value compounds produced. In addition, the cytotoxicity of crude extracts of C. paradoxa was investigated in human lung and breast cancer cells.
MATERIAL AND METHODS
Chemicals and reagents
Chemicals including sodium chloride (NaCl), sodium sulfate (Na2SO4), potassium phosphate monobasic (KH2PO4) and potassium phosphate dibasic (K2HPO4) were procured from Merck (Darmstadt, Germany) and Fisher Scientific (Leicestershire, UK). Solvents (methanol, chloroform, acetone, ammonium acetate, diethyl ether, ethyl acetate and dimethyl sulfoxide) were purchased from Fisher Scientific. Trime-thylsulfonium hydroxide (TMSH), the internal standard nonadecanoic acid (C19:0) and the anti-cancer agent doxorubicin was acquired from Merck. The Supelco® 37 Component FAME standards Mix was procured from VWR International (Leuven, Belgium) and the pigment standards zeaxanthin, chlorophyll a, and β-carotene were sourced from DHI Group (Horsholm, Denmark) and Merck. The growth media for cell culture studies, Ham’s F-12 Nutrient Mix medium and Dulbecco’s Modified Eagle medium (DMEM) came from Fisher Scientific, and cell culture supplements, fetal bovine serum from Cytiva (Pasching, Austria), and penicillin-streptomycin from Sigma-Aldrich (Steinheim, Germany). For bioactivity assays, the 3-(4,5-dimethylthiazol-2-yl)-5-(3-carboxymethoxyphenyl)-2-(4-sulfophenyl)-2H-tetrazolium (MTS) assay was purchased from Promega UK Ltd (UK). Trypan blue solution was obtained from VWR International and acridine orange from Thermo Fisher Scientific (Kandel, Germany). All chemicals and reagents used in this study were of analytical and chromatography grades.
Microalgal Cultivation and Experimental Setup
Cyanophora paradoxa CCAP 981/1 was cultivated under control (CONT) conditions in a 10-litre glass bioreactor containing seven litres of sterile Bold Basal Medium (BBM) and vitamins addition (Bold Citation1949; McGee et al. Citation2018). The strain was also grown under three different treatments: BBM medium with blue LED light (BLU) at wavelengths of 430-465 nm; BBM medium with 18 mM of sodium chloride (NaCl) with white LED light; and BBM medium without nitrogen addition (N-) under white LED light. All the experiments were conducted with the light intensity of 80 µmol photons m−2 s −1 under a light:dark cycle of 14:10 h. Growth was monitored by measuring cell concentration using a haemocytometer. The biomass was harvested from the four bioreactors after 27 days by centrifugation (2000x g, 3 min), freeze-dried and stored at -20°C until further analysis. Specific growth rates (μ) were derived from the equation:
Where No is the cell concentration at time zero, Nt is the cell concentration at time t and ∆t is the time interval in days between Nt and No based on Levasseur et al. (Citation1993) and McGee et al. (Citation2019).
Pigment Extraction and High-Performance Liquid Chromatography (HPLC) Analysis
Pigment extraction was carried out under subdued lighting according to McGee et al. (Citation2018). Biomass (1 mg dry weight) was extracted with 500 µl ice-cold 100% v/v acetone containing glass beads using a FastPrep FP 120 shaker (Thermofisher, USA) for 40 sec at a speed of 4.0 m s−1. Extracts were then supplemented with 150 µl of deionised water (diH2O) and 850 µl of 100% acetone. Bead homogenisation was then repeated. The extracts were filtered through 0.2 μm PTFE HPLC syringe filters (Fisher Scientific), transferred into amber vials, stored at -800C and analysed within 24 h.
Quantification of pigments was conducted in a constant temperature room, using the Varian ProStar HPLC binary system coupled with a ProStar 310 UV and 335 PDA detector (Varian, USA). The extracts were injected into a 20 µl loop. Pigments were separated using a Phenomenex Onyx C18 100 × 4.6mm ID monolithic column coupled with a Phenomenex Onyx C18 guard cartridge 10 × 4.6mm ID (Phenomenex, US). The gradient used was that of McGee et al. (Citation2018) with a stepped gradient solvent programme which involved 10% B initial isocratic condition for 0.10 min, a linear gradient to 65% B from 0.10 to 2.00 min, isocratic hold at 65% B for 2 min, linear gradient to 90% B for 1 min followed by a hold at 90% B for 1 min and a final re-equilibration step to the initial conditions from 6.01 to 7.50 min. The mobile phase was composed of two solvent mixtures: A, comprised of 80:20 (v/v) methanol:0.5M ammonium acetate; and B, comprised of 70:30 (v/v) acetone:acetonitrile. Pigment identification was achieved by the comparison between retention time and peak absorption spectrum. A five-point calibration curve ranging from 0.01-50 µg ml−1 was plotted using zeaxanthin, chlorophyll a, and β-carotene standards.
Fatty Acid Extraction and GC-MS Analysis
Lipid extraction was performed according to Parkes et al. (Citation2022) with slight modifications. Freeze-dried biomass (2.5 mg) was mixed with 400 μl methanol, 200 μl chloroform and 40 μl diH2O. In addition, 5 μl of nonadecanoic acid (C19:0) at a concentration of 33.5 mM was included as an internal standard before the samples were vortexed for 30 sec. A further 200 μl of chloroform and 200 μl of diH2O were introduced followed by an additional 30 sec of vortexing. The tubes were then centrifugated at 400x g for 10 min. The upper aqueous layer was discarded while the lower layer was transferred into a new tube. Following this, 200 μl of chloroform and 200 μl of methanol were added and subsequently vortexed for 30 sec followed by centrifugation (400x g, 10 min). The supernatant was transferred into a new tube and residual moisture was removed using sodium sulfate (Na2SO4) powder. The supernatant was collected and dried under a nitrogen stream. The extracts were suspended in 250 μl of chloroform. Prior to fatty acid methyl esters (FAME) analysis by Gas Chromatography-Mass Spectrometry (GC-MS), 50 μl of sample extract was mixed with 75 μl of trime-thylsulfonium hydroxide (TMSH) in the inserts of GC-MS vials.
The analysis of FAME profiles was conducted by gas chromatography (GC) (Agilent Technologies 6890N Network GC System) coupled to a mass spectrometer (MS) (Agilent Technologies 5973 inert Mass Selective Detector) equipped with a BPX70 120 m column (0.25 mm internal diameter) (Agilent, USA). Injections (3 μl) were carried out at a split ratio of 1:1. The program was set up with an inlet temperature of 250°C and helium carrier gas at 60 psi. The oven gradient temperature was as follows: an initial hold at 50°C for 2 min followed by a 20°C/min ramp to 160°C for 8 min, a 4°C/min ramp to 220°C for 5 min and finally a 4°C/min ramp to 240°C for 12.5 min. The MS solvent delay was set to 10.5 min. Fatty acids were characterised by comparing retention times to the Supelco® 37 Component FAME standards Mix and cross-referenced to the MS instrument internal NIST08 library.
Phycobiliproteins Analysis
Extraction of water-soluble phycobiliproteins (PBP) was carried out on C. paradoxa biomass using phosphate buffer according to McGee et al. (Citation2019). Briefly, 1 ml of 0.1 M phosphate buffer (pH 6.8) was added to 1 mg of freeze-dried biomass and stored at -200C until frozen. Samples were subsequently thawed and homogenised with glass beads by bead-beating (4.0 m s−1, 20 sec). After four freeze-thawing cycles, the samples were centrifuged at 6000x g for 5 min to remove cellular debris and the PBP content was determined from the supernatant via absorbance measurements at 562, 615, 652 and 720 nm using a Jenway 6305 UV/Vis spectrophotometer.
Phycocyanin (PC), Allophycocyanin (APC) and Phycoerythrin (PE) concentrations were determined according to the Bennett and Bogorad (Citation1973) equations:
The extraction yield was calculated according to the Silveira et al. (Citation2007) equation:
where PBP is phycobiliprotein concentration (mg ml−1), V is the solvent volume (ml) and DB is the dry biomass (g).
Preparation of Microalgae Extracts for Bioactivity Assays
Crude microalgal extracts were prepared by extracting 40 mg of freeze-dried microalgae in 30 ml of solvents of varying polarity in the order: diethyl ether (Et2O), ethyl acetate (EA) and methanol (MeOH) (McGee et al. Citation2020). Samples were stored at 2–6°C for 24 h before centrifugation (2000x g for 5 min). The supernatant was recovered and passed through a 0.22 μm PTFE syringe filter. The residual biomass was then mixed with the next solvent. The supernatants of the successive extracts obtained were then placed in pre-weighted vials and evaporated to dryness. The dried extracts were resuspended in 100 μl of dimethyl sulfoxide (DMSO) and kept at -20°C until analysed. Working stocks were freshly made on the day of analyses using the culture medium of the cell lines to reach <1% DMSO concentration.
Mammalian Cell Cultivation
A549 (human lung adenocarcinoma cell line) and MCF-7 (breast carcinoma cell line) were maintained at 37°C in a humidified atmosphere (5% CO2) in Ham’s F-12 Nutrient Mix medium and Dulbecco’s Modified Eagle medium (DMEM), respectively (Panja et al. Citation2016). Both culture media were supplemented with 10% v/v fetal bovine serum (FBS) and 1% w/v penicillin-streptomycin (Pen-Strep). The cells were sub-cultured every two days, with exponential phase cells being used throughout the experiments. Cell viability was assessed by trypan blue exclusion assay as described in Cooper et al. (Citation2016).
Cytotoxicity Analysis – MTS assay
Cytotoxicity was evaluated using the 3-(4,5-dimethylthiazol-2-yl)-5-(3-carboxymethoxyphenyl)-2-(4-sulfophenyl)-2H-tetrazolium (MTS) cell proliferation assay based on Archer et al. (Citation2021). Cells (2 x 104 cells ml−1, seeded in 200 μl medium) were added to 96-well flat-bottom plates and incubated for 24 h at 37°C. Following incubation, the cells were treated with microalgal extracts at a concentration of 100 μg ml−1, containing <1% DMSO, and incubated for 72 h. Doxorubicin (5 μg ml−1) a broad-spectrum chemotherapy drug was used as a positive control for cytotoxicity (Lalitha et al. Citation2020). DMSO (<1%) and non-treated cells were included as vehicle and negative controls, respectively. At the end of the treatment period, cytotoxicity assays were performed using CellTiter 96® AQueous One Solution Cell Proliferation (MTS) assay (Promega) according to the manufacturer’s instructions. Briefly, MTS reagent was added into each well and cells were incubated at 37°C for 3 h. Absorbance was measured with a microplate reader FLUOstar OPTIMA (BMG Labtech, Offenburg, Germany) at 490 nm. Cell viability was expressed as a percentage of treated cells against the untreated controls. Values were expressed as mean ± SD of three separate experiments, each experiment was performed in triplicate and samples were normalised to the vehicle control. The percentage of viable cells was calculated as follows:
Acridine Orange Staining
Acridine orange staining was conducted to assess the genotoxic potential of the microalgal crude extracts following Picciotto et al. (Citation2021). Briefly, cells were seeded in a 96-well plate (2x104 cells ml−1) and incubated for 24 h. After incubation, cells were treated with microalgal crude extracts at a concentration of 100 μg ml−1 for 72 h. Thereafter, the medium was carefully discarded, and the cells were washed twice with PBS. The cells were then stained with acridine orange/PBS solution (100 μl) at 100 μg ml−1 for 10 min at room temperature before examination by Eclipse Ts2 fluorescence microscopy (Nikon Instruments Inc., US) using red and green filters (560 and 470 nm).
Statistical Analysis
Analysis of variance (ANOVA) was performed to identify potentially significant differences between the yields of pigments, fatty acids and phycobiliproteins in the microalgal biomass, as well as cell viability in human cells. Principal component analysis (PCA) was also applied to the data matrix to assess the relationships among the different variables: zeaxanthin content, chlorophyll a content, carotenoid content, fatty acids, phycobiliproteins and bioactivity. All statistical analyses were performed using the IBM SPSS Statistics 28 package.
RESULTS
Microalgal Growth under Various Conditions
The growth of Cyanophora paradoxa under various cultivation conditions including control (CONT), blue light (BLU), nitrogen limitation (N-) and 18 mM sodium chloride (NaCl) was monitored (). The BLU (0.03 d−1 ± 0.03) and NaCl (0.08 d−1 ± 0.03) treatments returned significantly lower specific growth rates as compared to the CONT (0.16 d−1 ± 0.01) and N- (0.14 d−1 ± 0.02) treatments (ANOVA, p < 0.001, n = 12) (Table S1). Varying amounts of dried biomass were then recovered, following centrifugation, from the cultures (CONT 724 mg, BLU 57 mg, N- 100 mg and NaCl 304 mg).
Figs 1–4. Growth (cells ml−1) and pigment production of Cyanophora paradoxa in various cultivation conditions: control (CONT), blue light (BLU), nitrogen limitation (N-) and 18 mM of NaCl.

Effect of Cultivation Conditions on Pigment and Phycobiliprotein Composition
Alterations to the culture medium of C. paradoxa influenced pigment abundance as shown in . Chlorophyll a, β-carotene and zeaxanthin were detected in all extracts (). A significant 3-fold decrease in the yield of zeaxanthin was observed following BLU treatment (2.2 mg g−1) compared to the CONT treatment (6.8 mg g−1) (ANOVA, p < 0.001, n = 12). This pattern was also observed for β-carotene, with BLU treatment having significantly less pigment (2.1 mg g−1) as compared to the CONT (7.5 mg g−1), N- (4.6 mg g−1) and NaCl (8.7 mg g−1) treatments (ANOVA, p < 0.001, n = 12). There were also significant differences in chlorophyll a yields, with BLU and N- treatments having significantly less (~7.5 mg g−1) than the CONT and NaCl treatments (~19.0 mg g−1) (ANOVA, p < 0.001, n = 12).
Table 1. Pigment and phycobiliprotein content (mg g−1) in the biomass of Cyanophora paradoxa strain CCAP 981/1 under various conditions: control (CONT), blue light (BLU), nitrogen limitation (N-) and 18 mM NaCl. All data are mean ± SD (n = 3). (ANOVA, p < 0.001, n = 12). Letters show homogenous subsets compared by line.
Both the phycocyanin and allophycocyanin contents were significantly higher in the CONT as compared to the three treatments (ANOVA, p < 0.001, n = 12) (). The phycocyanin content ranged from 113.9 mg g−1 in the CONT treatment to 19.4 mg g−1 in the BLU treatment. The allophycocyanin yields were 71.1 and 12.6 mg g−1 for the CONT and BLU treatments, respectively.
Effect of Cultivation Conditions on Fatty Acid Composition and EPA Content
Significant changes in the relative proportions of some FAMEs were observed in response to stress conditions (). There was a significant decrease in the proportions of C20:4 following the BLU treatment (7.1%) compared to the CONT (22.7%), N- (24.5%) and NaCl (22.3%) treatments (ANOVA, p < 0.001, n = 12) (Table S2). There was also a significant increase in C16:1 following the BLU treatment (11.2%) as compared to the CONT (1.3%), N- (1.4%) and NaCl (1.8%) treatments (ANOVA, p < 0.001, n = 12). In addition, the BLU treatment enhanced the accumulation of unsaturated forms of C18 (34.3%) compared to the CONT (6.5%), N- (6.6%) and NaCl (19.4%) treatments (ANOVA, p < 0.001, n = 12). As shown in , the BLU treatment also led to a significant reduction in polyunsaturated fatty acids (PUFA) proportions, while increasing those of monounsaturated fatty acids (MUFA) (ANOVA, p < 0.001, n = 12). The EPA content was negatively affected by all the treatments compared to the CONT (ANOVA, p < 0.001, n = 12) (). The highest EPA yield obtained for the CONT (1.1 mg g−1) was ~4-fold greater than that of the BLU treatment (0.3 mg g−1).
Figs 5–7. Fatty acid analysis of Cyanophora paradoxa cultivated under various conditions: control (CONT), blue light (BLU), nitrogen limitation (N-) and 18 mM NaCl.

Table 2. Fatty acids proportions (%) in the biomass of Cyanophora paradoxa strain CCAP 981/1 under various conditions: control (CONT), blue light (BLU), nitrogen limitation (N-) and 18 mM NaCl. All data are mean ± SD (n = 3). (ANOVA, p < 0.001, n = 12). Letters show homogenous subsets compared by line.
Antiproliferative Effect of Crude Extracts on Cancer Cell Lines - MTS Assay
Extracts of Et2O, EA and MeOH (100 µg ml−1) obtained from each treatment were used to monitor their effect on the cell viability of human lung (A549) and breast (MCF-7) cancer cell lines. There was no significant difference in A549 cell viability with any extract (). The MCF-7 cell line appeared more sensitive to extracts, in particular to the EA extract obtained following the N- treatment, for which a significant reduction in viability was observed (~70%) (ANOVA, p < 0.001, n = 12) as compared to the Et2O extracts (CONT and N-) ().
Figs 8, 9. Relative cell viability of human cancer cell lines exposed to diethyl ether (Et2O), ethyl acetate (EA) and methanol (MeOH) extracts derived from Cyanophora paradoxa at a concentration of 100 µg ml−1 over 72 h. Values are expressed as mean ± SD of three separate experiments, each assay was performed in triplicate. Lettering indicates homogenous subsets (ANOVA, p < 0.001, n = 12).

Genotoxicity Assay - Acridine Orange Staining
A549 and MCF-7 human cancer exposed to C. paradoxa extracts were stained with acridine orange and examined microscopically to assess the presence of genotoxic effects after 72 h. The nuclei of treated cells appeared uniformly bright green, indicating that DNA within the nucleus remained undamaged, with a cellular structure comparable to that of the untreated control cells (). Only a minor population of MCF-7 cells (exposed to NaCl derived extract) exhibited red and orange fluorescence outside the nucleus, presumably associated with lysosomes.
Principal Component Analysis (PCA)
A PCA examined patterns among the treatments and parameters measured. The PCA revealed two components, accounting for 88% of the variance (). Component 1 (CP1) was positively related to variables such as phycobiliproteins and Et2O extract bioactivity on A549 cells and negatively associated with the Et2O and EA extract bioactivities on A549 and MCF-7 cells. Component 2 (CP2) was more closely aligned with the fatty acid content. In addition, both the zeaxanthin content and MeOH extract bioactivity on A549 cells were strongly associated with the positive domain on CP2.
Fig. 11. Principal Component Analysis (PCA) projections indicating the relationships between the treatment conditions applied to C. paradoxa and the variables measured (compounds including carotenoids, fatty acids and phycobiliproteins



The four treatments applied in this study were projected in different locations of the PCA plane. In particular, the CONT and N- treatments were firmly associated to the positive axes of CP1 and CP2, respectively, while the BLU treatment was instead located along the negative domain of CP2.
DISCUSSION
Microalgae cultivation has been characterised as an effective approach to fulfilling the currently increasing demand for bioactive natural products (Sousa et al. Citation2023). The sustainability of the production of large quantities of microalgal biomass on an industrial scale depends on carefully selecting species and strains (Gatamaneni et al. Citation2018; Sousa et al. Citation2023). Glaucophytes, in particular, have shown promise due to their biochemical composition, with potential applications in various industries (Bothe & Floener Citation1978; Manirafasha et al. Citation2016). To date, most research on this group has focused on the species Cyanophora paradoxa, which is relatively easy to cultivate (Chapman Citation1966; Schenk et al. Citation1983; Kleinig et al. Citation1986; Fischer & Häder Citation1992; Pfanzagl et al. Citation1996; Price et al. Citation2017). In order to improve cell cultivation and biomass processing, it is crucial to use conditions and parameters that maximise the desired cellular components (Siddiki et al. Citation2022). This work aimed to address this challenge by modulating cultivation conditions to alter the production of bioactive components in C. paradoxa.
Cultivation of C. paradoxa under our various treatments returned varying quantities of biomass and compounds measured. While most glaucophytes contain a rigid cell wall, it is absent in C. paradoxa, making it more easily susceptible to stress and damage (Jackson et al. Citation2015, Price et al. Citation2017). Light and mineral nutrient deficiency, especially the lack of nitrogen, have previously been shown to cause cellular stress and to reduce the productivity of microalgae (Solovchenko et al. Citation2008; Maltsev et al. Citation2021). It is possible that the BLU and N- treatments used in this study caused substantial cellular stress, leading to cell lysis at the end of the cultivation, thus explaining the lower biomass yields retrieved following centrifugation. Interestingly, the biomass of C. paradoxa obtained under the CONT and NaCl treatments was associated with greater intracellular pigment content.
Light is important for photosynthesis and can impact biosynthetic pathways and the growth rate of microalgae (Kholssi et al. Citation2023). Microalgae have evolved to utilise photoreceptors to detect variations in light quality and to regulate metabolic processes accordingly (Chen et al. Citation2023). Here, a decrease in biomass yield, pigments and some fatty acids was observed in response to the BLU treatment in C. paradoxa. It has previously been reported that a blue light intensity of 100 µmol photons m−2 s −1 could lead to a reduction in biomass in the green microalgae Chlorella vulgaris Beijerinck, possibly due to the photo-inhibition of photosystem II, affecting growth or even causing cell death (Atta et al. Citation2013). Blue light has also been shown to negatively impact the growth of the green microalgae Desmondesmus communis E. H. Hegewald (Fettah et al. Citation2022). Blue light has been shown to enhance pigment yields (lutein and β-carotene) in the chlorophytes Ankistrodesmus sp. (DMGFW08) and Pediastrum sp. (DMGFW31) (Parkes et al. Citation2022). Other studies have demonstrated that blue light can increase the fatty acid content of algal cells as compared to other wavelengths or white light (Shu et al. Citation2012; Atta et al. Citation2013; Chen et al. Citation2013; Ma et al. Citation2018; Abomohra et al. Citation2019). Under the experimental conditions used here, exposure to blue light increased the proportions of C16:1 and C18:n fatty acids in C. paradoxa, highlighting that some lipid re-modelling was occurring. Comparable results have previously been reported with PUFAs such as α-linolenic acid (ALA) increasing in four chlorophyte species in response to blue light (Zhong et al. Citation2018). Blue light can modulate gene expression, enzyme activation and metabolic pathways in microalgae via blue-light photoreceptors (Atta et al. Citation2013; Jaubert et al. Citation2017) including for instance fatty acid desaturases, (Kis et al. Citation1998; Helamieh et al. Citation2022). It may be that FA-desaturases activated under the blue light treatment tested here catalysed the desaturation of the C16:1 and C18:n. Further investigation into this possibility would require gene expression analyses.
Nitrogen is considered the most important nutrient in the culture medium of microalgae in terms of its impact on growth, biosynthesis of pigments and fatty acids (Minhas et al. Citation2016). Even though nitrogen limitation has previously been shown to significantly increase fatty acid production in green microalgae (Khozin-Goldberg et al. Citation2002; Mamaeva et al. Citation2018; Maltseva et al. Citation2021), here, no significant modification of the relative proportions of fatty acids was observed following N- treatment. Additionally, increased lipid content in terms of dry weight was recorded in several Chlorella strains (Illman et al. Citation2000). The present study showed nitrogen limitation significantly reduced the yields of β-carotene and chlorophyll a, while having no detectable impact on the zeaxanthin content. A similar reduction in pigment content was reported in the green algae Desmondesmus communis E. H. Hegewald when exposed to a nitrogen-limited condition (Anand & Arumugam Citation2015). On the contrary, a significant increase in carotenoid content in Dunaliella sp. (UTEX 2538) Ben-Amotz & Avron and Chromochloris zofingiensis (Dönz) Fucíková & L.A.Lewis was observed under nitrogen starvation, highlighting species-specific responses (Salguero et al. Citation2003; Mao et al. Citation2018). Phycobiliprotein content can also respond to nitrogen limitation, as observed with decreased yields of both PC and APC in the red algae Porphyridium purpureum (Bory) K. M. Drew & R. Ross (Zhao et al. Citation2017). While it is clear that nitrogen limitation can increase lipid levels in microalgae cells, it also impacts biomass production and pigment composition (Pancha et al. Citation2014). Therefore, the optimal conditions for inducing lipid and pigment accumulation in microalgae must be carefully optimised to ensure adequate biomass productivity. As such, a two-stage approach where exposure to stressors is introduced later in the cultivation period could be beneficial (Mujtaba et al. Citation2012; Alishah Aratboni et al. Citation2019).
Changes in salinity can lead to osmotic stress in microalgae, which may influence the accumulation of valuable metabolites (Panahi et al. Citation2019). Previous research found increased carotenoid production in Botryococcus braunii (LB 572) Kützing with rising NaCl (17 mM to 85 mM), while even higher NaCl concentrations (200 mM) decreased the photosynthetic pigment content of the freshwater microalgae Chlamydomonas reinhardtii P.A.Dangeard (Rao et al. Citation2007; Fal et al. Citation2022). Other studies have also shown that salinity stress can increase both biomass and fatty acid production (Pandit et al. Citation2017) or solely fatty acid content (Salama et al. Citation2013; Qiao et al. Citation2021). Here, the NaCl stress (18 mM) increased the proportions of saturated fatty acids C18:1 and C18:2 compared to the control, with no obvious effect on biomass or carotenoid accumulation. This is in line with suggestions that freshwater species respond to salt stress by accumulating fatty acids as high-energy storage compounds (Shetty et al. Citation2019).
The results of this study illustrated that treatment of human cancer cells with C. paradoxa crude extracts did not reduce their viability. Weak growth inhibition (~70%) was observed in MCF-7 cells when treated with the EA extracts obtained from biomass processed following the N- treatment. This is contrary to previous results which indicated growth inhibition in both A549 and MCF-7 cell lines using C. paradoxa EtOH fractions obtained after flash chromatography (Baudelet et al. Citation2013). This might reflect antagonism or synergism processes in complex extracts as opposed to more purified fractions containing less compounds. In contrast to what was observed here, previous studies have also shown that treatment with methanolic and ethanolic extracts derived from green microalgae can cause cytotoxic effects in tumour cells (Suh et al. Citation2017; Reyna-Martinez et al. Citation2018; Ferdous et al. Citation2023). It is possible that the pigments and fatty acids in the C. paradoxa crude extracts (Figs S1–S6) may have had a role in reducing the cytotoxic effect observed, possibly by granting protection against oxidative damage (Wang et al. Citation2023).
A correlation in the bioactivity to MCF-7 cells with the EA crude extract obtained following N- treatment was highlighted in the PCA analysis. Natural compounds have been shown to possess anti-cancer properties, which may act through the modulation of signalling pathways involved in cell proliferation and apoptosis (Sun et al. Citation2019; Alateyah et al. Citation2022). Since gene expression can differ between cell types, there may be differences in the susceptibility of certain cells to specific compounds (Singh et al. Citation2018). For instance, it has been shown that overexpression of LRP (low-density lipoprotein receptor-related protein) in A549 cells can occur compared to MCF-7 cells, seemingly reducing drug access to its molecular targets (Gariboldi et al. Citation2003; Paramasivan et al. Citation2020). This property of A549 cells may also have contributed to the apparent increased resistance of A549 cells to our microalgal extracts.
Cyanophora paradoxa showed promise in terms of high-value metabolite content (e.g., zeaxanthin, β carotene, EPA, and phycocyanin) with industrial applications. The stress treatments resulted in reduced phycobiliprotein production, with the BLU treatment exhibiting adverse effects on biomass and pigment production. BLU treatment also increased the proportions of unsaturated fatty acids. Changes in the biomass composition of C. paradoxa did not affect A549 cancer cells while they moderately affected the bioactivities of the crude extracts against MCF-7 cells. Further extract fractionation and chemical structure elucidation would be needed to pinpoint the compound(s) responsible for the observed bioactivity here, thereby enabling its possible application for medical purposes.
Supplementary Material Helen Herbert.docx
Download MS Word (567.8 KB)ACKNOWLEDGEMENTS
Authors express their sincere gratitude and thanks to the Department of Science, ATU Sligo, especially Siobhan O’Connor and Rόisín Atcheson for their support.
DISCLOSURE STATEMENT
No potential conflicts of interest are reported by the authors(s).
Supplemental data
Supplemental data for this article can be accessed online at https://doi.org/10.1080/00318884.2024.2330341
Additional information
Funding
REFERENCES
- Abomohra A.E.F., Shang H., El-Sheekh M., Eladel H., Ebaid R., Wang S. & Wang Q. 2019. Night illumination using monochromatic light-emitting diodes for enhanced microalgal growth and biodiesel production. Bioresource Technology 288: 121514. DOI: 10.1016/j.biortech.2019.121514.
- Alateyah N., Ahmad S.M.S., Gupta I., Fouzat A., Thaher M.I., Das P., Al Moustafa A.E. & Ouhtit, A. 2022. Haematococcus pluvialis microalgae extract inhibits proliferation, invasion, and induces apoptosis in breast cancer cells. Frontiers in Nutrition 9: 882956. DOI: 10.3389/FNUT.2022.882956.
- Alishah Aratboni H., Rafiei N., Garcia-Granados R., Alemzadeh A. & Morones-Ramírez J.R. 2019. Biomass and lipid induction strategies in microalgae for biofuel production and other applications. Microbial Cell Factories 18: 1–17. DOI: 10.1186/s12934-019-1228-4.
- Anand J. & Arumugam M. 2015. Enhanced lipid accumulation and biomass yield of Scenedesmus quadricauda under nitrogen starved condition. Bioresource Technology 188: 190–194. DOI: 10.1016/j.biortech.2014.12.097.
- Antunes A., Carmo F., Pinto S., Andrade N. & Martel F. 2022. The anti-proliferative effect of β-carotene against a triple-negative breast cancer cell line is cancer cell-specific and JNK-dependent. PharmaNutrition 22: 100320. DOI: 10.1016/j.phanu.2022.100320.
- Archer L., McGee D., Parkes R., Paskuliakova A., McCoy G.R., Adamo G., Cusimano A., Bongiovanni A., Gillespie E. & Touzet N. 2021. Antioxidant bioprospecting in microalgae: characterisation of the potential of two marine heterokonts from Irish waters. Applied Biochemistry and Biotechnology 193: 981–997. DOI: 10.1007/s12010-020-03467-8.
- Atta M., Idris A., Bukhari A. & Wahidin S. 2013. Intensity of blue LED light: a potential stimulus for biomass and lipid content in fresh water microalgae Chlorella vulgaris. Bioresource Technology 148: 373–378. DOI: 10.1016/j.biortech.2013.08.162.
- Baudelet P.H., Gagez A.L., Bérard J.B., Juin C., Bridiau N., Kaas R., Thiéry V., Cadoret J.P. & Picot L. 2013. Antiproliferative activity of Cyanophora paradoxa pigments in melanoma, breast and lung cancer cells. Marine Drugs 11: 4390–4406. DOI: 10.3390/md11114390.
- Bennett A. & Bogobad L. 1973. Complementary chromatic adaptation in a filamentous blue-green alga. Journal of Cell Biology 58: 419–435.
- Bold H.C. 1949. The Morphology of Chlamydomonas chlamydogama, sp. nov. Bulletin of the Torrey Botanical Club 76: 101–108. DOI: 10.2307/2482218.
- Bothe H. & Floener L. 1978. Physiological characterization of Cyanophora paradoxa, a flagellate containing cyanelles in endosymbiosis. Zeitschrift Fur Naturforschung - Section C Journal of Biosciences 33: 981–987. DOI: 10.1515/znc-1978-11-1228.
- Chainapong T., Traichaiyaporn S. & Deming R.L. 2012. Effect of light quality on biomass and pigment production in photoautotrophic and mixotrophic cultures of Spirulina platensis. Journal of Agricultural Technology 8: 1593–1604.
- Chapman D.J. 1966. The pigments of the symbiotic algae (cyanomes) of Cyanophora paradoxa and Glaucocystis nostochinearum and two Rhodophyceae, Porphyridium aerugineum and Asterocytis ramosa. Archiv Für Mikrobiologie 55: 17–25. DOI: 10.1007/BF00409152.
- Chen B., Wan C., Mehmood M.A., Chang J.S., Bai F. & Zhao X. 2017. Manipulating environmental stresses and stress tolerance of microalgae for enhanced production of lipids and value-added products–a review. Bioresource Technology 244: 1198–1206. DOI: 10.1016/j.biortech.2017.05.170.
- Chen C.Y., Chen Y.C., Huang H.C., Huang C.C., Lee W.L. & Chang J.S. 2013. Engineering strategies for enhancing the production of eicosapentaenoic acid (EPA) from an isolated microalga Nannochloropsis oceanica CY2. Bioresource Technology 147: 160–167. DOI: 10.1016/j.biortech.2013.08.051.
- Chen S., Li X., Ma X., Qing R., Chen Y., Zhou H., Yu Y., Li J. & Tan Z. 2023. Lighting the way to sustainable development: physiological response and light control strategy in microalgae-based wastewater treatment under illumination. Science of the Total Environment 903: 166298. DOI: 10.1016/j.scitotenv.2023.166298.
- Cooper J.R., Abdullatif M.B., Burnett E.C., Kempsell K.E., Conforti F., Tolley H., Collins J.E. & Davies D.E. 2016. Long term culture of the a549 cancer cell line promotes multilamellar body formation and differentiation towards an alveolar type II Pneumocyte phenotype. PLoS ONE 11: e0164438. DOI: 10.1371/journal.pone.0164438.
- Eze C.N., Onyejiaka C.K., Ihim S.A., Ayoka T.O., Aduba C.C., Nwaiwu O. & Onyeaka H. 2023. Bioactive compounds by microalgae and potentials for the management of some human disease conditions. AIMS Microbiology 9: 55–74. DOI: 10.3934/microbiol.2023004.
- Fabris M., Abbriano R.M., Pernice M., Sutherland D.L., Commault A.S., Hall C.C., Labeeuw L., McCauley J.I., Kuzhiuparambil U., Ray P. et al. 2020. Emerging technologies in algal biotechnology: toward the establishment of a sustainable, algae-based bioeconomy. Frontiers in Plant Science 11: 279. DOI: 10.3389/fpls.2020.00279.
- Fal S., Aasfar A., Rabie R., Smouni A. & Arroussi H.E (2022). Salt induced oxidative stress alters physiological, biochemical and metabolomic responses of green microalga Chlamydomonas reinhardtii. Heliyon 8: e08811. DOI: 10.1016/j.heliyon.2022.e08811.
- Fatemeh K., Massoud S., Mohammad T. & Fatemeh N. 2021. Microalgae: therapeutic potentials and applications. Molecular Biology Reports 48: 4757–4765. DOI: 10.1007/s11033-021-06422-w.
- Ferdous U.T., Nurdin A., Ismail S. & Yusof Z.N.B. 2023. Evaluation of the antioxidant and cytotoxic activities of crude extracts from marine Chlorella sp. Biocatalysis and Agricultural Biotechnology 47: 102551. DOI: 10.1016/j.bcab.2022.102551.
- Fettah N., Derakhshandeh M., Tezcan Un.U. & Mahmoudi L. 2022. Effect of light on growth of green microalgae Scenedesmus quadricauda: influence of light intensity, light wavelength and photoperiods. International Journal of Energy and Environmental Engineering 13: 703–712. DOI: 10.1007/s40095-021-00456-3.
- Fischer M. & Häder D.P. 1992. UV effects on the pigmentation of the flagellate Cyanophora paradoxa – biochemical and spectroscopic analysis. European Journal of Protistology 28: 163–169. DOI: 10.1016/S0932-4739(11)80045-3.
- Gariboldi M.B., Ravizza R., Riganti L., Meschini S., Calcabrini A., Marra M., Arancia G., Dolfini E. & Monti E. 2003. Molecular determinants of intrinsic resistance to doxorubicin in human cancer cell lines. International Journal of Oncology 22: 1057–1064. DOI: 10.3892/ijo.22.5.1057.
- Gatamaneni B.L., Orsat V. & Lefsrud M. 2018. Factors affecting growth of various microalgal species. Environmental Engineering Science 35: 1037–1048. DOI: 10.1089/ees.2017.0521.
- Gürlek C., Yarkent Ç., Köse A., Tuğcu B., Gebeloğlu I.K., Öncel S.Ş. & Elibol M. 2020. Screening of antioxidant and cytotoxic activities of several microalgal extracts with pharmaceutical potential. Health and Technology 10: 111–117. DOI: 10.1007/s12553-019-00388-3.
- Hamed I., Özogul F., Özogul Y. & Regenstein J.M. 2015. Marine bioactive compounds and their health benefits: a review. Comprehensive Reviews in Food Science and Food Safety 14: 446–465. DOI: 10.1111/1541-4337.12136.
- Hao S., Li S., Wang J., Yan Y., Ai X., Zhang J., Ren Y., Wu T., Liu L. & Wang C. 2019. Phycocyanin exerts anti-proliferative effects through down-regulating TIRAP/NF-κB activity in human non-small cell lung cancer cells. Cells 8: 588. DOI: 10.3390/cells8060588.
- Helamieh M., Reich M., Bory S., Rohne P., Riebesell U., Kerner M. & Kümmerer K. 2022. Blue-green light is required for a maximized fatty acid unsaturation and pigment concentration in the microalga Acutodesmus obliquus. Lipids 57: 221–232. DOI: 10.1002/lipd.12343.
- Illman A.M., Scragg A.H. & Shales S.W. 2000. Increase in Chlorella strains calorific values when grown in low nitrogen medium. Enzyme and Microbial Technology 27: 631–635. DOI: 10.1016/S0141-0229(00)00266-0.
- Jackson C., Clayden S. & Reyes-Prieto A. 2015. The Glaucophyta: the blue-green plants in a nutshell. Acta Societatis Botanicorum Poloniae 84: 149–165. DOI: 10.5586/asbp.2015.020.
- Jaubert M., Bouly J.P., d’Alcalà M.R. & Falciatore A. 2017. Light sensing and responses in marine microalgae. Current Opinion in Plant Biology 37: 70–77. DOI: 10.1016/j.pbi.2017.03.005.
- Kholssi R., Lougraimzi H. & Moreno-Garrido I. 2023. Effects of global environmental change on microalgal photosynthesis, growth and their distribution. Marine Environmental Research 184: 105877. DOI: 10.1016/j.marenvres.2023.105877.
- Khozin-Goldberg I., Bigogno C., Shrestha P. & Cohen Z. 2002. Nitrogen starvation induces the accumulation of arachidonic acid in the freshwater green alga Parietochloris Incisa (Trebuxiophyceae). Journal of Phycology 38: 991–994. DOI: 10.1046/j.1529-8817.2002.01160.x.
- Kis M.M., Zsiros O., Farkas T., Wada H., Nagy F. & Gombos Z.Z. 1998. Light-induced expression of fatty acid desaturase genes. Proceeding of the National Academy of Sciences 95: 4209–4214. DOI: 10.1073/pnas.95.8.4209.
- Kleinig H., Beyer P., Schubert C., Liedvogel B. & Lütke-Brinkhaus F. 1986. Cyanophora paradoxa: fatty acids and fatty acid synthesis in vitro. Zeitschrift Fur Naturforschung C 41: 169–171. DOI: 10.1515/znc-1986-1-225.
- Koyande A.K., Chew K.W., Rambabu K., Tao Y., Chu D.T. & Show P.L. 2019. Microalgae: a potential alternative to health supplementation for humans. Food Science and Human Wellness 8: 16–24. DOI: 10.1016/j.fshw.2019.03.001.
- Lalitha L.J., Sales T.J., Clarance P.P., Agastian P., Kim Y.O., Mahmoud A.H., Mohamed S.E., Tack J.C., Na S.W. & Kim H.J. 2020. In-vitro phytopharmacological and anticancer activity of Loranthus Longiflorus Desv. Var. Falcatuskurz against the human lung cancer cells. Journal of King Saud University - Science 32: 1246–1253. DOI: 10.1016/j.jksus.2019.11.022.
- Levasseur M., Thompson P.A. & Harrison P.J. 1993. Physiological acclimation of marine phytoplankton to different nitrogen sources. Journal of Phycology 29: 587–595. DOI: 10.1111/j.0022-3646.1993.00587.x.
- Ma R., Thomas-Hall S.R., Chua E.T., Eltanahy E., Netzel M.E., Netzel G., Lu Y. & Schenk P.M. 2018. LED power efficiency of biomass, fatty acid, and carotenoid production in Nannochloropsis microalgae. Bioresource technology 252: 118–126. DOI: 10.1016/j.biortech.2017.12.096.
- Maltsev Y., Maltseva I., Maltseva S., Kociolek J.P. & Kulikovskiy M. 2021. A new species of freshwater algae Nephrochlamys yushanlensis sp. nov. (Selenastraceae, Sphaeropleales) and its lipid accumulation during nitrogen and phosphorus starvation. Journal of Phycology 57: 606–618. DOI: 10.1111/jpy.13116.
- Maltsev Y., Maltseva K., Kulikovskiy M. & Maltseva S. 2021. Influence of light conditions on microalgae growth and content of lipids, carotenoids, and fatty acid composition. Biology 10: 1060. DOI: 10.3390/biology10101060.
- Mamaeva A., Namsaraev Z., Maltsev Y., Gusev E., Kulikovskiy M., Petrushkina M., Filimonova A., Sorokin B., Zotko N., Vinokurov V. et al. 2018. Simultaneous increase in cellular content and volumetric concentration of lipids in Bracteacoccus bullatus cultivated at reduced nitrogen and phosphorus concentrations. Journal of Applied Phycology 30: 2237–2246. DOI: 10.1007/s10811-018-1471-9.
- Manirafasha E., Ndikubwimana T., Zeng X., Lu Y. & Jing K. 2016. Phycobiliprotein: potential microalgae derived pharmaceutical and biological reagent. Biochemical Engineering Journal 109: 282–296. DOI: 10.1016/j.bej.2016.01.025.
- Mao X., Wu T., Sun D., Zhang Z. & Chen F. 2018. Differential responses of the green microalga Chlorella zofingiensis to the starvation of various nutrients for oil and astaxanthin production. Bioresource Technology 249: 791–798. DOI: 10.1016/j.biortech.2017.10.090.
- McGee D., Archer L., Fleming G.T., Gillespie E. & Touzet N. 2020. The effect of nutrient and phytohormone supplementation on the growth, pigment yields and biochemical composition of newly isolated microalgae. Process Biochemistry 92: 61–68. DOI: 10.1016/j.procbio.2020.03.001.
- McGee D., Archer L., Paskuliakova A., Mc Coy G.R., Fleming G.T., Gillespie E. & Touzet N. 2018. Rapid chemotaxonomic profiling for the identification of high-value carotenoids in microalgae. Journal of Applied Phycology 30: 385–399. DOI: 10.1007/s10811-017-1247-7.
- McGee D., Archer L., Smyth T.J., Fleming G. T. & Touzet N. 2020. Bioprospecting and LED-based spectral enhancement of antimicrobial activity of microalgae isolated from the West of Ireland. Algal Research 45: 101704. DOI: 10.1016/j.algal.2019.101704.
- McGee D., Archer L., Fleming G.T., Gillespie E. & Touzet N. 2019. Influence of spectral intensity and quality of LED lighting on photoacclimation, carbon allocation and high-value pigments in microalgae. Photosynthesis Research 143: 67–80. DOI: 10.1007/s11120-019-00686-x.
- Minhas A.K., Hodgson P., Barrow C.J. & Adholeya A. 2016. A review on the assessment of stress conditions for simultaneous production of microalgal lipids and carotenoids. Frontiers in Microbiology 7: 1–19. DOI: 10.3389/fmicb.2016.00546.
- Mujtaba G., Choi W., Lee C.G. & Lee K. 2012. Lipid production by Chlorella vulgaris after a shift from nutrient-rich to nitrogen starvation conditions. Bioresource Technology 123: 279–283. DOI: 10.1016/j.biortech.2012.07.057.
- Mutanda T., Naidoo D., Bwapwa J.K. & Anandraj A. 2020. Biotechnological applications of microalgal oleaginous compounds: current trends on microalgal bioprocessing of products. Frontiers in Energy Research 8: 598803. DOI: 10.3389/fenrg.2020.598803.
- Panahi Y., Khosroshahi A.Y., Sahebkar A. & Heidari, H.R. 2019. Impact of cultivation condition and media content on Chlorella vulgaris composition. Tabriz University of Medical Sciences 9: 182–194. DOI: 10.15171/apb.2019.022.
- Pancha I., Chokshi K., George B., Ghosh T., Paliwal C., Maurya R. & Mishra S. 2014. Nitrogen stress triggered biochemical and morphological changes in the microalgae Scenedesmus sp. CCNM 1077. Bioresource technology 156: 146–154. DOI: 10.1016/j.biortech.2014.01.025.
- Pandit P.R., Fulekar M.H. & Karuna M.S.L. 2017. Effect of salinity stress on growth, lipid productivity, fatty acid composition, and biodiesel properties in Acutodesmus obliquus and Chlorella vulgaris. Environmental Science and Pollution Research 24: 13437–13451. DOI: 10.1007/s11356-017-8875-y.
- Panja S., Ghate N.B. and Mandal N. 2016. A microalga, Euglena tuba induces apoptosis and suppresses metastasis in human lung and breast carcinoma cells through ROS-mediated regulation of MAPKs. Cancer Cell International 16: 1–3: DOI: 10.1186/s12935-016-0330-5.
- Paramasivan P., Kumar J.D., Baskaran R., Weng C.F. & Padma V.V. 2020. Reversal of doxorubicin resistance in lung cancer cells by neferine is explained by nuclear factor erythroid-derived 2-like 2 mediated lung resistance protein down regulation. Cancer Drug Resistance 3: 647. DOI: 10.20517/cdr.2019.115.
- Parkes R., Barone M.E., Aranyos A., Fierli D., Koehler H., Gillespie E. & Touzet N. 2022. Species-specific responses in pigments and fatty acids of five freshwater Chlorophytes exposed to varying cultivation conditions. Process Biochemistry 112: 35–44. DOI: 10.1016/j.procbio.2021.11.018.
- Pfanzagl B., Zenker A., Pittenauer E., Allmaier G., Martinez-Torrecuadrada J., Schmid E.R., De Pedro M.A. & Löffelhardt W. 1996. Primary structure of cyanelle peptidoglycan of Cyanophora paradoxa: a prokaryotic cell wall as part of an organelle envelope. Journal of Bacteriology 178: 332–339. DOI: 10.1128/jb.178.2.332-339.1996.
- Picciotto S., Barone M.E., Fierli D., Aranyos A., Adamo G., Božič D., Romancino D.P., Stanly C., Parkes R., Morsbach S. et al. 2021. Isolation of extracellular vesicles from microalgae: towards the production of sustainable and natural nanocarriers of bioactive compounds. Biomaterials Science 9: 2917–2930. DOI: 10.1039/D0BM01696A.
- Price D.C., Steiner J.M., Yoon H.S., Bhattacharya D. & Löffelhardt W. 2017. Glaucophyta. In: Handbook of the protists (Ed. by J.M. Archibald, A.G.B. Simpson, & C.H. Slamovits), pp 23–87. Springer, Cham, Switzerland.
- Qiao T., Zhao Y., Zhong D.B., & Yu X. 2021. Hydrogen peroxide and salinity stress act synergistically to enhance lipids production in microalga by regulating reactive oxygen species and calcium. Algal Research 53: 102017. DOI: 10.1016/j.algal.2020.102017.
- Rao A.R., Dayananda C., Sarada R., Shamala T.R. & Ravishankar G.A. 2007. Effect of salinity on growth of green alga Botryococcus braunii and its constituents. Bioresource Technology 98: 560–564. DOI: 10.1016/j.biortech.2006.02.007.
- Reyna-Martinez R., Gomez-Flores R., López-Chuken U., Quintanilla-Licea R., Caballero-Hernandez D., Rodríguez-Padilla C., Beltrán-Rocha J.C. & Tamez-Guerra P. 2018. Antitumor activity of Chlorella sorokiniana and Scenedesmus sp. microalgae native of Nuevo León State, México. PeerJ 6: e4358. DOI: 10.7717/peerj.4358.
- Rizwan M., Mujtaba G., Memon S.A., Lee K. & Rashid N. 2018. Exploring the potential of microalgae for new biotechnology applications and beyond: a review. Renewable and Sustainable Energy Reviews 92: 394–404. DOI: 10.1016/j.rser.2018.04.034.
- Saadaoui I., Rasheed R., Abdulrahman N., Bounnit T., Cherif M., Al Jabri H. & Mraiche F. 2020. Algae-derived bioactive compounds with anti-lung cancer potential. Marine Drugs 18: 197. DOI: 10.3390/md18040197.
- Salama E.S., Kim H.C., Abou-Shanab R.A., Ji M.K., Oh Y.K., Kim S.H. & Jeon B.H. 2013. Biomass, lipid content, and fatty acid composition of freshwater Chlamydomonas mexicana and Scenedesmus obliquus grown under salt stress. Bioprocess and Biosystems Engineering 36: 827–833. DOI: 10.1007/s00449-013-0919-1.
- Salama E.S., Abou-Shanab R.A., Kim J.R., Lee S., Kim S.H., Oh S.E., Kim H.C., Roh H.S. & Jeon B.H. 2014. The effects of salinity on the growth and biochemical properties of Chlamydomonas mexicana GU732420 cultivated in municipal wastewater. Environmental Technology 35: 1491–1498. DOI: 10.1080/09593330.2013.871350.
- Salguero A., De La Morena B., Vigara J., Vega J.M., Vilchez C. & León R. 2003. Carotenoids as protective response against oxidative damage in Dunaliella bardawil. Biomolecular Engineering 20: 249–253. DOI: 10.1016/S1389-0344(03)00065-0.
- Sang M., Wang M., Liu J., Zhang C. & Li A. 2012. Effects of temperature, salinity, light intensity, and pH on the eicosapentaenoic acid production of Pinguiococcus pyrenoidosus. Journal of Ocean University of China 11: 181–186. DOI: 10.1007/s11802-012-1868-z.
- Sarma S., Sharma S., Rudakiya D., Upadhyay J., Rathod V., Patel A. & Narra M. 2021. Valorization of microalgae biomass into bioproducts promoting circular bioeconomy: a holistic approach of bioremediation and biorefinery. 3 Biotech 11: 1–29. DOI: 10.1007/s13205-021-02911-8.
- Schenk H.E., Hanf J. & Neu-Müller M. 1983. The phycobihproteids in Cyanophora paradoxa as accessoric pigments and nitrogen storage proteins. Zeitschrift Fur Naturforschung - Section C Journal of Biosciences 38: 972–977. DOI: 10.1515/znc-1983-11-1216.
- Sheng Y.N., Luo Y.H., Liu S.B., Xu W.T., Zhang Y., Zhang T., Xue H., Zuo W.B., Li Y.N., Wang C.Y. et al. 2020. Zeaxanthin induces apoptosis via ROS-regulated MAPK and AKT signaling pathway in human gastric cancer cells. OncoTargets and therapy. DOI: 10.2147/OTT.S272514.
- Shetty P., Gitau M.M. & Maróti G. 2019. Salinity stress responses and adaptation mechanisms in eukaryotic green microalgae. Cells 8: 1–16. DOI: 10.3390/cells8121657.
- Shin Y.S., Choi H.I., Choi J.W., Lee J.S., Sung Y.J. & Sim, S.J. 2018. Multilateral approach on enhancing economic viability of lipid production from microalgae: a review. Bioresource Technology 258: 335–344. DOI: 10.1016/j.biortech.2018.03.002.
- Shu C.H., Tsai C.C., Liao W.H., Chen K.Y. & Huang H.C. 2012. Effects of light quality on the accumulation of oil in a mixed culture of Chlorella sp. and Saccharomyces cerevisiae. Journal of Chemical Technology and Biotechnology 85: 601–607. DOI: 10.1002/jctb.2750.
- Siddiki S.Y.A., Mofijur M., Kumar P.S., Ahmed S.F., Inayat A., Kusumo F., Badruddin I.A., Khan T.Y., Nghiem L.D., Ong H.C. et al. 2022. Microalgae biomass as a sustainable source for biofuel, biochemical and biobased value-added products: an integrated biorefinery concept. Fuel 307: 121782. DOI: 10.1016/j.fuel.2021.121782.
- Silveira S.T., Burkert J.F.M., Costa J.A.V., Burkert C.A.V. & Kalil S.J. 2007. Optimization of phycocyanin extraction from Spirulina platensis using factorial design. Bioresource Technology 98: 1629. DOI: 10.1016/j.biortech.2006.05.050.
- Singh K.P., Miaskowski C., Dhruva A.A., Flowers E. & Kober K.M. 2018. Mechanisms and measurement of changes in gene expression. Biological Research for Nursing 20: 369–382. DOI: 10.1177/1099800418772161.
- Solovchenko A.E., Khozin-Goldberg I., Didi-Cohen S., Cohen Z. & Merzlyak M.N. 2008. Effects of light and nitrogen starvation on the content and composition of carotenoids of the green microalga Parietochloris incisa. Russian Journal of Plant Physiology 55: 455–462. DOI: 10.1134/S1021443708040043.
- Sousa V., Pereira R.N., Vicente A.A., Dias O. & Geada P. 2023. Microalgae biomass as an alternative source of biocompounds: new insights and future perspectives of extraction methodologies. Food Research International 113282. DOI: 10.1016/j.foodres.2023.113282.
- Suh S.S., Kim S.M., Kim J.E., Hong J.M., Lee S.G., Youn U.J., Han S.J., Kim I.C. & Kim S. 2017. Anticancer activities of ethanol extract from the Antarctic freshwater microalga, Botryidiopsidaceae sp. BMC Complementary and Alternative Medicine 17: 1–9. DOI: 10.1186/s12906-017-1991-x.
- Sun L.R., Zhou W., Zhang H.M., Guo Q.S., Yang W., Li B.J., Sun Z.H., Gao S.H. & Cui R.J. 2019. Modulation of multiple signaling pathways of the plant-derived natural products in cancer. Frontiers in Oncology 9: 1153. DOI: 10.3389/fonc.2019.01153.
- Talero E., García-Mauriño S., Ávila-Román J., Rodríguez-Luna A., Alcaide A. & Motilva V. 2015. Bioactive compounds isolated from microalgae in chronic inflammation and cancer. Marine Drugs 13: 6152–6209. DOI: 10.3390/md13106152.
- Tan Y., Ye Z., Wang M., Manzoor M.F., Aadil R.M., Tan X. and Liu Z. 2021. Comparison of different methods for extracting the astaxanthin from Haematococcus pluvialis: chemical composition and biological activity. Molecules 26: 3569. DOI: 10.3390/molecules26123569.
- Vilakazi H., Olasehinde T.A. & Olaniran A.O. 2021. Chemical characterization, antiproliferative and antioxidant activities of polyunsaturated fatty acid-rich extracts from Chlorella sp. s14. Molecules 26: 4109. DOI: 10.3390/molecules26144109.
- Wang N., Pei H., Xiang W., Li T., Lin S., Wu J., Chen Z., Wu H., Li C. & Wu H. 2023. Rapid screening of microalgae as potential sources of natural antioxidants. Foods 12: 2652. DOI: 10.3390/foods12142652.
- Zhang Z., Wang Y. & Li J. 2023. Fucoxanthin suppresses the malignant progression of ovarian cancer by inactivating STAT3/c-Myc signaling. American journal of translational research 15: 2528–2540.
- Zhao L.S., Li K., Wang Q.M., Song X.Y., Su H.N., Xie B.B, Zhang X.Y., Huang F., Chen X.L., Zhou B.C. et al. 2017. Nitrogen starvation impacts the performance of Porphyridium cruentum as revealed by chlorophyll a fluorescence. Scientific Reports 7: 8542. DOI: 10.1038/s41598-017-08428-6.
- Zhong Y., Jin P. & Cheng J.J. 2018. A comprehensive comparable study of the physiological properties of four microalgal species under different light wavelength conditions. Planta 248: 489–498. DOI: 10.1007/s00425-018-2899-5.