Abstract
Purpose: To use an established computer simulation model of radiofrequency (RF) ablation to further characterize the effect of varied perfusion on RF heating for commonly used RF durations and electrode types, and different tumor sizes.
Methods: Computer simulation of RF heating using 2-D and 3-D finite element analysis (Etherm) was performed. Simulated RF application was systematically modeled on clinically relevant application parameters for a range of inner tumor perfusion (0–5 kg/m3-s) and outer normal surrounding tissue perfusion (0–5 kg/m3-s) for internally cooled 3-cm single and 2.5-cm cluster electrodes over a range of tumor diameters (2–5 cm), and RF application times (5–60 min; n = 4618 simulations). Tissue heating patterns and the time required to heat the entire tumor ± a 5-mm margin to >50°C were assessed. Three-dimensional surface response contours were generated, and linear and higher order curve-fitting was performed.
Results: For both electrodes, increasing overall tissue perfusion exponentially decreased the overall distance of the 50°C isotherm (R2 = 0.94). Simultaneously, increasing overall perfusion exponentially decreased the time required to achieve thermal equilibrium (R2 = 0.94). Furthermore, the relative effect of inner and outer perfusion varied with increasing tumor size. For smaller tumors (2 cm diameter, 3-cm single; 2–3 cm diameter, cluster), the ability and time to achieve tumor ablation was largely determined by the outer tissue perfusion value. However, for larger tumors (4–5 cm diameter single; 5 cm diameter cluster), inner tumor perfusion had the predominant effect.
Conclusion: Computer modeling demonstrates that perfusion reduces both RF coagulation and the time to achieve thermal equilibrium. These results further show the importance of considering not only tumor perfusion, but also size (in addition to background tissue perfusion) when attempting to predict the effect of perfusion on RF heating and ablation times.
Introduction
Radiofrequency (RF) ablation has been used for the treatment of focal primary and secondary liver malignancies as a minimally invasive, image-guided alternative to standard surgical resection Citation[1–3]. Indeed, for hepatocellular carcinoma, long-term studies have demonstrated survival outcomes similar to surgical resection for focal tumors <3 cm in diam Citation[3], Citation[4]. However, an inability to achieve complete ablation of larger tumors (3–5 cm in diameter) persists, secondary to unfavorable tissue characteristics, most notably blood flow, and limitations to intratumoral RF energy depositio Citation[5], Citation[6]. Even so, RF ablation is being applied for the minimally invasive, image-guided treatment of focal tumors in a wide range of tissue sites, including kidney Citation[7], liver Citation[3], Citation[8], lu Citation[9], Citation[10] and bone Citation[11], Citation[12] with markedly varied tissue characteristics.
RF ablation-induced coagulation is dependent upon achieving focal temperatures usually exceeding 50°C for 4–6 min within designated tissue Citation[13]. The principles of this are characterized by Pennes et al. Citation[14] in the Bioheat equation and further simplified to describe the basic relationship guiding thermal ablation induced coagulation necrosis as ‘coagulation necrosis = energy deposited X local tissue interactions—heat loss” Citation[15]. RF and other methods of thermal ablation require a defined thermal dose be delivered to tissue to achieve coagulation Citation[16]. Thus, for in vivo tissues, a heat-sink effect from flowing blood limits the extent of tissue in which the necessary cytotoxic temperatures can be achieved. The role of perfusion-mediated vascular cooling in limiting RF-induced tissue heating and the ability to mitigate its effects somewhat with mechanical or pharmacologic modulation of perfusion are well-established in both in vivo experimental and multiple clinical studies in vascular organs such as the liver and kidney Citation[17–19]. Based upon this, intrinsic tissue characteristics such as perfusion-mediated tissue cooling are important in predicting RF-induced tissue heating patterns Citation[20]. Hence, further characterization is warranted, but challenging using clinical data or in vivo models alone, given the innate heterogeneity of perfusion of the tumors studied and other confounding variables such as variable electrical Citation[21] and thermal conductivity Citation[20].
The purpose of this study is to use an established computer simulation model of RF energy (Etherm) to characterize further the effect of varied perfusion on RF heating for commonly used RF durations and electrode types, and different tumor sizes.
Materials and methods
Overview of experimental design
Computer simulation of RF heating using a finite element analysis program (Etherm) was performed in three phases. In the first phase, simulation of RF heating patterns over time was performed by studying the effect of ablation duration 0–60 min for a range of perfusion values (0–10 kg/m3-s; n = 5 parameters) for a single compartment model (i.e. no difference in tissue characteristics between ‘tumor’ and surrounding ‘background’ tissue) for an internally cooled 3-cm single and 2.5-cm cluster electrode (n = 10). In the second phase, two compartment simulations were performed for varied inner ‘tumor’ (0–5 kg/m3-s) and outer ‘background’ (0–5 kg/m3-s) perfusions for simulated tumor sizes of 2–5 cm to determine the effects of different perfusion in the tumor and background tissue. A matrix of eight inner and eight outer perfusion values (n = 64) were studied for each of four tumor diameters for the two electrodes (total n = 64 × 4 × 2 = 512). In the third phase, simulations were performed characterizing the effect of different tumor and tissue perfusions (0–5 kg/m3-s) on the time (6–20 min) necessary to heat completely theoretical 2–5-cm diameter tumors with or without a 5-mm ablative margin to 50°C using a 3-cm single or 2.5-cm cluster electrode. Five additional RF durations (6, 8, 10, 16, and 20 min) were studied for the original four tumor sizes of Phase II (5 × 64 matrix parameters × 4 tumors × 2 electrodes = 2560 simulations). Two additional tumor sizes were also studied for an additional 1536 simulations, for a total of 4608 simulations [including phase II data] that were analyzed in Phase III.
Computer simulation model
All software was provided by Field Precision (Albuquerque, NM) Citation[22]. A two-dimensional finite-element model of coupled RF electric fields and thermal transport was used as a platform for performing computer simulated RF ablation of 3-cm single electrodes, while a three-dimensional model was used for the more complex geometry of cluster electrodes. Comparative temperature profiles were generated using computer simulation of the electrostatic equations coupled to Pennes’ Bio-Heat equation (Etherm) Citation[22], Citation[23]. Prior versions of this model have been validated as predictive of tissue heating and ablation in agar phantoms and non-perfused ex vivo livCitation[21], Citation[24], Citation[25]. The computer model has been modified with a general control algorithm to permit the generation of matrices of large volumes of data from which we fashioned temperature response surfaces. The model has been previously modified to be biologically relevant, most notably by constructing a temperature limitation control Citation[20]. Specifically, temperature within the model is not allowed to exceed 100°C, the temperature at which boiling limits the ability to apply RF energy Citation[26]. The model automatically provides the necessary current to achieve up to 100°C heating in the system to a maximum specified current (as would occur clinically based upon maximum current output of presently available commercial RF generators). When 100°C is achieved at any point in space or time during the simulated RF application, the program automatically reduces the applied voltage to the maximum level, at which no tissue is heated beyond this temperature. Thus, our model mimics the biologic and physical constraints present during actual RF ablation including experimentally realistic temperature and current limitations. Additionally, for the purposes of this study, simulated perfusion was eliminated for all mesh points that reached a temperature of 60°C to simulate coagulation eliminated microvascular perfusion.
Finite-element analysis software
To simulate a 3-cm single probe of 0.146 cm (17 gauge) in diameter in tumors of different sizes (2 cm, 3 cm, 4 cm) in a cylindrical background tissue of 10 cm in length and 14 cm in diameter, we used the computer program MESH version 5.0 (Field Precision) to generate conformal triangular meshes. For 2-D finite-element calculation for both the electrical and thermal solutions, we used ETHERM to perform coupled electro-thermal solutions using the geometry generated by MESH (). To simulate a 2.5-cm cluster electrode in tumors of different sizes in a cylindrical background tissue of 10 cm in length and 14 cm in diameter, we used the computer program METAMESH (Field Precision) to generate conformal meshes of hexahedrons for the 3-D calculations (). For 3-D finite-element calculation for both the electrical and thermal solutions, we used ETHERM3 to perform coupled electro-thermal solutions using the geometry generated by METAMESH. We used ETHERM3 to perform coupled electro-thermal solutions using the geometry generated by METAMESH.
Figure 1. Finite element model meshes. This is an illustrative representation of both the (a) 2-D finite element model used for simulating a 3-cm single internally cooled electrode (red arrow) in a 4-cm tumor (yellow tumor, white arrow), and (b) the 3-D finite element model used to simulate a 2.5-cm cluster electrode (red arrow) in a 4-cm tumor (white arrow).
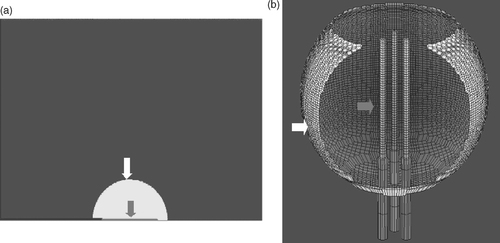
Simulated RF application
RF parameters were selected to best simulate clinical practice in liver using a commonly used electrode system. This included the use of internally cooled electrodes (10°C) of 3 cm tip length, a generator maximum current output of 2000 mA and RF application for 12 min at 500 KHz Citation[2], Citation[5]. The time of RF application was varied where specified.
Two-compartment model
In order to assess the wide range of clinically relevant tumor and background perfusions, a previously reported Citation[20] template was used that enabled us to study the effects of a defined parameter space (response contour) consisting of a varied range of tissue perfusion for inner ‘tumors’ of variable radius, surrounded by uniform tissue parenchyma. A variable sized two-compartment model with a 10 cm radius was used. At the innermost region of the cylindrically symmetrical model, is a 3-cm long, 0.146-cm (17G) wide electrode or 2.5-cm cluster electrodes (). Surrounding this is an inner compartment of 4 cm length of a variable diameter measuring 1–5 cm (simulating tumors of corresponding diameters). For two-compartment modeling, a range of varied inner (0–5 kg/m3-s) and outer (0–5 kg/m3-s) perfusions were used for each tumor size. The remainder of the underlying tissue characteristics were standardized and identical for both the inner and outer compartments and were selected to approximate normal liver parenchyma (including specific permittivity of 2000, specific heat of 3400 J/Kg-°C, baseline electrical conductivity of 0.5 S/m, and thermal conductivity of 0.5 W/m-°C) Citation[27].
Additionally, for 3-cm single and 2.5-cm cluster electrodes, the 50°C isotherm was calculated for a range of inner and outer tissue perfusions (0–5 kg/m3-s) for RF application times of 6, 8, 10, 12, 16 and 20 min for tumor sizes of 2–5 cm.
Outcome measurements
The primary measurement used to evaluate RF heating patterns was the 50°C isotherm (i.e. the distance from RF electrode at which the temperature of the tissue is simulated to be 50°C at the end of the RF ablation session), as this has been used in the past to represent the most clinically relevant outcome (i.e. the temperature above which the tissue is completely irreversibly coagulated) Citation[20]. This measure was also used to calculate an estimated coagulation diameter where specified. For two-compartment models, the times required to achieve a minimum 50°C temperature at specified distances from the electrode, specifically at the tumor margin and an ‘ablative margin’ of normal tissue (defined as 5 mm of surrounding normal tissue beyond the tumor margin Citation[28]) was also recorded ().
Figure 2. Measurement of temperatures at the tumor and ablative margin. In this example, a 3-cm single electrode is inserted into a 3-cm tumor (light green area). The times needed to achieve a 50°C isotherm at the margin of this tumor as well as at the ablative margin 5-mm (red zone) surrounding the tumor were calculated.
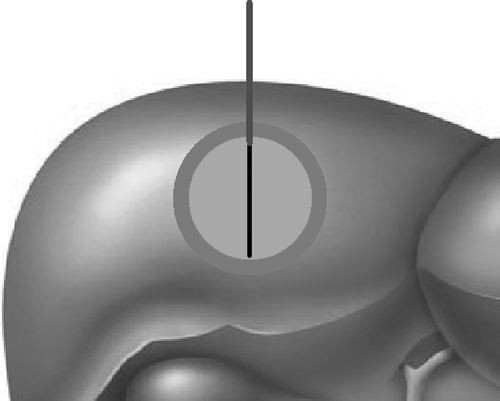
For the initial single compartment studies, computer-simulated heating profiles were also created for a distance of 1, 2, 3 and 4 cm away from the electrode. Additionally, the time required to reach thermal equilibrium, as defined by the time to reach 90% or 95% of the maximum 50°C isotherm during RF ablation, was determined.
Data analysis
Non-linear curve fitting including exponential and power function (Origin 6.1, Microcal Software Inc., Northampton, MA) was performed for plotting the effects of varied perfusions on the time required to reach 50°C isotherms, with determination of goodness of fit. Subsequently, for both single and cluster electrodes, resultant 50°C isotherms were used to construct three-dimensional contour maps (surface responses) expressing the relationship between inner and outer perfusion and heating, using Dataplot graphing software (D-plot, Vicksburg, MS). Separate surface responses were created for different tumor sizes (2–5 cm diameter) and single and cluster electrodes. Additionally, surface contour plots were also constructed demonstrated the 50°C isotherms for varying RF times (6–20 min) for both single and cluster electrodes. The ‘approximate’ known perfusion of three different, and clinically relevant, tumor types and their background tissues were overlaid onto these graphs (renal cell carcinoma in normal kidney: inner perfusion 3 kg/m3-s, outer perfusion 3.34 kg/m3-s, hepatocellular carcinoma in cirrhotic liver: inner perfusion 3 kg/m3-s, and outer perfusion 1 kg/m3-s, and colorectal metastasis to normal liver: inner perfusion 0.5 kg/m3-s, outer perfusion 3.34 kg/m3-s) Citation[27], Citation[29].
Results
Phase I: Effect of perfusion on RF heating patterns in a single compartment model
Computer modeling demonstrated that increasing perfusion from 0 kg/m3-s to 10 kg/m3-s reduced the size of the heated zone (as evidenced by decreases in the distances at which the 50°C isotherm was identified; , ). For example, for heating with the 3-cm single probe at 3600 s, the 50°C isotherm was located 3.3 cm from the electrode for 0 kg/m3-s compared with just 1.1 cm for higher perfusion (10 kg/m3-s). Greater coagulation diameters were observed using the cluster electrode compared with the single electrode (p < 0.05) (). Additionally, increasing perfusion reduced the time required to achieve thermal equilibrium in a negative exponential fashion ( and , ).
Figure 3. The effect of tissue perfusion on the size of the ablation zone, and the time required to reach thermal equilibrium. The effects of varied perfusion from 0 kg/m3-s to 10 kg/m3-s on tissue heating are displayed for (a) 3-cm single and (b) 2.5 cm cluster electrodes. Black arrowheads represent the time necessary to achieve 95% of the largest 50°C isotherm; while open arrows represent 90% of the largest 50°C isotherm. Increasing perfusion not only reduces the size of the heated zone, but also reduces the time necessary to achieve 90% or 95% of the 50°C isotherm. Additionally, cluster electrodes substantially increase the size of the 50°C isotherm.
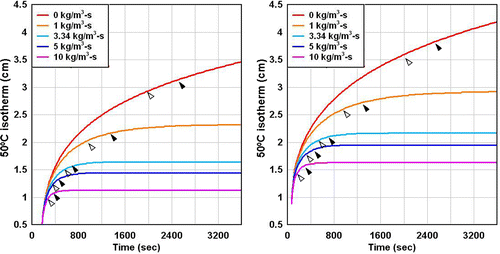
Figure 4. Tissue perfusion vs. 50°C isotherm. In a single-compartment model, the size of the ablation zone decreases with increasing tissue perfusion. As tissue perfusion increases (0–10 kg/m3-s), the size of the ablation zone (demonstrated here as the 50°C isotherm) decreases. This is represented by negative exponential correlations between perfusion and the 50°C isotherm (i.e. 90% and 95% of maximum) for both 3-cm single and 2.5-cm cluster electrodes.
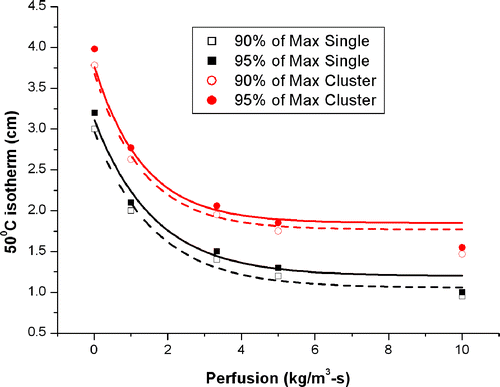
Figure 5. Tissue perfusion vs. time to reach thermal equilibrium. The time required to achieve thermal equilibrium decreases in a negative exponential manner with increasing tissue perfusion. Increasing tissue perfusion (0–10 kg/m3-s) decreases the time required to achieve tissue thermal equilibrium (90% and 95% of maximum thermal equilibrium).
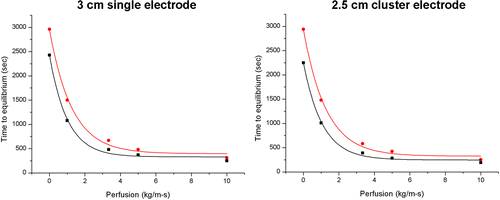
Table I. Effect of perfusion on the size of the ablation zone and time required to achieve thermal equilibrium. Increasing tissue perfusion (0–10 kg/m3-s) for both a 3-cm single and 2.5-cm cluster electrode decreases both the 50°C isotherm and the time required to achieve thermal equilibrium in a negative exponential manner.
Phase II: Effect of varied inner and outer tissue perfusions in a two-compartment model
The surface response maps generated with varying inner and outer perfusion for different tumor diameters for ablation times of 12 min () identified several trends. For smaller tumors, results were dependent largely on background tissue perfusion. For larger tumors (greater than 4 cm for single and 5 cm for cluster electrodes), the location of the 50°C isotherm (i.e. the amount of ablation) depended largely on the perfusion within the tumor (). For intermediate sized tumors, there was a mixed pattern, as both tumor and background tissue perfusion effected the time to achieve ablation.
Figure 6. Surface contour maps for RF ablation of varied tumor and tissue perfusion. This set of surface contour maps plots the different 50°C isotherms at 12 min for varied inner (i.e. tumor; X-axis) and outer perfusions (i.e. background tissues; Y-axis) (0–5 kg/m3-s) over a range of tumor sizes (2–5 cm in diameter) for both 3-cm single and 2.5-cm cluster electrodes. For smaller tumors, the horizontal pattern denotes that results were dependent largely on background tissue perfusion. For larger tumors (greater than 4 cm for single and 5 cm for cluster electrodes), the vertical orientation of the 50°C isotherm (i.e. the amount of ablation) depended largely on the perfusion within the tumor.
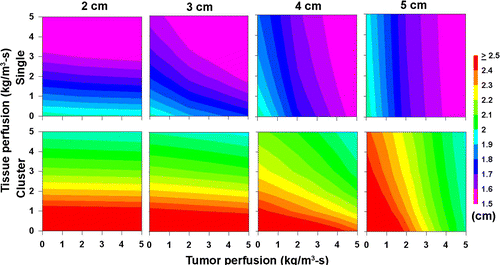
Phase III: Effect of perfusion on achieving tumor ablation with or without an ablative margin
Although phase I demonstrated that thermal equilibrium is reached faster with increasing perfusion, for each tumor of a given size increasing ablation time enables successful ablation of a greater portion of the perfusion surface domain ( and ). Additionally, these plots further demonstrated in general that the thermal profile is much steeper when ablating just the tumor compared with the tumor and ablative margin ( and ). In other words, achieving complete ablation of the tumor alone was more dependent on inner/tumor perfusion, while achieving ablation of the tumor and 5-mm ablative margin was more dependent on the outer perfusion.
Figure 7. Effect of perfusion and the time required to ablate with RF energy. The 50°C isotherm for achieving complete tumor ablation without (red isobars) and with (blue isobars) a 5-mm ablative margin was plotted for different RF ablation times (6–20 min) and different tumor sizes (2–4 cm diameter) using a 3-cm single electrode. Tumor and background tissue perfusion were varied 0–5 kg/m3-s. For 2- and 3-cm tumors, the red line denotes that complete ablation of the tumor could be achieved at 6 min of RF ablation time for all levels of outer and inner perfusion. With increasing tumor size, achieving complete ablation requires longer RF times, achieving an ablative margin becomes increasingly difficult, and the effect becomes increasingly dependent on inner perfusion. Additionally, there are almost no inner/outer perfusion levels at which an ablative margin could be achieved for tumors equal to, or larger than 3.5 cm.
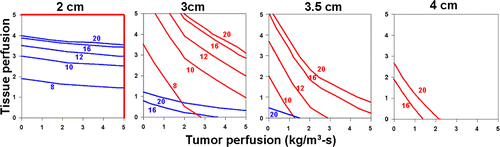
Figure 8. Effect of perfusion on the time required to achieve ablation for a cluster electrode. The 50°C isotherm for achieving complete tumor ablation without (red isobars) and with (blue isobars) a 5-mm ablative margin was plotted for different RF ablation times (6–20 min) and different tumor sizes (3–5 cm diameter) and varied inner and outer perfusion 0–5 kg/m3-s using a 2.5-cm cluster electrode. The approximate inner/outer perfusions for varying types of commonly treated tumors overlay the 4.5-cm tumor surface response contour and are represented as: Colorectal metastases to normal liver (M), renal cell carcinoma (R), and hepatocellular carcinoma (H). The time and ability to completely treat tumor or achieve a 5-mm ablative margin varies considerably based upon tumor type and size. In this scenario, achieving complete ablation of the colorectal metastasis would take 10 min, while the HCC would require greater than 12 min of heating and the RCC in excess of 20 min.
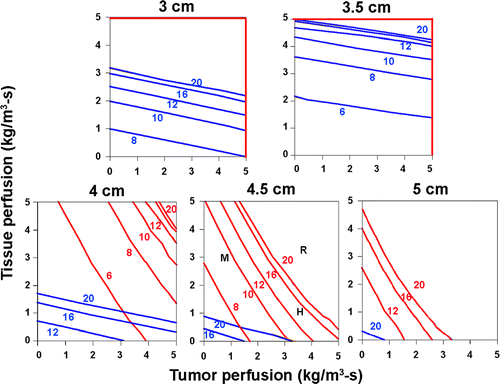
In these modeling scenarios, tumors with a diameter of 2 cm or less could be completely ablated for a 3-cm single electrode, and tumors with a diameter of 3.5 cm or less for a 2.5-cm cluster electrode. Both inner and outer perfusion had a major impact on the ability to achieve complete ablation and the time necessary to completely treat tumor and/or ablative margin. As expected, lower perfusion and smaller tumor size increased the likelihood of getting the tumor and/or the margin and reduced time.
Accordingly, the time and ability to completely treat tumor or achieve a 5-mm ablative margin varied considerably based upon tumor type and size between HCC in cirrhotic liver (high inner perfusion, low outer perfusion), RCC (high inner perfusion, high outer perfusion), and metastatic colorectal carcinoma to normal liver (low inner perfusion, normal/high outer perfusion) (). For example, achieving complete ablation of a 4.5-cm colorectal metastasis would take 10 min, while the HCC would require more than 12 min of heating and the RCC in excess of 20 min.
Discussion
As RF ablation is increasingly applied in a wide range of tumor and tissue types, further characterization and understanding of the role of intrinsic tumor and tissue characteristics will be required to optimize RF energy delivery paradigms and tumor coagulation. Both experimental and clinical studies have demonstrated that perfusion-mediated vascular cooling significantly decreases overall tissue heating and tumor ablation efficacy Citation[30–33]. Our study uses computer modeling of the Bioheat equation to characterize further the effects of simulated tissue and tumor perfusion on RF heating patterns.
The results of our study demonstrate that increasing overall tissue perfusion exponentially decreases achievable RF tissue heating for both single and cluster electrodes. These findings confirm the results of many experimental and clinical studies that have demonstrated significantly reduced zones of coagulation in tissues with higher perfusion Citation[27], such as liver and kidney, and increased coagulation when RF ablation is combined with perfusion modulators such as mechanical vessel occlusion and pharmacologic agents Citation[31–35]. However, while prior studies have suggested a linear relationship between tissue/tumor perfusion and ablative sizes Citation[16], our results go beyond this to suggest a non-linear, exponential relationship between these variables. We attribute this finding to our ability to interrogate a wider range of perfusion variables more precisely, thereby enabling determination of the true shape of the curve. As such, computer modeling may be beneficial in reducing experimental noise or increasing the number of data points to provide greater insight into physical relationships.
Additionally, our results demonstrate that increased overall tissue perfusion not only reduces the size of the heated area, corresponding to decreased 50°C isotherms, but also decreases the time required to achieve tissue heating. Several currently used clinical RF ablation paradigms apply RF energy for set standard amounts of time (12 min is the most commonly used) Citation[13]. In our study, thermal equilibrium was reached earlier at higher perfusion rates, which suggests that the actual time required to achieve maximum heating probably differs, based in part on tissue perfusion. Therefore, in highly vascular tissues such as liver and kidney, shorter amounts of RF time may yield maximum gain, compared with tissues with relatively smaller amounts of perfusion, such as lung and bone.
Differences in both inner tumor and background tissue perfusion also appear to significantly influence RF heating patterns depending on the size of the tumor. Our results demonstrate that for smaller tumors, the ability and time to achieve tumor ablation was largely determined by background tissue perfusion, whereas for larger tumors, the inner tumor perfusion had the predominant effect on the time to achieve maximum ablation. This has significant potential clinical implications as there are often differences in tumor and background tissue perfusion, especially when comparing primary HCC in cirrhotic liver (high tumor perfusion and low surrounding perfusion) and colorectal metastases to liver (low tumor and normal/high surrounding perfusion) Citation[27]. Thus, optimal heating times for different tumors is likely to vary substantially (6–20 min) from currently suggested guidelines (i.e. 12 min) Citation[13]. Accordingly, one can envision future pre-procedural planning with CT or MR perfusion studies to permit better refinement of ablation algorithms or improve clinical outcome predictability Citation[36], Citation[37]. Furthermore, characterization of varying perfusion on RF-induced tissue heating patterns may ultimately permit extrapolation to other similar and clinically relevant high-temperature point-source thermal ablative therapies from microwave, laser and ultrasound energies Citation[38].
As our study suggests, with further refinement, computer modeling of tumor and surrounding tissue perfusion characteristics for specific tumors, such as renal cell carcinomas or hepatic colorectal metastases, may permit prediction of the likelihood of achieving complete ablation with currently recommended RF ablation strategies (i.e. 3-cm single or 2.5-cm cluster electrode for 12 min), and the optimal (either shorter or longer) times required to treat different tumors. Additionally, as clinical studies have demonstrated, achieving optimal tumor ablation includes ablating at least a 5-mm margin of surrounding normal-appearing background tissue Citation[28], our results demonstrate that differences in tumor/background perfusion can determine if and when ablation of a peripheral margin can be achieved. Given the well-documented difficulties in achieving complete RF ablation of larger tumors, especially in the liver, using differences in tumor/background perfusion in conjunction with tumor size may help to identify which tumors have a higher likelihood for success with RF treatment. Alternatively, in those tumors that are unlikely to be completely ablated with the existing clinical RF paradigms, further modeling may allow us to identify the extent of ablation that can be achieved with a single RF application, which can help design the more robust protocols for ‘overlapping’ ablations Citation[6], Citation[39].
Our results, along with prior studies, demonstrate that generation of surface response maps using data from multiple simulations from computer modeling of the Bioheat equation continues to be a robust strategy for optimizing and evaluating RF ablation techniques. Indeed, in this paper we show how this mapping of trends in tissue heating can help explain why given RF parameters are successful for some clinical scenarios (such as treating a 3-cm HCC with a single electrode) and not others (such as a similarly sized, more vascular RCC). Given that current clinical RF paradigms, and the most clinical experience, are based upon treatment of hepatic tumors, it is likely that optimized RF ablation techniques in other tissues will require differing amounts of RF application time based upon differences in perfusion.
While these initial results give further insight into the role of RF tissue interactions on RF-induced heating, there are several limitations of this study, and further characterization of the effects of tissue perfusion is required. While several prior studies have used this model in characterization of the effects of both electrical and thermal conductivity on RF ablation with good ex vivo correlatio Citation[21], Citation[24], correlation of these findings to in vivo models will be required. Additionally, this study only examines the effects of tissue perfusion, while standardizing other tissue characteristics such as thermal and electrical conductivity. Ultimately, further computer modeling will be required to examine the specific influences of each of these characteristics when varied in a compounded manner. Finally, our modeling assumes that 50°C is an absolute thermal threshold for tissue destruction when, in fact, RF thermal dosimetry is likely to vary, as different tissues demonstrate varying tissue-specific thermal sensitivities. Additionally, we assume tissue perfusion homogeneity and have as of yet not incorporated the potential effect of larger vessels that are known to induce ‘heat-sink’ and influence ablation shape and outcomCitation[19], Citation[40], Citation[41]. Thus, further refinements in defining endpoints may ultimately be needed for true predictability. Regardless, the current strategy of characterizing RF-tissue interactions by isolating and modulating individual parameters has already expanded our understanding of clinical RF ablation.
In conclusion, computer modeling demonstrates that blood flow reduces not only RF coagulation but also the time to achieve thermal equilibrium. These results further show the importance of considering not only tumor blood flow, but also size (in addition to background tissue blood flow) when attempting to predict the effect of perfusion on RF heating and ablation times. Accordingly, computer modeling demonstrates that a greater appreciation of tumor and background tissue blood flow, as well as tumor size, will probably be beneficial in choosing optimal RF application parameters and for improving predictability of RF outcome.
Acknowledgements
This work was supported by a grant from the National Cancer Institute, National Institutes of Health, Bethesda, MD (RO1-CA87992-01A1) and Valleylab, Boulder, CO, USA, and by the Tyco Healthcare/Mallinkrodt/RSNA Research Resident Grant from the RSNA Research & Education Foundation, Oak Brook, IL (RR0603) USA.
References
- Curley SA, Izzo F, Ellis LM, Nicolas Vauthey J, Vallone P. Radiofrequency ablation of hepatocellular cancer in 110 patients with cirrhosis. Ann Surg 2000; 232: 381–391
- Solbiati L, Livraghi T, Goldberg SN, Ierace T, DellaNoce M, Gazelle GS. Percutaneous radiofrequency ablation of hepatic metastases from colorectal cancer: Long-term results in 117 patients. Radiology 2001; 221: 159–166
- Livraghi T, Meloni F, Morabito A, Vettori C. Multimodal image-guided tailored therapy of early and intermediate hepatocellular carcinoma: Long-term survival in the experience of a single radiologic referral center. Liver Transpl 2004; 10(2 Suppl 1)S98–106
- Lencioni R, Cioni D, Crocetti L, Franchini C, Pina CD, Lera J, Bartolozzi C. Early-stage hepatocellular carcinoma in patients with cirrhosis: Long-term results of percutaneous image-guided radiofrequency ablation. Radiology 2005; 234: 961–967
- Livraghi T, Meloni F, Goldberg SN, Lazzaroni S, Solbiati L, Gazelle GS. Hepatocellular carcinoma: Radiofrequency ablation of medium and large lesions. Radiology 2000; 214: 761–768
- Dodd GD, III, Soulen MC, Kane RA, Livraghi T, Lees WR, Yamashita Y, Gillams AR, Karahan OI, Rhim H. Minimally invasive treatment of malignant hepatic tumors: At the threshold of a major breakthrough. Radiographics 2000; 20: 9–27
- Gervais DA, McGovern FJ, Arellano RS, McDougal WS, Mueller PR. Renal cell carcinoma: Clinical experience and technical success with radio-frequency ablation of 42 tumors. Radiology 2003; 226: 417–424
- Solbiati L, Ierace T, Tonolini M, Osti V, Cova L. Radiofrequency thermal ablation of hepatic metastases. Eur J Ultrasound 2001; 13: 149–158
- Dupuy DE, Mayo-Smith WW, Abbott GF, DiPetrillo T. Clinical applications of radio-frequency tumor ablation in the thorax. Radiographics 2002; 22: S259–269
- Zagoria RJ, Chen MY, Kavanagh PV, Torti FM. Radio frequency ablation of lung metastases from renal cell carcinoma. J Urol 2001; 166: 1827–1828
- Dupuy DE, Hong R, Oliver B, Goldberg SN. Radiofrequency ablation of spinal tumors: Temperature distribution in the spinal canal. AJR Am J Roentgenol 2000; 175: 1263–1266
- Rosenthal DI, Hornicek FJ, Wolfe MW, Jennings LC, Gebhardt MC, Mankin HJ. Percutaneous radiofrequency coagulation of osteoid osteoma compared with operative treatment. J Bone Joint Surg Am 1998; 80: 815–821
- Goldberg SN, Gazelle GS, Mueller PR. Thermal ablation therapy for focal malignancy: A unified approach to underlying principles, technqiues, and diagnostic imaging guidance. Am J Radiol 2000; 174: 323–331
- Pennes H. Analysis of tissue and arterial blood temperatures in the resting human forearm. J Appl Physiol 1948; 1: 93–122
- Goldberg SN. Radiofrequency tumor ablation: Principles and techniques. Eur J Ultrasound 2001; 13: 129–147
- Goldberg SN, Hahn PF, Tanabe KK, Mueller PR, Schima W, Athanasoulis CA, Compton CC, Solbiati L, Gazelle GS. Percutaneous radiofrequency tissue ablation: Does perfusion-mediated tissue cooling limit coagulation necrosis?. J Vas Interv Radiol 1998; 9: 101–111
- Goldberg SN, Hahn PF, Halpern EF, Fogle R, Gazelle GS. Radiofrequency tissue ablation: Effect of pharmacologic modulation of blood flow on coagulation diameter. Radiology 1998; 209: 761–769
- Patterson EJ, Scudamore CH, Owen DA, Nagy AG, Buczkowski AK. Radiofrequency ablation of porcine liver in vivo: Effects of blood flow and treatment time on lesion size. Ann Sur 1998; 227: 559–565
- Lu DS, Raman SS, Vodopich DJ, Wang M, Sayre J, Lassman C. Effect of vessel size on creation of hepatic radiofrequency lesions in pigs: Assessment of the ‘heat sink' effect. AJR Am J Roentgenol 2002; 178: 47–51
- Liu Z, Ahmed M, Weinstein Y, Yi M, Mahajan RL, Goldberg SN. Characterization of the RF ablation-induced ‘oven effect': The importance of background tissue thermal conductivity on tissue heating. Int J Hyperthermia 2006; 22: 327–342
- Solazzo SA, Liu Z, Lobo SM, Ahmed M, Hines-Peralta AU, Lenkinski RE, Goldberg GN. Radiofrequency ablation: Importance of background tissue electrical conductivity—an agar phantom and computer modeling study. Radiology 2005; 236: 495–502
- Humphries S, Platt RC, Ryan TR. Etherm finite element modeling of electrical heating and non-linear thermal transport in biological media. Proc Amer Soc Mech Eng High Temp 1997; 355: 131–134
- Humphries S. Field solutions on computers, sections 11.3, 11.4, 12.2, 12.3. CRC Press, Boca Raton, FL 1997
- Lobo SM, Afzal KS, Ahmed M, Kruskal JB, Lenkinski RE, Goldberg SN. Radiofrequency ablation: Modeling the enhanced temperature response to adjuvant NaCl pretreatment. Radiology 2004; 230: 175–182
- Lobo SM, Liu ZJ, Yu NC, Humphries S, Ahmed M, Cosman ER, Lenkinski RE, Goldberg W, Goldberg SN. RF tumour ablation: Computer simulation and mathematical modelling of the effects of electrical and thermal conductivity. Int J Hyperthermia 2005; 21: 199–213
- Goldberg SN, Gazelle GS, Halpern EF, Rittman WJ, Mueller PR, Rosenthal DI. Radiofrequency tissue ablation: Importance of local temperature along the electrode tip exposure in determining lesion shape and size. Acad Radiol 1996; 3: 212–218
- Duck FA. Physical properties of tissue: A comprehensive reference book. Academic Press ; RCOURT Brace Jovanovich, Publishers, San Diego, CA 1990
- Goldberg SN, Charboneau JW, Dodd GD, III, et al. Image-guided tumor ablation: Proposal for standardization of terms and reporting criteria. Radiology 2003; 228: 335–345
- Gabriel C, Gabriel S. Compilation of the dielectric properties of body tissues at RF and microwave frequencies. Internet document, http://www.brooks.af.mil/AFRL/HED/hedr/reports/dielectric/home.html
- Aschoff AJ, Merkle EM, Wong V, Zhang Q, Mendez MM, Duerk JL, Lewin JS. How does alteration of hepatic blood flow affect liver perfusion and radiofrequency-induced thermal lesion size in rabbit liver?. J Magn Reson Imaging 2001; 13: 57–63
- Buscarini L, Buscarini E, Di Stasi M, Quaretti P, Zangrandi A. Percutaneous radiofrequency thermal ablation combined with transcatheter arterial embolization in the treatment of large hepatocellular carcinoma. Ultraschall Med 1999; 20: 47–53
- Horkan C, Ahmed M, Liu Z, Gazelle GS, Solazzo SA, Kruskal JB, Goldberg SN. Radiofrequency ablation: Effect of pharmacologic modulation of hepatic and renal blood flow on coagulation diameter in a VX2 tumor model. J Vasc Interv Radiol 2004; 15: 269–274
- de Baere T, Bessoud B, Dromain C, et al. Percutaneous radiofrequency ablation of hepatic tumors during temporary venous occlusion. AJR Am J Roentgenol 2002; 178: 53–59
- Hines-Peralta A, Sukhatme V, Regan M, Signoretti S, Liu ZJ, Goldberg SN. Improved tumor destruction with arsenic trioxide and radiofrequency ablation in three animal models. Radiology 2006; 240: 82–89
- Kariya Z, Yamakado K, Nakatuka A, Onoda M, Kobayasi S, Takeda K. Radiofrequency ablation with and without balloon occlusion of the renal artery: An experimental study in porcine kidneys. J Vasc Interv Radiol 2003; 14: 241–245
- De Bazelaire C, Rofsky NM, Duhamel G, Michaelson MD, George D, Alsop DC. Arterial spin labeling blood flow magnetic resonance imaging for the characterization of metastatic renal cell carcinoma(1). Acad Radiol 2005; 12: 347–357
- Kan Z, Phongkitkarun S, Kobayashi S, Tang Y, Ellis LM, Lee TY, Charnsangavej C. Functional CT for quantifying tumor perfusion in antiangiogenic therapy in a rat model. Radiology 2005; 237: 151–158
- Diederich CJ. Thermal ablation and high-temperature thermal therapy: Overview of technology and clinical implementation. Int J Hyperthermia 2005; 21: 745–753
- Patriciu A, Awad M, Solomon SB, Choti M, Mazilu D, Kavoussi L, Stoianovici D. Robotic assisted radio-frequency ablation of liver tumors—randomized patient study. Med Image Comput Comput Assist Interv Int Conf 2005; 8: 526–533
- Steinke K, Haghighi KS, Wulf S, Morris DL. Effect of vessel diameter on the creation of bovine lung radiofrequency lesions in vivo: Preliminary results. J Surg Res 2005; 124: 85–91
- Haemmerich D, Wright AW, Mahvi DM, Lee FT, Jr., Webster JG. Hepatic bipolar radiofrequency ablation creates coagulation zones close to blood vessels: A finite element study. Med Biol Eng Comput 2003; 41: 317–323