Abstract
Purpose: The presented theoretical study investigates the influence of CFMA-like electromagnetic microstrip applicators (MAs) on ionizing radiation intensity and depth radiation dose distribution (DRDD) in irradiated tissues which are tightly covered with a MA.
Methods: It is shown that at relatively low photon energy (<200 keV) the MA does not affect noticeable the profile of the DRDD curve and does not lead to skin overdosing. Nevertheless, it significantly (up to 20–35%) decreases the low energy ionizing radiation intensity. For high energy photons (>1 MeV), on the contrary, the decrease of radiation intensity, caused by the MA, is small (3–10%), but the profile of the DRDD curve, calculated by means of the Monte-Carlo method, is significantly affected.
Results: The radiation dose maximum is shifted to the skin, resulting in possible skin overdosing. Radiation absorption characteristics of MA are calculated and compared with published parameters of EM horn and US applicators now in use for external simultaneous radiation and hyperthermia (ESRH) procedures. The MA provides the minor ionizing radiation absorption. Due to it and owing to their conformability with the tissue surface the MAs would not require any additional means or devices to be used for ESRH treatment procedures with any common ionizing radiation equipment.
Conclusions: The necessity of development means for decreasing the time of radiation equipment occupation during ESRH procedures is pointed out.
Introduction
The highest thermal enhancement ratio (TER) of ionizing radiation efficacy, produced by hyperthermia, occurs when radiation and hyperthermia act simultaneously (further on—Simultaneous Radiation and Hyperthermia—SRH) Citation[1–5]. The external SRH (ESRH) clinical trials were pioneered in the USA in 1992 Citation[5–7]. Promising results of a series of ESRH and brachytherapy SRH trials were reported at the 8th and 9th ICHO sessions in Korea and Saint-Louis (USA) Citation[8–10]. Conducted trials confirmed the clinical feasibility of SRH. Nevertheless, there remain many problems which need further investigation and development to make this treatment modality clinically acceptable and significant. Among them the problem of hyperthermic EM applicators suited for SRH Citation[5]. Intensive investigations and successful development of micro-strip and interstitial applicators for simultaneous brachytherapy and hyperthermia have been carried out recently Citation[11–15], Citation[19]. EM horn and ultrasound (US) applicators are used for ESRH treatments. They are located in either of two positions. In the first, ‘en face’, position axes of the ionizing radiation beam and of the heating EM (or US) energy flow are parallel. In this case the ionizing radiation penetrates through elements of the EM (or US) applicator. In the second position axes of the ionizing radiation and EM energy flow are mutually perpendicular. The ‘en face’ ESRH order is more effective and allows treating a significantly more number of malignant tumour locations Citation[5]. Applicators intended for ‘en face’ ESRH must introduce minimum attenuation of the ionizing radiation and minimally perturb the depth dose distribution in tissues Citation[5], Citation[16]. The microstrip EM applicators are best suited to meet these requirements Citation[17].
This article shall try to perform a quantitative analysis of the radiation absorption characteristics of EM micro-strip applicators for ESRH procedures, as well as analyse their influence on ionizing radiation dose distribution in tissues. The analysis is performed with different photon energies. CFMA-like applicators Citation[17], Citation[18] are chosen as specific samples for calculations.
Absorption of X, γ-rays by EM microwave micro-strip applicators
As a rule, a micro-strip applicator is a layered structure Citation[13], Citation[14] which consists of micro-strip copper film electrodes, dielectric substrate layers, a silicon rubber frame and a thin silicon rubber bolus, a deionized water (or mineral oil) layer in the silicon bolus ().
Attenuation of the monoenergetic ionizing radiation intensity, I, occurs in every layer in accordance with the exponential function:where i is the number of the layer being penetrated by the radiation; Ii is the ionizing radiation intensity behind the ith layer; μi is the linear attenuation coefficient of the ith layer material and di is its thickness.
If the applicator structure consists of m layers, then the output ionizing radiation intensity It at the surface of the irradiated tissue would be equal to the product:where I0 is the initial radiation intensity at the outer surface of the micro-strip applicator.
Calculations of X, γ-rays intensity attenuation caused by the applicators were performed for three energy levels of the ionizing radiation: 150 keV (X-rays) and 1.3 and 10 MeV (γ-radiation). The estimation of X-rays attenuation was done taking into account the peculiarities of the X-ray spectrum, which is excited by the 150 kV electron beam (the spectrum extends from 30 keV up to 150 keV Citation[20]) and selected by 6 mm Al filters.
demonstrates the calculated X, γ-rays absorption characteristics of a micro-strip CFMA-SRH applicator prototype.
Table I. Ionizing radiation intensity absorption produced by each element and of the micro-strip CFMA-SRH applicator prototype as a whole.
Three inferences could be made from the data of . First, the water (or oil) layer in the applicator bolus contributes the main part to radiation absorption produced by the micro-strip applicator. Secondly, the low energy (100–200 keV) radiation is absorbed by the MA significantly (20–30%), whereas absorption of the high energy radiation (10 MeV) does not exceed 2–5% dependent on the water layer thickness. Thirdly, the radiation absorption contributed by an applicator with a silicon oil bolus is greater than that of the applicator with a water bolus of the same thickness d. The absorption increases by a factor of 1.15 if d = 0.5 cm and by a factor of 1.3 if d = 1 cm.
Superficial distribution of X, γ-rays absorption over the applicator area
Calculations of absorption properties of the micro-strip applicators were done () with the assumption that all structures of the MA have a constant thickness over the entire surface of the applicator. However, as is seen in , there are constant thickness heterogeneities defined by the applicator design: these are gaps in the electrodes and, especially, the silicon projections on the applicator frame which are immersed in the bolus water layer (WL) and serve to prevent the collapse of the bolus.
As follows from , the absence of the copper film in the gaps changes the total radiation absorption by the MA less than 0.1–4% (depending on incident energy level) and, thus, might be neglected. The extent to which the absorption is affected by the silicon projections was evaluated by means of the EGS-Nova program and turned out to be less than 1% and can be neglected also.
The third and main source heterogeneity can be the WL in the bolus. If the irradiated tissue surface is not an even flat plate and the water bolus of the MA conforms with it, the thickness of the WL over the applicator aperture would be heterogeneous. It can be shown that a 5 mm variation in water layer thickness of a 10 mm regular water layer leads to a change of radiation absorption ∼7% at low energy radiation (100–200 keV), 3% at 1.25 MeV and ≤1% at 10 MeV and at higher photon energies as the absorption decrease with increase of the photon energy.
Computer simulation of depth radiation dose distribution (DRDD) in tissues covered with a micro-strip applicator
Due to boundary conditions at the surface of the irradiated tissue, the maximum of the radiation dose, created by a single ionizing radiation beam, is localized at some depth zm in the tissue. The depth zm depends on the photon energy. The shift of the dose maximum from the skin decreases the probability of skin overdosing when superficial or deep located tumours are treated with high photon energy radiotherapy. The situation changes drastically when any material (in this case the applicator) lies tightly at the irradiated skin: the dose maximum, if it exists, is shifted to the skin. The magnitude of the shift Δzm depends, except on the photon energy, on the radiological parameters of the material lying on the skin.
If the photon energy is ≤250 keV, the extrapolated electron range Re in tissues is ≤0.05 cm and, thus, the dose maximum without the applicator practically lies on (or in) the skin. Thus, the applicator does not affect the profile of the depth dose distribution. Moreover, the applicator does not change noticeably the relation Ds /Dt (where Dt is the radiation dose in the tumour, Ds is the dose at the skin), though the time of irradiation must be increased by 20–30% to reach the prescribed value of Dt.
The situation changes radically when the photon energy is near or exceeds 1 MeV.
The case of high energy photons. Calculation techniques and modeling
For computer simulations of depth radiation dose distribution (DRDD) in tissue, the tissues were modelled by a water phantom (WPh). Calculations were performed taking into account the real parameters of the heterogeneous CFMA-SRH applicator prototype (see ). To simplify the calculations, a model of the real prototype was elaborated on.
The model geometrical sizes are equal to those of the real applicator, but its material is a homogeneous liquid medium with a specific density ρm. The value of ρm is chosen so as to provide equality of the radiological thickness of the model and that of the heterogeneous applicator. In this case (the CFMA-SRH applicator prototype) the magnitude of ρm turns out to be equal to 1.158 g cm−3.
Calculations of DRDD were performed by the Monte-Carlo method following codes MCNP4C2 and EGS-Nova. The code EGS-Nova has been modernized to allow performing calculations not only of mono-directional, but also of divergent beams.
Results
By means of the program MCN P4C2 the photon energy spectrum of the bremsstrahlung produced by a medical accelerator Clinac 2100C (Varian) with a maximum electron energy of 6 MeV was calculated. The calculated energy spectrum created by this unit is shown in . It is close to the spectrum given in Bagheri and Rogers Citation[21] except the data in the range of 5.5–6.0 MeV. It is necessary to mention a pronounced maximum of the photon energy spectrum in the interval 0.5–1.0 MeV.
Figure 2. Calculated photon energy spectrum Clinac 2100C accelerator (Varian) 6 MV. - - - Data from Bagheri and Rogers Citation[21].
![Figure 2. Calculated photon energy spectrum Clinac 2100C accelerator (Varian) 6 MV. - - - Data from Bagheri and Rogers Citation[21].](/cms/asset/bbf2ed60-2d03-44e4-8907-266016c9c80c/ihyt_a_166148_f0002_b.gif)
The calculated DRDD created in a water phantom (WPh) by this bremsstrahlung beam (considered as a point collimated source) (PCS) is shown in . The distance SSD between the PCS and the WPh was taken as 100 cm and the collimation angle equals 3.8°, thus the dimensions of the radiated field at the WPh surface were 11.5 × 11.5 cm2. The DRDD was calculated without and with the CFMA-SRH applicator prototype over the WPh surface. The profile of both DRDD curves does not differ qualitatively at depths of z more than 3 cm.
Figure 3. Depth radiation dose distribution (DRDD) in a water phantom, zm = 2 cm. Bremsstrahlung. Accelerating voltage 6 MV. - - - DRDD with a micro-strip applicator on the phantom surface; —— DRDD with a ‘naked’ phantom surface.
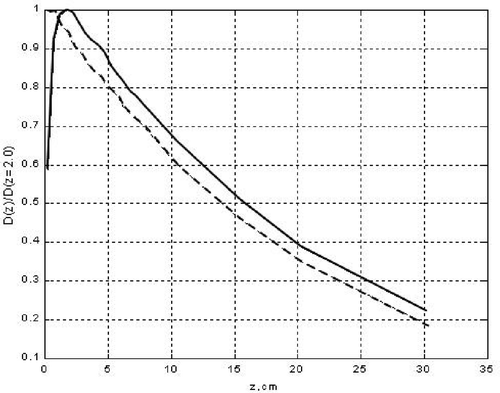
At less depth and, particularly, near the WPh surface the distinction between the curves becomes drastic: the presence of the MA over the WPh surface increases the superficial (skin) dose Ds at ΔDs ≈ 60%.
Analogous calculations were carried out for a PCS with a monoenergetic 1.25 MeV photon beam. Results are shown in . The main difference from is the magnitude of ΔDs which in this case equals ≈20%.
Figure 4. Depth radiation dose distribution (DRDD) in a water phantom, zm = 0.5 cm. Monoenergetic beam 1.25 MeV. - - - DRDD with a micro-strip applicator on the phantom surface; —— DRDD with a ‘naked’ phantom surface.
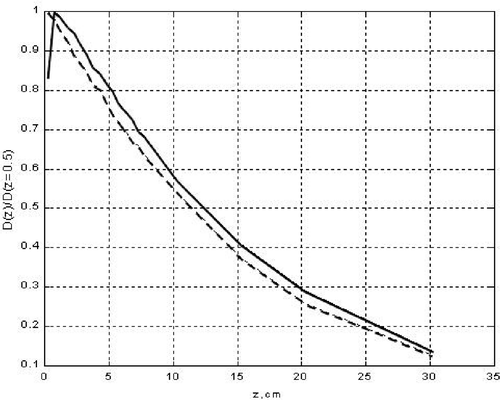
To evaluate the validity of the proposed homogeneous model, equivalent to the heterogeneous applicator, comparative calculations were performed of DRDD when the WPh was coated either by the heterogeneous CFMA-SRH applicator prototype or by the homogeneous applicator model. Calculations were done by means of a modernized version of the EGS-Nova code utilizing the Monte-Carlo method.
DRDD created in the WPh by bremsstrahlung beams of the accelerator Clinac 2100C (6 MV) and of a home 18MV accelerator, so as DRDD created by monoenergetic 1.25 MeV beams, were calculated in three combinations: (a) without any applicator (PCS–air gap–WPh); (b) with a CFMA-SRH applicator coating on the WPh (PCS–air gap–CFMA-SRH applicator–WPh); and (c) with a homogeneous applicator model on the WPh (PCS–air gap–homogeneous applicator model–WPh). The sizes of the air gap and the PCS collimation angle were taken as typical ones for irradiation treatments on medical accelerators.
Results of the calculations, summarized in and , showed that the influence of the heterogeneous CFMA-SRH applicator on dose distribution in the WPh is rather precisely (with an error less than 1.5%) reproduced by the homogeneous model in a wide range of energies.
Table II. Dose distributions in a water phantom for different irradiation combinations; field size 10 × 10 cm2; SSD = 85 cm (E = 1.25 MeV) and 100 cm (V = 6 MV); 10−14 (Gy cm2).
Table III. Dose distributions in a water phantom for different irradiation combinations; field size 10 × 10 cm2, SSD = 100 cm and V0 = 18 MV, 10−14 (Gy cm2).
Discussion and conclusion
There can be made some inferences from the accomplished calculations concerning the influence of micro-strip applicators (MA) on the surface intensity and on DRDD in irradiated tissue phantoms in case radiation penetrating through the MA. First of all, it must be stated that the water layer (WL) in the MA water bolus contributes the most part to the attenuation produced by the MA as a whole. Apart of the WL thickness, its (WL) relative contribution to the total attenuation (TA) depends drastically on the photon energy and constitutes ∼45–75% of the TA at low energies of X-rays (80–200 keV), ∼55–85% at energies of 1 MeV, about and over 75–95% at energies ≥10 MeV. Nevertheless, the total attenuation of the radiation intensity, introduced by the MA as a whole, decreases with the increase of the photon energy being equal to ∼20–35% at low energies (≤200 keV) and diminishing to 11–2% at photon energies exceeding 1 MeV. For comparison, the hyperthermia applicators used at present in clinics for external SRH treatments attenuate the high energy (≈1 MeV, Clinac 6) ionizing radiation by ∼20% (EM microwave wave-guide type applicators) and up to 50% (US applicators) Citation[7]. This is significantly more than the calculated attenuation introduced by the MA.
The dependence on photon energy of MAs influence on the DRDD profile is quite opposite to that of the attenuation effect of the MAs. At low photon energies the MA coating on the WPh does not noticeably change the DRDD curve profile in the WPh and, thus, does not lead to surface (skin) overdosing. Moreover, in this case the ratio of the surface (skin) dose, Ds, to the depth (tumour) dose, Dt, remains practically unchanged.
On the contrary, when the photon energy is near or over 1 MeV the MA coating on the WPh significantly changes the profile of the DRDD-curve in it. Particularly, it shifts the point zm of the dose maximum, Dm, to the WPh surface and, thus, may lead to essential overdosing of the skin. As follows from and and and the higher the photon energy of the ionizing beam, the greater the relative (as compared with the ‘naked’ WPh case) skin overdosing. If it is assumed that the skin thickness is <1 mm, then the magnitude of skin relative overdosing, following the data in and , is: at 1.25 MeV ∼20%, at 6 MV bremsstrahlung this figure increases near to 60% and at 18 MV the relative skin overdosing is more than two-fold. At the same time, the DRDD profile, as well as the absolute dose values at tissue depths more than 1 cm, do not differ essentially from one another for all three (a), (b), (c)-combinations of ionizing radiation in photon energy intervals from 1 MeV up to 6 MeV and at depths more than 2 cm up to18 MeV.
Skin overdosing is the main restriction on MA usage for SRH ‘en face’ treatments with high energy (≈1 MeV) photon single beam radiation. If the range of overdosing becomes unacceptable, then multi-beam or rotational ionizing radiation equipment is necessary to accomplish simultaneous radiation and hyperthermia treatments with micro-strip applicators.
Besides, the same difficulties with skin overdosing arise for any hyperthermic applicator type which water (or oil) bolus is in tight contact with the irradiated skin area.
The MAs have several important advantages in comparison with EM wave-guide or US applicators. First, the constructive elements of the MA introduce a uniform attenuation of the ionizing radiation over the whole applicator area. Only when the MA water bolus conforms an uneven tissue surface may various WL thicknesses arise and, as a consequence, variations of the ionizing radiation intensity over the irradiated area. This effect is predominantly pronounced at low (<200 keV) photon energies and contributes ∼1.2–1.4% intensity attenuation per every mm of the WL (if its thickness does not exceed 2.5 cm). At high energies this value diminishes to ≤0.5% per mm at 1.25 MeV and to 0.2% per mm at 10 MeV. To avoid this effect completely, the design of the MA must provide a constant thickness of the WL over the whole aperture of the MA, independent of the structure of the tissue surface.
The main advantage of the MAs is that they do not require any additional means or devices to be adapted to any radiation equipment to provide simultaneous radiation and hyperthermia treatments. Of course, influence of the MA on depth radiation dose distribution in tissues must be taken into account when planning the SRH treatment procedure. Herewith the uniformity of the radiological properties of the MA over its whole aperture is of great importance. It is important to note that the dose depth distribution in a phantom calculated for real heterogeneous applicators and for its homogeneous model, mentioned above, practically coincides with a difference of less than 1%. This fact permits one to essentially simplify the procedure of dose planning for ESRH.
To the advantageous properties of MAs there must be added the low absorption of ionizing radiation intensity which is 2–11% at high photon energies exceeding 1 MeV.
Summing up the results of the presented investigations, it can be concluded that micro-strip applicators and particularly contact flexible micro-strip applicators are to be an effective and convenient tool to provide hyperthermia treatments for malignant tumours simultaneously with external radiotherapy.
One of the main usually mentioned problems associated with the clinical application of the ESRH treatment modality consists of the necessity to occupy for ESRH the very expensive and intensively used in clinics radiation equipment for a long time. Of course, this problem is very important and must (and one is convinced would) be solved. However, this subject is beyond the frames of this article.
CFMA-SRH applicators for simultaneous external radiation and hyperthermia with parameters discussed in this article are now under development.
Acknowledgements
The authors are thankful to Ms Marina Kartashova for her help in preparing this paper. These investigations were supported by the ISTC Grant on Project 2221.
References
- Overgaard J. The current and potential role of hyperthermia in radiotherapy. Int J Radiat Oncol Biol Phys 1989; 16: 538–549
- Raaphorst GP. Fundamental aspects of hyperthermic biology. An introduction to the practical aspects of clinical hyperthermia, Field SB, Hand JW. Taylor & Francis, London 1990; 27–29
- Field SB. In vivo aspects of hyperthermic oncology. An introduction to the practical aspects of clinical hyperthermia, Field SB, Hand JW. Taylor & Francis, London 1990; 64–65
- Horsman MR, Overgaard J. The influence of nicotinamid and hyperthermia on the radiation response of a murine tumour and normal tissue. In Book of Abstracts, 15th Annual Meeting of the ESHO. Oxford, UK 3–6 September, 1995; 12
- Moros EG, Straube WL, Klein EE, Myerson RJ. Clinical system for simultaneous external superficial microwave hyperthermia and cobalt-60 radiation. Int J Hyperthermia 1995; 11: 11–26
- Myerson RJ, Straube WL, Moros EG, Emami BN, Lee HK, Perez CA, Taylor ME. Simultaneous superficial hyperthermia and external radiotherapy. Int J Hyperthermia 1999; 15: 251–266
- Straube WL, Klein EE, Moros EG, Low DA, Myerson RJ. Dosimetry and techniques for simultaneous hyperthermia and external beam radiation therapy. Int J Hyperthermia 2001; 17: 48–62
- Corry PM, Armour EP, Desten D, Martinez A. Simultaneous hyperthermia and brachytherapy. Abstract Book, The 8th ICHO. Kyong-Ju, Korea 26–29 April, 2000; 83
- Myerson RJ, Straube WL, Moros EG. Effect of increasing number of hyperthermia sessions for patients receiving simultaneous US hyperthermia and radiation. Abstract Book, The 8th ICHO. Kyong-Ju, Korea 26–29 April, 2000; 199
- Myerson RJ, Straube WL, Taylor M, Zoberi I, Moros EG. Simultaneous radiation therapy and hyperthermia in the elective treatment of subclinical disease in high risk breast carcinoma. Abstracts, The 9th ICHO. St. Louis, MissouriUSA, 20–24 April 2004. 80
- Rossetto F, Stauffer PR. Theoretical characterization of dual concentric conductor microwave array applicators for hyperthermia at 433 MHz. Int J Hyperthermia 2001; 17: 258–270
- Stauffer P, Schlorf J, Taschereau R, Juang T, Neuman D, Maccarini P, Pouliot J, Hsu J. Combination applicator for simultaneous heat and radiation. Int Conf IEEE Eng Med Biol. IEEE press, San Francisco 2004; 4
- Turner P, Hegman M, Youd T. Microwave interstitial hyperthermia and HDR brachytherapy integration. Abstracts, The 9th ICHO. St. Louis, MissouriUSA 20–24 April, 2004; 92
- Stauffer PR, Schlorf JL, Taschereau R, Pouliot J, Neuman D, Hsu J. Clinical implementation of conformal applicators for simultaneous heat and brachytherapy of superficial disease. Abstracts, The 9th ICHO. St. Louis, MissouriUSA 20–24 April, 2004; 162
- Taschereau R, Stauffer PR, Hsu IC, Schlorff JL, Milligan AJ, Pouliot J. Radiation dosimetry of a conformal heat-brachytherapy applicator. Tech Cancer Res Treat 2004; 3: 347–358
- Myerson RJ, Straube WL, Moros EG, Myerson RJ. The effect of hyperthermia applicators on radiation surface dose for simultaneous thermoradiotherapy. Abstracts, The 9th ICHO. St. Louis, MissouriUSA 20–24 April, 2004; 90
- Gelvich EA. Some technical aspects of simultaneous external radiotherapy on the background of EM hyperthermia. Abstracts, 17th Annual Meeting of the ESHO. Centre Alexis Vautrin, NancyFrance 2–5 September, 1998; 23
- Gelvich EA, Mazokhin VN. Contact flexible microstrip applicators (CFMA) in a range from microwaves up to short waves. IEEE Trans Biomed Eng 2002; 49: 1015–1023
- Stauffer PR, Rossetto F, Leoncini M, Gentilli GB. Radiation patterns of dual concentric conductor microstrip antennas for superficial hyperthermia. IEEE Trans Biomed Eng 1998; 45: 605–613
- Vasilyev VN, Lebedyev LA, Sodorin VP, Stavitsky RV. Radiation spectra of Roentgen installations. Handbook. Energoatomisdat, Moscow 1990; 144, (in Russian)
- Bagheri D, Rogers DWO. Monte-Carlo calculation of nine megavoltage beam spectra using beam code. Med Phys 2002; 29: 391–401