Abstract
Purpose/Aim
Bronchopulmonary dysplasia (BPD) is associated with poor survival in preterm infants. Intrauterine infection can aggravate the degree of obstruction of alveolar development in premature infants; however, the pathogenic mechanism remains unclear. In this study, we sought to determine whether pyroptosis could be inhibited by downregulating mammalian target of rapamycin (mTOR) activation and inducing autophagy in BPD-affected lung tissue.
Materials and Methods
We established a neonatal rat model of BPD induced by intrauterine infection via intraperitoneally injecting pregnant rats with lipopolysaccharide (LPS). Subsequently, mTOR levels and pyroptosis were evaluated using immunohistochemistry, immunofluorescence, TUNEL staining, and western blotting. The Shapiro–Wilk test was employed to assess the normality of the experimental data. Unpaired t-tests were used to compare the means between two groups, and comparisons between multiple groups were performed using analysis of variance.
Results
Pyroptosis of lung epithelial cells increased in BPD lung tissues. After administering an mTOR phosphorylation inhibitor (rapamycin) to neonatal rats with BPD, the level of autophagy increased, while the expression of autophagy cargo adaptors, LC3 and p62, did not differ. Following rapamycin treatment, NLRP3, Pro-caspase-1, caspase-1, pro-IL-1β, IL-1β, IL-18/Pro-IL-18, N-GSDMD/GSDMD, Pro-caspase-11, and caspase-11 were negatively regulated in BPD lung tissues. The opposite results were observed after treatment with the autophagy inhibitor MHY1485, showing an increase in pyroptosis and a significant decrease in the number of alveoli in BPD.
Conclusions
Rapamycin reduces pyroptosis in neonatal rats with LPS-induced BPD by inhibiting mTOR phosphorylation and inducing autophagy; hence, it may represent a potential therapeutic for treating BPD.
Background
Bronchopulmonary dysplasia (BPD) is the most common chronic lung disease in premature infants.Citation1 Although advances in medical technology have improved the survival rates of preterm infants younger than 29 wk gestation (up to 45%), the incidence of BPD remains unchanged.Citation2–4 BPD is a systemic disease that increases the incidence of infant mortality, short- and long-term respiratory diseases, and neurodevelopmental disorders, while also having lifelong effects on adult health and quality of life.Citation5,Citation6 Various factors increase the risk of developing BPD, including hyperoxia, mechanical ventilation, prolonged patency of the ductus arteriosus, sepsis, inflammation, and other postpartum factors. Adverse prenatal factors, such as chorioamnionitis, preeclampsia, preexisting hypertensive disorders, gestational diabetes mellitus, and maternal obesity, are also risk factors for BPD.Citation10,Citation11 Among them, infection and inflammation can become pathogenic factors for BPD before and after delivery. That is, intrauterine infection can directly affect the normal development of alveoli through inflammation and promote preterm birth via chorioamnionitis. Meanwhile, effective methods to prevent or control intrauterine infection are relatively limited,Citation10–12 resulting in high associated costs.Citation7–9 Moreover, the precise mechanism by which intrauterine infection influences the pathogenic process of BPD remains unclear and warrants further investigation.
One potential pathway with an important role in the pathological process of intrauterine infection-induced BPD is pyroptosis—a pro-inflammatory form of cell death—which can cause tissue damage.Citation13,Citation14 Indeed, pyroptosis occurs in hyperoxia-induced BPD and LPS-induced acute lung injury models, while its inhibition can alleviate lung injury.Citation16–20 Pyroptosis initiation requires at least one pro-inflammatory caspase, including caspase-1 and caspase-11 in mice (caspase-4 and caspase-5 in humans) as well as the participation of various other key factors, including NOD-like receptor pyrin domain containing 3 (NLRP3), apoptosis-associated speck-like protein containing a CARD (ASC), gasdermin D (GSDMD), interleukin (IL)-1β, and IL-18. Moreover, the non-classical caspase-11 inflammasome is formed during lipopolysaccharide (LPS) cytoplasmic exposure.Citation13–15 Within the lung tissues of neonatal mice exposed to hyperoxia, caspase-1 activation, IL-1β expression, and inflammation levels are increased, whereas alveolarization is reduced. However, blocking IL-1β with IL-1 receptor antagonists or the NLRP3 inflammasome with glyburide treatment decreases inflammation and increases alveolarisation.Citation17 Autophagy can also regulate NLRP3 inflammasomeCitation22,Citation23 and caspase-11 inflammasome activation.Citation24,Citation25
Autophagy is an intracellular process critical for transporting cytoplasmic components and clearing pathogens.Citation21 Promotion of autophagy can inhibit NLRP3 inflammasome activity, reducing acute lung injury in mice caused by sepsis.Citation16 The protein kinase B (Akt)/mammalian target of rapamycin (mTOR) signaling pathway is a key regulator of autophagy. In fact, within a mouse model of acute lung injury induced by exogenous mitochondrial damage-associated molecular patterns, administration of an autophagy agonist (i.e., rapamycin) inhibited the NLRP3 inflammasome, alleviating associated inflammation and damage.Citation18 Rapamycin also inhibits IL-1β secretion. Moreover, the anti-inflammatory factor IL-10 negatively regulates inflammasome activation by decreasing mTOR levels in mouse models of colitis and patients with inflammatory bowel disease. IL-10 also inhibits caspase-1-dependent inflammasome activation. However, the relationship between mTOR, autophagy, and pyroptosis in intrauterine infection-induced BPD remains unknown.Citation26,Citation27
The main objective of the current study is to investigate the role and significance of pyroptosis and mTOR-related autophagy in intrauterine infection-induced BPD. Specifically, we evaluate whether rapamycin administration to a BPD rat model increases mTOR-related autophagy and inhibits pyroptosis, ultimately promoting alveolar development and maturation.
Methods
Animals and BPD model generation
All animal experiments in this study were performed per the ARRIVE guidelines and carried out according to the UK Animals (Scientific Procedures) Act of 1986 and National Research Council’s Guide for the Care and Use of Laboratory Animals. The Institution’s Animal Ethics Committee approved this study (Reference number: CHENGYIDONGLUN2021007).
Female SD rats weighing 300–350 g and male SD rats weighing 350–400 g, aged 3–4 months, were purchased from Chengdu Dossy Laboratory Animal Co., Ltd. (Sichuan, China). According to previous studies, the sex of neonatal rats has little effect on the experimental outcomes; therefore, we did not distinguish between the sexes. All rats were acclimated to standard cages for 1 week before initiating the study procol and were provided ad libitum access to feed and water. Female and male rats were randomly assigned to cages at a 2:1 ratio. The conditions in the animal facility were maintained at a temperature of 22 ± 2 °C, relative humidity of 50–60%, and 12 h/12 h light/dark cycle. A positive vaginal smear was recorded as embryonic time point 0 (i.e., E0) of pregnancy. The necessary precautions were taken throughout the study to minimize pain and stress associated with the experimental treatments.
Finally, 22 pregnant female rats were included, of which 14 were assigned to the BPD model group and 8 to the sham operation group. For the pregnant rats in the intrauterine infection group, LPS (L2880-10MG; Sigma-Aldrich, St. Louis, MO, USA; dissolved in sterile 0.9% sodium chloride solution at a concentration of 0.1 mg/mL) was administered. The dose was 0.4 mg/kg per day for 2 days on E18 and E19, while the control group was injected with sterile 0.9% sodium chloride solution only (volume 4 mL/kg per day for 2 days). The timing and frequency of LPS dosing were based on protocols reported previously.Citation28,Citation29 LPS doses were determined through preliminary studies and validation to establish concentration gradients. All rats were randomly numbered after surgery using a random number table generated in Excel (Microsoft, Redmond, WA, USA). Random numbers were sorted from small to large and assigned to different groups. Numerical sample identifiers were used during the experimental procedure and data analysis, and the investigators were blinded to the treatment.
Bisex SD rat pups born at E21–E22 were defined as reaching term delivery and included in the study. To ensure consistency between groups, we reduced the number in each group to 7–9 animals at birth. We raised the remaining rats to adulthood or used them for other studies. Finally, 144 full-term neonatal rats were selected for the study, including 88 in the BPD model group and 56 in the control group. Birth was defined as the first time point after birth (i.e., P0, no more than 6 h). Neonatal rats in the LPS-induced BPD group were randomly divided into the BPD, BPD + rapamycin, or BPD + MHY1485 groups. Rapamycin (Cat# S1039; Selleck, USA) and MHY1485 (Cat# S7811; Selleck) were prepared according to the manufacturer’s instructions in a total volume of 50 µL; the neonatal rats from the BPD + rapamycin and BPD + MHY1485 groups received right intraperitoneal injections in the lower abdomen twice (6 h and 24 h after birth). The non-medicated BPD group and the control group were injected with the same volume of sterile water and sterile 0.9% sodium chloride solution. The P1–P5 (i.e., postnatal days 1–5) rodent lungs correspond to 23–32 wk of gestation in humans and therefore are well suited for studying BPD in preterm infants.Citation30 Accordingly, our main study time point was P0–P5.
We followed the ‘Three Rs of Animal Research: (Replace, Reduce, and Refine).’Citation31 To minimize pain, intraperitoneal injection of 4% sodium pentobarbital (200 mg/kg body weight) was used for euthanasia.Citation32 Each injection was performed at the level of the right hip and femoral joint of the abdomen and ∼5 mm to the right of the midline, with the needle at a 45° angle to the body wall. Euthanasia was considered successful in the absence of voluntary movement, loss of righting reflex, and respiratory and heartbeat arrest. Each experiment was performed at least three times to improve data quality. Deceased animals were excluded from this study. This study has not been pre-registered.
Histopathology
The left lung tissues of the neonatal rats were fixed and embedded in paraffin for pathological examination. Briefly, the animals were deeply anesthetized and perfused transcardially with saline, followed by 4% paraformaldehyde. The tissues were fixed at 22 ± 2 °C for 72 h, dehydrated in graded ethanol solutions, embedded in paraffin, and sliced uniformly horizontally after freezing. Hematoxylin and eosin (H&E) staining (Cat# G1121; Solarbio, Beijing, China) was performed to observe the pathological structure of the placenta and the lung tissues and determine the radial alveolar count (RAC) and mean linear intercept (MLI). Images of lung tissues in each section were captured using a Leica microscope (DM4000B; Leica, Wetzlar, Germany). Six discrete sections were collected from each lung tissue, each section was randomly selected with a 20× objective magnification, and a blinded investigator randomly selected microscopic fields using ImageJ 1.8.0 (RRID: SCR_003070; National Institute of Health [NIH], Bethesda, MD, USA).
Terminal deoxynucleotidyl transferase dUTP nick end labeling (TUNEL) assay
The paraffin-embedded sections were deparaffinized, rehydrated, soaked in a solution of 3% H2O2 and methanol for 10 min, and treated with proteinase K (Cat# ST533; Beyotime, Shanghai, China; 20 mg/mL at 22 ± 2 °C). The terminal deoxynucleotidyl transferase enzymatic reaction solution (Cat# KGA700; KeyGen Biotech, Nanjing, China) was added dropwise to the sections and incubated in a humidified chamber at 37 °C for 1 h. Horseradish peroxidase working solution (Cat# KGA700; KeyGen Biotech) was added to the sections and incubated at 37 °C for 30 min. The 3,3′-diaminobenzidine working solution (Cat# KGA700; ZSGB-Bio) was then added after washing to trigger the color reaction. Finally, the sections were observed by a fluorescence microscope (DM4000B; Leica), and TUNEL-positive cells were counted by a researcher blinded to the experimental groups.
Western blotting
Protein concentrations in the right lung tissue of each group were assessed via western blot analysis using the bicinchoninic acid protein assay kit (Cat# PC0020; Solarbio). The samples were separated using 6–15% sodium dodecyl sulfate-polyacrylamide gel electrophoresis (50–70 μg/10 μL), transferred to polyvinylidene difluoride membranes, and incubated overnight at 4 °C with the following primary antibodies: anti-p-Akt (Ser473; 1:1000; RRID: AB_2315049; Cell Signaling Technology), anti-Akt (1:2000; RRID: AB_915783; Cell Signaling Technology), anti-p-mTOR (1:2000; Cat# R25033; ZenBio), anti-mTOR (1:2000; Cat# 380411; ZenBio), anti-p62 (1:2000; RRID: AB_10694431; Proteintech), anti-LC3 (1:1000; RRID: AB_2137737; Proteintech), anti-NLRP3 (1:1000; RRID: AB_2889890; Abcam, Cambridge, UK), anti-caspase-1 (1:1000; RRID: AB_2819181; Santa Cruz Biotechnology), anti-IL-1β (1:1000; Cat# 350193; ZenBio), anti-IL-18 (1:2000; Cat# DF6252; Affinity), anti-GSDMD N-Terminal Antibody (1:1000; Cat# DF13758; Affinity), anti-caspase-11 (1:1000; Cat# R23724; ZenBio), and anti-GAPDH (1:2000; RRID: AB_2107436; Proteintech). The samples were incubated with goat anti-mouse or anti-rabbit IgG secondary antibody conjugated to horseradish peroxidase (HRP; 1:5000; RRID: AB_449890; Abcam) for 2 h. Western blot signals were detected using a bioimaging system (ChemiDoc XRS+; Bio-Rad), and the band intensities were analyzed using the ImageJ software.
Immunofluorescence
Immunofluorescence was performed on the paraffin-embedded left lung tissues collected from neonatal rats in each group. Tissue antigen retrieval was performed by blocking with 10% normal goat serum for 20 min. The tissues were then sliced and incubated with anti-p62 (1:2000; RRID: AB_10694431; Proteintech), anti-NLRP3 (1:100; RRID: AB_2889890; Abcam), anti-caspase-11 (1:100; Cat# R23724; ZenBio), and primary antibody solution for immunofluorescence labeling. The sections were conjugated with an appropriate fluorescent fluorescein isothiocyanate or cyanine 3 secondary antibody (1:300; Cat# 550066; ZenBio) and counterstained with 4,6-diamidino-2-phenylindole to label the DNA. The intensities of the fluorescence signals were measured using ImageJ (NIH) and expressed as the mean fluorescence intensity of the lung tissue.
Statistical analysis
Data were presented as the mean ± standard error of the mean (SEM). Owing to the small sample size, no outlier tests were conducted. The Shapiro–Wilk test was used to assess the normality of the experimental data. Unpaired t-tests were used to compare the means between two groups. Comparisons between multiple groups were performed using one-way analysis of variance (ANOVA) and the least significant difference post-hoc test. All graphs were prepared using the Prism 8.02 software (RRID: SCR_002798; GraphPad, San Diego, CA, USA). SPSS 25.0 (RRID: SCR_002865; IBM, Armonk, NY, USA) was used for statistical analyses. A p < 0.05 indicated a significant difference.
Results
Effect of LPS on placenta and alveolar development in neonatal rats
The placentas of the control group were normal after delivery, with no signs of inflammation or obvious neutrophil infiltration. H&E staining of the placenta of rats in the LPS group showed a significantly higher degree of neutrophil infiltration in each layer of tissue than in the control group (p < 0.0001; ).
Figure 1. Effect of LPS on placenta and alveolar development in neonatal rats. (a, b) H&E staining of placental tissue and comparison of neutrophil counts between two groups. The data were expressed as mean ± SEM for n = 6 rats/group (****p < 0.0001). (c–e) Comparison of pathological manifestations, RAC and MLI values in lung tissues at each postnatal time point (P0, P1.5, P3, P5, and P7) between the control and LPS groups. Data are expressed as the mean ± SEM for n = 6 rats/group (*p < 0.05 at P3, **p < 0.01 at P5, and ***p < 0.05 at P7). P0, P1.5, P3, P5, and P7 are postnatal time points.
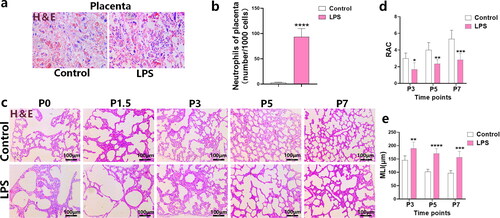
Additionally, H&E staining was used to observe the alveolar development of the LPS and control groups at various birth time points (P0, P1.5, P3, P5, and P7). The RAC was evaluated to compare the number of alveoli between the LPS and control groups; no significant differences were observed at P0 or P1.5. This may be attributed to the number of alveoli approaching 1, which occurs at the beginning of development. However, emphysema was more pronounced, and the alveolar volume was larger in the LPS group than in the control group (). At P3 (p < 0.05), P5 (p < 0.01), and P7 (p < 0.001), the LPS group had a lower RAC value than the control group. Meanwhile, the MLI was significantly higher in the LPS group (P3, p < 0.01; P5, p < 0.0001; P7, p < 0.001), suggesting increased alveolar cross section and volume, which was consistent with the RAC results. Hence, overall, the number of alveoli was significantly reduced, the distribution of alveoli was more disordered, and the degree of emphysema was higher in the LPS group than in the control group. These results suggest that LPS induced BPD development ().
Effects of LPS on pyroptosis in neonatal rat lung tissues
The dead cells in BPD and control lung tissues were counted via TUNEL staining at various birth time points (P0, P1.5, P3, P5, and P7). The results showed that the rate of abnormal cell death in lung tissues of the BPD group significantly increased at P0, and the degree of increase was greater. There was an unexpected decrease in the number of cell death at P1.5 compared to other time points. Cell death significantly increased at P3, P5, and P7 (). The relative levels of the pyroptosis-related proteins Pro-caspase-1, caspase-1, NLRP3, Pro-IL-1β, IL-1β, IL-18/Pro-IL-18, GSDMD-N/GSDMD, Pro-caspase-11 and caspase-11 () in the lung tissue homogenates of the two groups was compared via western blotting. The levels of Pro-caspase-1 (P0, p < 0.05; P3, p < 0.01), caspase-1 (P0, p < 0.001; P3, p < 0.0001), NLRP3 (P0, p < 0.01; P3, p < 0.001), Pro-IL-1β (P0, p < 0.05; P3, p < 0.01), IL-1β (P0, p < 0.0001; P3, p < 0.001), IL-18/Pro-IL-18 (P0, p < 0.001; P3, p < 0.0001), GSDMD-N/GSDMD (P0, p < 0.05; P3, p < 0.0001), Pro-caspase-11 (P0, p < 0.01; P3, p < 0.05), and caspase-11 (P3, p < 0.01) () were significantly increased at P0 and P3 in the BPD group. Furthermore, significant differences in caspase-1 (p < 0.001), IL-1β (p < 0.01), GSDMD-N/GSDMD (p < 0.01), caspase-11 (p < 0.05) () were found at P5. Caspase-11 (P0), Pro-caspase-1, Pro-IL-1β, IL-18/Pro-IL-18, and Pro-caspase-11 (P5) had no significant differences. These results demonstrate that the level of pyroptosis induced by the formation of the NLRP3 inflammasome and caspase-11 inflammasome may be increased primarily during early alveolar development (P0–P3).
Figure 2. Effects of LPS on pyroptosis in neonatal rat lung tissues. (a, b) The ratio of dead cells to all cells (per 1000 cells) at each postnatal time point (P0, P1.5, P3, P5, and P7) in the BPD and control groups was compared using TUNEL staining (n = 6 rats/group; ***p < 0.001 and ****p < 0.0001). (c–f) Relative levels of the indicators related to pyroptosis: Pro-caspase-1, caspase-1, NLRP3, Pro-IL-1β, IL-1β, IL-18/Pro-IL-18, GSDMD-N/GSDMD, Pro-caspase-11, and caspase-11 in lung tissues of the BPD and LPS groups, measured via western blotting. Data are expressed as mean ± SEM for n = 6 rats/group (*p < 0.05, **p < 0.01, ***p < 0.001 and ****p < 0.0001).
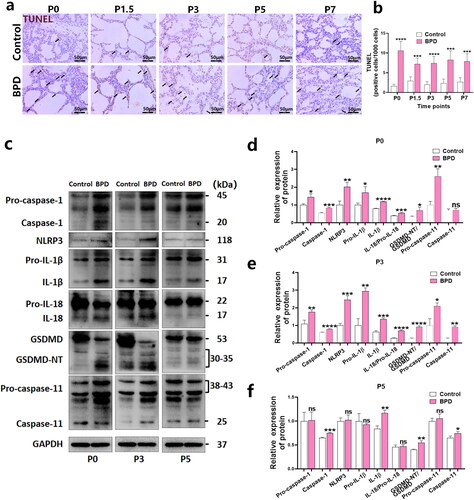
Rapamycin promotes alveolar development in BPD
Compared with that of the BPD group, the RAC value of the BPD + rapamycin group was increased at P2, and significantly increased at P5 (p < 0.05) and P14 (p < 0.05) (, b, d, f). RAC values decreased at P2, P5 and P14 following MHY1485 treatment, although not significantly. Compared with that of the BPD group, the MLI level of the BPD + rapamycin group was significantly decreased at P2 (p < 0.05), P5 (p < 0.001), and P14 (p < 0.01) (, c, e, g). The MLI level increased at P14 following MHY1485 treatment (p < 0.05) (, c, e, g), and at P2 and P5, however, not significantly. After rapamycin treatment, the congestion of the left lung tissue was improved, and the volume of the lung tissue was increased. However, the lung tissue congestion of the MHY1485 + BPD group was more serious, and the lung tissue volume was low (). These results confirm that rapamycin can promote alveolar development in BPD and has therapeutic potential.
Figure 3. Rapamycin promotes alveolar development in BPD. (a-g) RAC and MLI values of the four groups (control, BPD, BPD + rapamycin, and BPD + MHY1485) were compared at P2, P5, and P14 using hematoxylin and eosin staining. Data are expressed as mean ± SEM for n = 6 rats/group (*p < 0.05, **p < 0.01, ***p < 0.001 and ****p < 0.0001). (h) Macroscopic specimens of lung tissue from the four groups are also shown. The morphology, including the size, of the left lung of neonatal rats was observed mainly from the dorsal side. *P represents the statistical data values of the control and BPD groups, BPD and BPD + rapamycin groups, and BPD and BPD + MHY1485 groups.
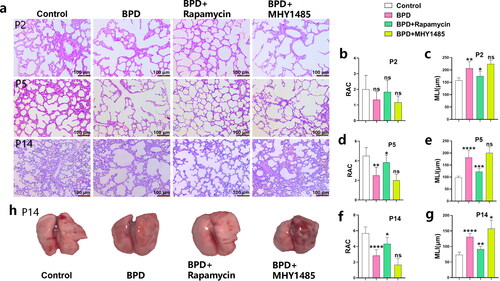
Rapamycin increases autophagy levels in BPD
Compared with those of the control group, activation of the Akt/mTOR signaling pathway in the BPD group was significantly inhibited at P0 and P3, p-Akt/Akt (p < 0.05) and p-mTOR/mTOR (p < 0.01) levels decreased at P0, and were very low at P3 (p-Akt/Akt: p < 0.001; and p-mTOR/mTOR: p < 0.05). However, no significant differences were observed in these indicators at P5 (). This may be a feedback and defense mechanism in neonatal rats. The decreased activation of Akt/mTOR increased the levels of autophagy, which may have had an inhibitory effect on pyroptosis.
Figure 4. Rapamycin upregulates autophagy levels in BPD. (a–d) The phosphorylation levels of Akt and mTOR in lung tissues of the BPD and control groups were detected using western blotting at P0 and P3. Quantitative comparisons of p-Akt/Akt and p-mTOR/mTOR levels were performed. Data are expressed as the mean ± SEM for n = 6 rats/group (*p < 0.05, **p < 0.01, and ***p < 0.001). (e–h) The effects of rapamycin and MHY1485 treatments on p-mTOR/mTOR, p62, and LC3 II/I levels in BPD at P2 were examined. Data are expressed as the mean ± SEM for n = 6 rats/group (*p < 0.05 and **p < 0.01). (i, j) The p62 immunofluorescence images and mean fluorescence intensity analysis showed the differences observed at P2 following rapamycin and MHY1485 treatment. Data are expressed as the mean ± SEM for n = 6 rats/group (****p < 0.0001).
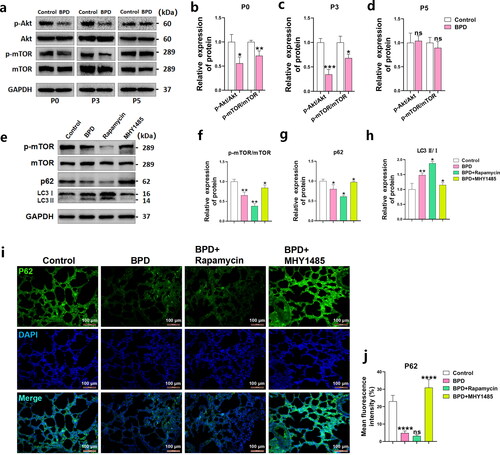
We further explored the relationship between the Akt/mTOR signaling pathway and autophagy in BPD. Western blotting showed that the p-mTOR/mTOR (p < 0.01) and p62 (p < 0.05) levels were decreased, while that of LC3 II/I (p < 0.01) was increased in the BPD group at P2 (48 h; ). The mean fluorescence intensity of p62 (p < 0.0001) in the lungs of the BPD group decreased at P2 (), indicating that autophagy was increased in the lung tissue, consistent with our speculation. After treating newborn rats with BPD twice with rapamycin at 6 and 24 h (P1) post-birth, mTOR phosphorylation (p < 0.01) in the lung tissues was further reduced at P2, while the level of p-mTOR/mTOR (p < 0.05) was restored after administering MHY1485 (). Following rapamycin treatment, along with the decrease in p-mTOR/mTOR levels, the western blotting results showed further decreased levels of p62 (p < 0.05) and LC3 II/I (p < 0.05) in the lung tissues of the BPD group (). The opposite results were observed for p62 (p < 0.05) and LC3 II/I (p < 0.05) following MHY1485 treatment (). Tissue fluorescence images showed that the mean fluorescence intensity of p62 in the BPD + rapamycin group was further decreased, while the level of p62 in the lung tissues significantly increased (p < 0.0001; ) following MHY1485 treatment, which was consistent with the western blot results.
These results suggest that rapamycin can maintain and reduce mTOR activation and upregulate p62 and LC3 II/I-related autophagy levels, while MHY1485 has the opposite effect, breaking the hypophosphorylation state of mTOR.
Rapamycin reduces the activation of the NLRP3 inflammasome and attenuates pyroptosis levels in BPD
TUNEL staining of the lung tissues revealed an increased rate of cell death in the BPD group at P2 (p < 0.01). Meanwhile, rapamycin reduced cell death (p < 0.05) and abnormal cell death was more evident following MHY1485 treatment (p < 0.0001; ). The levels of pyroptosis-related proteins NLRP3 (p < 0.0001), Pro-caspase-1 (p < 0.01), caspase-1 (p < 0.05), Pro-IL-1β (p < 0.0001), IL-1β (p < 0.05), IL-18/pro-IL-18 (p < 0.05) and GSDMD-N/GSDMD (p < 0.01; ) were increased in the lung tissues of the BPD group at P2, indicating that the NLRP3 inflammasome was activated and pyroptosis was initiated. The levels of NLRP3 (p < 0.001), Pro-caspase-1 (p < 0.05), caspase-1 (p < 0.05), Pro-IL-1β (p < 0.001), IL-1β (p < 0.05), IL-18/Pro-IL-18 (p < 0.05), and GSDMD-N/GSDMD (p < 0.05; ) decreased in the BPD + rapamycin group compared with the BPD group. Meanwhile, the BPD + MHY1485 group showed increased levels and activation of NLRP3 (p < 0.05), Pro-caspase-1 (p < 0.05), caspase-1 (p < 0.001), IL-1β (p < 0.05), and IL-18/Pro-IL-18 (p < 0.01), whereas no differences were observed in Pro-IL-1β and GSDMD-N/GSDMD expression (). Moreover, immunofluorescence staining showed that NLRP3 levels in the BPD group increased significantly at P2 (p < 0.0001). Rapamycin significantly reduced NLRP3 inflammasome activation (p < 0.05), while MHY1485 had the opposite effect (p < 0.001; ). These results suggest that rapamycin attenuated NLRP3 inflammasome-induced pyroptosis and reduced the inflammatory response in BPD lung tissues at P2, while MHY1485 aggravated pyroptosis.
Figure 5. Rapamycin reduces NLRP3 inflammasome activation and attenuates pyroptosis levels in BPD. (a, b) TUNEL-positive cells rates (per 1000 cells) were counted to evaluate the degree of pyroptosis in lung tissues of four groups (control, BPD, BPD + rapamycin, and BPD + MHY1485) at P2. Data are expressed as the mean ± SEM for n = 6 rats/group (*p < 0.05, **p < 0.01, and ****p < 0.0001). (c–j) The relative levels of Pro-caspase-1, caspase-1, NLRP3, Pro-IL-1β, IL-1β, IL-18/Pro-IL-18, GSDMD-N/GSDMD, Pro-caspase-11, and caspase-11 in these four groups was detected using western blotting; the grey value was determined. Data are expressed as the mean ± SEM for n = 6 rats/group (*p < 0.05, **p < 0.01, ***p < 0.001, and ****p < 0.0001). (k l) Tissue immunofluorescence was used to analyze differences in NLRP3 levels among groups. Data are expressed as the mean ± SEM for n = 6 rats/group (*p < 0.05, ***p < 0.001, and ****p < 0.0001).
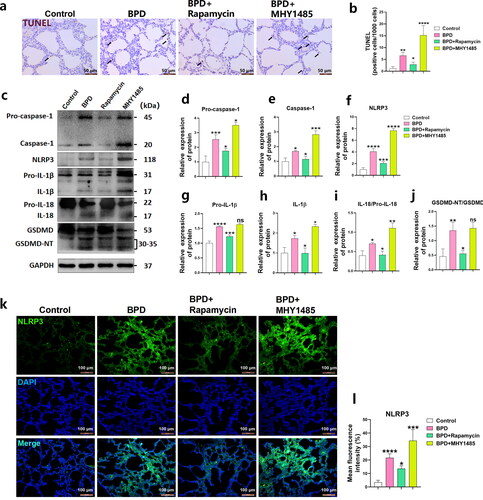
Rapamycin reduces caspase-11 inflammasome production in BPD
Western blotting showed that Pro-caspase-11 (p < 0.0001) and caspase-11 (p < 0.0001) levels () in neonatal lung tissues from rats with BPD significantly increased at P2, consistent with the immunological results (p < 0.0001; ). The levels of Pro-caspase-11 (p < 0.01) and caspase-11 (p < 0.01) were decreased in the BPD + rapamycin group (), consistent with the immunofluorescence results (p < 0.001; ). Activation of the caspase-11 inflammasome was aggravated in the BPD + MHY1485 group (p < 0.05) (), while the average immune strength value of caspase-11 increased (p < 0.0001; ). These results indicated that rapamycin also inhibited the non-canonical pathway of pyroptosis induced by the caspase-11 inflammasome in the lung tissues of neonatal rats with BPD at P2, while MHY1485 had the opposite effect.
Figure 6. Rapamycin reduces caspase-11 inflammasome production in BPD. (a–c) The relative levels of Pro-caspase-11 and caspase-11 in the four groups (control, BPD, BPD + rapamycin, and BPD + MHY1485) was detected using western blotting; the differences in grey value among the groups were compared. Data are expressed as the mean ± SEM for n = 6 rats/group (*p < 0.05, **p < 0.01, and ****p < 0.0001). (d, e) Tissue immunofluorescence was used to analyze differences in caspase-11 levels among groups. Data are expressed as the mean ± SEM for n = 6 rats/group (***p < 0.001, and ****p < 0.0001).
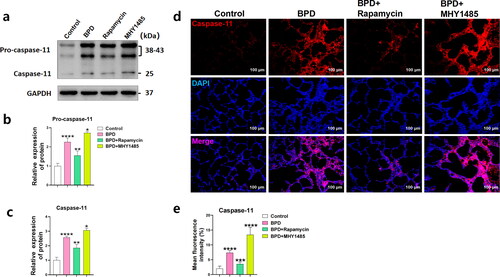
Discussion
BPD is a serious chronic lung disease in premature infants with a prevalence rate of 11–50% across developed and developing countries.Citation1 Nearly 50 000 extremely preterm infants are born annually in the United States, of which approximately 35% (18 000) present with BPD.Citation33 BPD is also a risk factor for serious complications, such as pulmonary hypertension, cardiac dysfunction, chronic obstructive pulmonary disease, and neurodevelopmental disorders.Citation1,Citation2,Citation34,Citation35 Children with BPD clinically exhibit fewer, larger, and more simplified alveoli, mild thickening of the smooth muscle, and pathological findings of fewer but deformed arteries.Citation36,Citation37 BPD pathogenesis includes damage to the immature lung caused by various unfavorable factors, including oxygen toxicity, barotrauma or volume trauma, intrauterine inflammation, and sepsis, based on genetic susceptibility, low gestational age, and abnormal repair of lung tissue after injury.Citation36,Citation38,Citation39 Intrauterine infection can lead to intrauterine inflammation, which is not only an important independent risk factor for preterm birth, but also plays an important role in the occurrence and development of BPD.Citation38,Citation39 However, the pathogenic mechanism of intrauterine infection remains unclear; therefore, it is necessary to clarify its mechanism and seek possible treatment strategies.
In this study, a neonatal model of BPD was successfully established via intraperitoneal injection of LPS to pregnant rats. Our results suggest that in the critical period of alveolar development (P1–P5),Citation30 LPS can significantly reduce the RAC value and increase the MLI level () of the BPD group, causing a decreased number of alveoli, a simpler alveolar structure, smaller blood vessels in the alveolar wall, and interstitial pulmonary hyperplasia in the BPD group. Previous studies have found increased pyroptosis in animal models of hyperoxia lung injury and LPS-induced acute lung injury.Citation16–20 Pyroptosis induced by the NLRP3 inflammasomeCitation40,Citation41 and the caspase-11 inflammasomeCitation42,Citation43 have also been reported in several other inflammatory models as a detrimental factor for normal tissue differentiation and development. Our study found that cell death significantly increased in the lung tissues of neonatal rats in the BPD group at each time point (P0, P1.5, P3, P5, and P7; ). The levels of Pro-caspase-1, caspase-1, NLRP3, Pro-IL-1β, IL-1β, IL-18/Pro-IL-18, GSDMD-N/GSDMD, Pro-caspase-11, and caspase-11 increased at P0 and P3 (), while those of caspase-11 (P0), Pro-caspase-1, Pro-IL-1β, IL-18/Pro-IL-18, and Pro-caspase-11 (P5) did not differ significantly (). Only significant differences in caspase-1, IL-1β, GSDMD-N/GSDMD, caspase-11 were detected (). Hence, pyroptosis occurred at the early stage of alveolar development (P0–P3) in the lung tissues of the LPS-induced BPD neonatal rat model, which may account for the slow increase in = alveoli number. At P3–P5, the pyroptosis level gradually decreased with a weakening of the influence imposed by intrauterine inflammation and the recovery of neonatal rats; accordingly, we did not focus on this period.
The Akt/mTOR signaling pathway was significantly inhibited during the early stage of alveolar development (P0, P2, and P3; ) in the LPS-induced neonatal rat model of BPD. LPS can significantly inhibit Akt/mTOR signaling and reduce mTOR activation.Citation44,Citation45 With the reduction in p-mTOR levels, the level of autophagy in tissues increases. During autophagy, the light chain of the microtubule-associated protein 3 (LC3) is converted from Pro-LC3 to LC3-I, which is processed into LC3-II and converted to LC3-I.Citation46–48 Localized on the autophagosome membrane, LC3-II is degraded and recycled via autophagy. The interaction of p62 with LC3 plays a key role in the formation and degradation of autophagosomes; the higher the level of autophagy, the more p62 is degraded. Therefore, determining LC3-II/LC3-I and p62 levels is a reliable method for monitoring the entire autophagy process.Citation46–48 In our study, the level of autophagy in the lung tissues of neonatal rats with BPD increased at P2, while those of p62 decreased, and the ratio of LC3 II/I increased. Autophagy inhibits pyroptosis;Citation22–27 therefore, we speculate that it may be a self-defense or feedback mechanism of neonatal rats to cope with pyroptosis. Accordingly, we explored this possible mechanism using the mTOR inhibitor rapamycin and the mTOR agonist MHY1485 in early alveolar development (6 and 24 h post birth) in BPD pups.
Our results show that rapamycin, as an enhancer of autophagy, can promote alveolar development to some extent (at P14; ), while MHY1485 has the opposite effect on alveolar development (at P14) (). Following administration of rapamycin, p62 levels in BPD lung tissues further decreased at P2, while the LC3 II/I ratio increased, suggesting that the level of autophagy increased, and MHY1485 had the opposite effect (). The protective effect of rapamycin on BPD can be attributed to its inhibition of the NLRP3 inflammasome-induced classical pyroptosis pathway and the caspase-11 inflammasome-induced non-canonical pyroptosis pathway by continuously enhancing the level of autophagy in the early stage of alveolar development. This study showed that, while the level of autophagy increased after using rapamycin, those of Pro-caspase-1, caspase-1, NLRP3, Pro-IL-1β, IL-1β, IL-18/Pro-IL-18, GSDMD-N/GSDMD (), Pro-caspase-11, and caspase-11 () were significantly inhibited, and the number of cells undergoing pyroptosis was reduced (). Interestingly, MHY1485 can suppress the level of autophagy upregulated in the lung tissues of neonatal rats with BPD due to decreased mTOR phosphorylation, which also weakens the feedback protection mechanism of offspring against pyroptosis and increases pyroptosis. Our findings suggest that the administration of MHY1485 early in alveolar development may deleteriously affect BPD. Rapamycin can prevent the development of lung tissues of neonatal rats from being affected by pyroptosis in the early stage of alveolar development to some extent.
Conclusions
Our results indicate that rapamycin can reduce the occurrence of NLRP3 inflammasome- and caspase-11 inflammasome-mediated pyroptosis by increasing the level of mTOR-induced autophagy in the early stage of alveolar development in the neonatal rat model of BPD caused by LPS-induced intrauterine infection and ultimately promote the normal development and maturation of alveolar tissue (). These findings suggest rapamycin as a potential drug to prevent BPD after intrauterine infection.
Authors’ contributions
FZ conceptualized and designed the study, validated the data, analyzed and curated the data, performed the experiments, performed software-related analysis, and wrote and revised the manuscript. LL conceptualized the study, validated and curated the data, acquired funding, obtained resources, revised the manuscript, supervised the study, and performed visualization and administration of the project. MW, ZL, YF, ZG, YZ, and LH performed the experiments and wrote the original draft of the manuscript. JD performed the experiment and revised the manuscript. All authors read and approved the final manuscript.
Acknowledgments
We thank Editage for their assistance with English language editing.
Disclosure statement
The authors report there are no competing interests to declare.
Additional information
Funding
References
- Thébaud B, Goss KN, Laughon M, et al. Bronchopulmonary dysplasia. Nat Rev Dis Primers. 2019;5(1):78. doi:10.1038/s41572-019-0127-7.
- Gilfillan M, Bhandari A, Bhandari V. Diagnosis and management of bronchopulmonary dysplasia. BMJ. 2021;375:n1974. doi:10.1136/bmj.n1974.
- Lui K, Lee SK, Kusuda S, et al. Trends in outcomes for neonates born very preterm and very low birth weight in 11 high-income countries. J Pediatr. 2019;215:32–40.e14. doi:10.1016/j.jpeds.2019.08.020.
- Stoll BJ, Hansen NI, Bell EF, et al. Trends in care practices, morbidity, and mortality of extremely preterm neonates, 1993–2012. JAMA. 2015;314(10):1039–1051. doi:10.1001/jama.2015.10244.
- Davidson LM, Berkelhamer SK. Bronchopulmonary dysplasia: chronic lung disease of infancy and long-term pulmonary outcomes. J Clin Med. 2017;6(1):4. doi:10.3390/jcm6010004.
- Katz TA, Vliegenthart RJS, Aarnoudse-Moens CSH, et al. Severity of bronchopulmonary dysplasia and neurodevelopmental outcome at 2 and 5 years corrected age. J Pediatr. 2022;243:40–46.e2. doi:10.1016/j.jpeds.2021.12.018.
- Abiramalatha T, Ramaswamy VV, Bandyopadhyay T, et al. Interventions to prevent bronchopulmonary dysplasia in preterm neonates: an umbrella review of systematic reviews and meta-analyses. JAMA Pediatr. 2022;176(5):502–516. doi:10.1001/jamapediatrics.2021.6619.
- Marc I, Piedboeuf B, Lacaze-Masmonteil T, et al. Effect of maternal docosahexaenoic acid supplementation on bronchopulmonary dysplasia-free survival in breastfed preterm infants: a randomized clinical trial. JAMA. 2020;324(2):157–167. doi:10.1001/jama.2020.8896.
- Watterberg KL, Walsh MC, Li L, et al. Hydrocortisone to improve survival without bronchopulmonary dysplasia. N Engl J Med. 2022;386(12):1121–1131. doi:10.1056/NEJMoa2114897.
- Morrow LA, Wagner BD, Ingram DA, et al. Antenatal determinants of bronchopulmonary dysplasia and late respiratory disease in preterm infants. Am J Respir Crit Care Med. 2017;196(3):364–374. doi:10.1164/rccm.201612-2414OC.
- Taglauer E, Abman SH, Keller RL. Recent advances in antenatal factors predisposing to bronchopulmonary dysplasia. Semin Perinatol. 2018;42(7):413–424. doi:10.1053/j.semperi.2018.09.002.
- Lapcharoensap W, Gage SC, Kan P, et al. Hospital variation and risk factors for bronchopulmonary dysplasia in a population-based cohort. JAMA Pediatr. 2015;169(2):e143676. doi:10.1001/jamapediatrics.2014.3676.
- Bergsbaken T, Fink SL, Cookson BT. Pyroptosis: host cell death and inflammation. Nat Rev Microbiol. 2009;7(2):99–109. doi:10.1038/nrmicro2070.
- Jorgensen I, Miao EA. Pyroptotic cell death defends against intracellular pathogens. Immunol Rev. 2015;265(1):130–142. doi:10.1111/imr.12287.
- de Gassart A, Martinon F. Pyroptosis: caspase-11 unlocks the gates of death. Immunity. 2015;43(5):835–837. doi:10.1016/j.immuni.2015.10.024.
- Li D, Li C, Wang T, et al. Geranylgeranyl diphosphate synthase 1 knockdown suppresses NLRP3 inflammasome activity via promoting autophagy in sepsis-induced acute lung injury. Int Immunopharmacol. 2021;100:108106. doi:10.1016/j.intimp.2021.108106.
- Liao J, Kapadia VS, Brown LS, et al. The NLRP3 inflammasome is critically involved in the development of bronchopulmonary dysplasia. Nat Commun. 2015;6(1):8977. doi:10.1038/ncomms9977.
- Peng W, Peng F, Lou Y, et al. Autophagy alleviates mitochondrial DAMP-induced acute lung injury by inhibiting NLRP3 inflammasome. Life Sci. 2021;265:118833. doi:10.1016/j.lfs.2020.118833.
- Tayman C, Çakır U, Akduman H, Karabulut Ş, Çağlayan M. The therapeutic effect of apocynin against hyperoxy and inflammation-induced lung injury. Int Immunopharmacol. 2021;101(Pt A):108190. doi:10.1016/j.intimp.2021.108190.
- Zhao W, Ma L, Cai C, Gong X. Caffeine inhibits NLRP3 inflammasome activation by suppressing MAPK/NF-κB and A2aR signaling in LPS-induced THP-1 macrophages. Int J Biol Sci. 2019;15(8):1571–1581. doi:10.7150/ijbs.34211.
- Yu L, Chen Y, Tooze SA. Autophagy pathway: cellular and molecular mechanisms. Autophagy. 2018;14(2):207–215. doi:10.1080/15548627.2017.1378838.
- Biasizzo M, Kopitar-Jerala N. Interplay between NLRP3 inflammasome and autophagy. Front Immunol. 2020;11:591803. doi:10.3389/fimmu.2020.591803.
- Sun Q, Fan J, Billiar TR, Scott MJ. Inflammasome and autophagy regulation - a two-way street. Mol Med. 2017;23(1):188–195. doi:10.2119/molmed.2017.00077.
- Biasizzo M, Trstenjak-Prebanda M, Dolinar K, et al. Cystatin C deficiency increases LPS-induced sepsis and NLRP3 inflammasome activation in mice. Cells. 2021;10(8):2071. doi:10.3390/cells10082071.
- Ghoneim ME, Abdallah DM, Shebl AM, El-Abhar HS. The interrupted cross-talk of inflammatory and oxidative stress trajectories signifies the effect of artesunate against hepatic ischemia/reperfusion-induced inflammasomopathy. Toxicol Appl Pharmacol. 2020;409:115309. doi:10.1016/j.taap.2020.115309.
- Cosin-Roger J, Simmen S, Melhem H, et al. Hypoxia ameliorates intestinal inflammation through NLRP3/mTOR downregulation and autophagy activation. Nat Commun. 2017;8(1):98. doi:10.1038/s41467-017-00213-3.
- Ip WKE, Hoshi N, Shouval DS, Snapper S, Medzhitov R. Anti-inflammatory effect of IL-10 mediated by metabolic reprogramming of macrophages. Science. 2017;356(6337):513–519. doi:10.1126/science.aal3535.
- Liu C, Chen Z, Li W, Huang L, Zhang Y. Vitamin D enhances alveolar development in antenatal lipopolysaccharide-treated rats through the suppression of interferon-γ production. Front Immunol. 2017;8:1923. doi:10.3389/fimmu.2017.01923.
- Zhou O, You J, Xu X, et al. Microvesicles derived from human umbilical cord mesenchymal stem cells enhance alveolar type II cell proliferation and attenuate lung inflammation in a rat model of bronchopulmonary dysplasia. Stem Cells Int. 2022;2022:8465294.
- Nardiello C, Mižíková I, Morty RE. Looking ahead: where to next for animal models of bronchopulmonary dysplasia? Cell Tissue Res. 2017;367(3):457–468. doi:10.1007/s00441-016-2534-3.
- Curzer HJ, Perry G, Wallace MC, Perry D. The three Rs of animal research: what they mean for the institutional animal care and use committee and why. Sci Eng Ethics. 2016;22(2):549–565. doi:10.1007/s11948-015-9659-8.
- Laferriere CA, Pang DS. Review of intraperitoneal injection of sodium pentobarbital as a method of euthanasia in laboratory rodents. J Am Assoc Lab Anim Sci. 2020;59(3):254–263. doi:10.30802/AALAS-JAALAS-19-000081.
- Collaco JM, McGrath-Morrow SA. Bronchopulmonary dysplasia as a determinant of respiratory outcomes in adult life. Pediatr Pulmonol. 2021;56(11):3464–3471. doi:10.1002/ppul.25301.
- Charafeddine L, D'Angio CT, Phelps DL. Atypical chronic lung disease patterns in neonates. Pediatrics. 1999;103(4 Pt 1):759–765. doi:10.1542/peds.103.4.759.
- DeMauro SB. Neurodevelopmental outcomes of infants with bronchopulmonary dysplasia. Pediatr Pulmonol. 2021;56(11):3509–3517. doi:10.1002/ppul.25381.
- Ahn SY, Chang YS, Lee MH, et al. Stem cells for bronchopulmonary dysplasia in preterm infants: a randomized controlled phase II trial. Stem Cells Transl Med. 2021;10(8):1129–1137. doi:10.1002/sctm.20-0330.
- Rojas MA, Gonzalez A, Bancalari E, Claure N, Poole C, Silva-Neto G. Changing trends in the epidemiology and pathogenesis of neonatal chronic lung disease. J Pediatr. 1995;126(4):605–610. doi:10.1016/s0022-3476(95)70362-4.
- Liu Y, Hoyo C, Murphy S, et al. DNA methylation at imprint regulatory regions in preterm birth and infection. Am J Obstet Gynecol. 2013;208(5):395.e1–7–395.e7. doi:10.1016/j.ajog.2013.02.006.
- Thomas W, Speer CP. Chorioamnionitis is essential in the evolution of bronchopulmonary dysplasia – the case in favour. Paediatr Respir Rev. 2014;15(1):49–52. doi:10.1016/j.prrv.2013.09.004.
- Faro J, Romero R, Schwenkel G, et al. Intra-amniotic inflammation induces preterm birth by activating the NLRP3 inflammasome. Biol Reprod. 2019;100(5):1290–1305. doi:10.1093/biolre/ioy261.
- Tersigni C, D'Ippolito S, Di Nicuolo F, et al. Recurrent pregnancy loss is associated to leaky gut: a novel pathogenic model of endometrium inflammation? J Transl Med. 2018;16(1):102. doi:10.1186/s12967-018-1482-y.
- Qiu X, Cheng X, Zhang J, Yuan C, Zhao M, Yang X. Ethyl pyruvate confers protection against endotoxemia and sepsis by inhibiting caspase-11-dependent cell pyroptosis. Int Immunopharmacol. 2020;78:106016. doi:10.1016/j.intimp.2019.106016.
- Wu Y, Pan B, Zhang Z, et al. Caspase-4/11-mediated pulmonary artery endothelial cell pyroptosis contributes to pulmonary arterial hypertension. Hypertension. 2022;79(3):536–548. doi:10.1161/HYPERTENSIONAHA.121.17868.
- Hseu YC, Tseng YF, Pandey S, et al. Coenzyme Q0 inhibits NLRP3 inflammasome activation through mitophagy induction in LPS/ATP-stimulated macrophages. Oxid Med Cell Longev. 2022;2022:4266214–4266215. doi:10.1155/2022/4266214.
- Turnquist HR, Cardinal J, Macedo C, et al. mTOR and GSK-3 shape the CD4+ T-cell stimulatory and differentiation capacity of myeloid DCs after exposure to LPS. Blood. 2010;115(23):4758–4769. doi:10.1182/blood-2009-10-251488.
- Lyu W, Li Q, Wang Y, et al. Computational design of binder as the LC3-p62 protein–protein interaction. Bioorg Chem. 2021;115:105241. doi:10.1016/j.bioorg.2021.105241.
- Salazar G, Cullen A, Huang J, et al. SQSTM1/p62 and PPARGC1A/PGC-1alpha at the interface of autophagy and vascular senescence. Autophagy. 2020;16(6):1092–1110. doi:10.1080/15548627.2019.1659612.
- Rahman MA, Cho Y, Nam G, Rhim H. Antioxidant compound, oxyresveratrol, inhibits APP production through the AMPK/ULK1/mTOR-mediated autophagy pathway in mouse cortical astrocytes. Antioxidants (Basel). 2021;10(3):408. doi:10.3390/antiox10030408.