Abstract
Therapeutic ultrasound is currently enjoying increasingly widespread clinical use especially for the treatment of cancer of the prostate, liver, kidney, breast, pancreas and bone, as well as for the treatment of uterine fibroids. The optimum method of treatment delivery varies between anatomical sites, but in all cases monitoring of the treatment is crucial if extensive clinical acceptance is to be achieved. Monitoring not only provides the operating clinician with information relating to the effectiveness of treatment, but can also provide an early alert to the onset of adverse effects in normal tissue. This paper reviews invasive and non-invasive monitoring methods that have been applied to assess the extent of treatment during the delivery of therapeutic ultrasound in the laboratory and clinic (follow-up after treatment is not reviewed in detail). The monitoring of temperature and, importantly, the way in which this measurement can be used to estimate the delivered thermal dose, is dealt with as a separate special case. Already therapeutic ultrasound has reached a stage of development where it is possible to attempt real-time feedback during exposure in order to optimize each and every delivery of ultrasound energy. To date, data from MR imaging have shown better agreement with the size of regions of damage than those from diagnostic ultrasound, but novel ultrasonic techniques may redress this balance. Whilst MR currently offers the best method for non-invasive temperature measurement, the ultrasound techniques under development, which could potentially offer more rapid visualisation of results, are discussed.
Introduction
Therapeutic ultrasound can be delivered non-invasively using extracorporeal devices or minimally invasively using intracavitary or intraoperative devices. Therefore, the term ‘therapeutic ultrasound’ is probably more applicable than high-intensity focused ultrasound (HIFU or FUS). FUS is usually delivered under either magnetic resonance (MR) or diagnostic ultrasound imaging guidance. Ultrasound is cheaper to implement and more portable, but the development of MR monitoring and temperature measurement (thermometry) techniques is more advanced. Regardless of the method of FUS delivery, there is a need for treatment monitoring and, perhaps, for the accurate measurement of temperature. Thermometry requires quantification of temperature, preferably as a function of time, with known spatial and temporal resolution and confidence limits. Monitoring often relies on qualitative, and sometimes indirect, measures of effect, but measuring the extent of tissue damage (for example) may be more useful clinically than thermometry. Both MR and diagnostic ultrasound imaging are reviewed below.
The ability to measure temperature rise within the patient is important for all thermal treatments, particularly in the USA, where it is a regulatory pre-requisite to clinical use. Thermometry can be used to localise the ultrasound source relative to the treatment volume, avoid damage to surrounding tissues and predict the extent of damage (see thermal dose below). Whilst absolute temperature measurement might seem attractive, accurate quantification of temperature rise above baseline is more easily achievable and probably sufficient for current treatment monitoring and feedback control needs. The need for high-resolution temperature measurement for hyperthermia has been discussed by, for example, Hand Citation[1], Martin Citation[2] and Bruggmoser Citation[3]. For FUS, the required spatial resolution depends partially on the size of the focal region, which can be as small as 0.6 mm (full width half maximum pressure i.e, −6 dB beamwidth) for 3 MHz transrectal probes. Temporal resolution requirements depend on the exposure time (which can vary from less than 1 s to several minutes), the rate of temperature rise (which can exceed 30°C s−1) and how the temperature measurement is to be used. For feedback control during exposure a much higher temporal resolution will be necessary than for the post-exposure calculation of delivered thermal dose.
Thermal dose is used to predict the extent of tissue damage caused by a heating exposure. Definitions of thermal dose, arising from hyperthermia studies, have been reviewed by Kapp Citation[4]. Thermal dose is usually quantified using an ‘equivalent minutes’ calculation Citation[5] which was derived from in vitro cell survival experiments during the application of hyperthermia temperatures between 41°C and 50°C Citation[6]. A lethal dose was found to be 43°C for 240 min Citation[7]. Thus it is normal practice to convert the time, tT, at which tissue is held at a constant temperature T into the time that would have the equivalent effect at a temperature of 43°C, t43. This is often called the ‘equivalent time’ and is calculated using the relationship:where R = 0.5 above 43°C and 0.25 below 42°C. One widely used thermal dose term intended to account for a temperature that changes with time is ‘thermal dose cumulative equivalent minutes at 43°C to 90% of the target volume’ Citation[8].
This method is often used with MR thermometry to predict the size and shape of regions of FUS damage (called lesions) Citation[9–11], but it relies on the assumption that the relationship determined from hyperthermia treatments at 42–50°C is relevant to the much higher temperatures used in FUS which result in thermal coagulation and protein denaturation. Although not all FUS damage mechanisms are thermal, cavitation monitoring is not thoroughly reviewed here.
Monitoring
It is important that the term ‘treatment monitoring’ is clearly understood. We use it in the sense given by Goldberg et al. Citation[12], Citation[13] who defined it as ‘the process with which therapy effects are viewed during a procedure’. Treatment monitoring includes the assessment of both efficacy and safety (i.e. whether any adjacent normal structures are adversely affected). Goldberg et al. used ‘treatment assessment’ or ‘follow-up’ to describe the tissue response following treatment. We review mainly ultrasound and MR examinations carried out during and immediately after FUS since these imaging modalities have been used for clinical guidance.
Diagnostic ultrasound monitoring
Hyperechogenicity
Perhaps the simplest way to perform FUS treatment monitoring is to use diagnostic ultrasound. The appearance, in conventional B-mode images, of hyperechoic (bright) regions at the location of the focus, which can persist for periods from a few seconds to many minutes after an exposure, is well documented Citation[14–16]. Hyperechoic regions reported in porcine liver in vivo Citation[17] were thought to be caused by the formation of acoustically or thermally generated bubbles (cavitation), which scatter more incident ultrasound than soft tissue. In practice, a combination of bubble generation mechanisms is likely since heating will lower the cavitation threshold. Both active and passive cavitation detection, used during FUS exposure, have shown that cavitation activity was temporally well correlated with the appearance of hyperechoic areas Citation[18]. Tests using phantoms and ex vivo turkey breast tissue found similar results Citation[19]. Studies using overpressure, investigated as a means to change the onset of cavitation and to study its effects on lesion formatio Citation[20], Citation[21], found that bubble formation led to the production of ‘tadpole’-shaped, rather than ellipsoidal, lesions (). This is thought to be due to enhanced energy deposition caused by scattering from the bubbles. Bailey et al. Citation[20] found that B-mode monitoring was ineffective for FUS lesions in the absence of acoustic cavitation, presumably because the backscattering properties of normal and ablated tissue are similar, leading to low contrast in the absence of bubble formation.
Figure 1. Ultrasound backscatter image monitoring of a 3100 W cm−2 (free field spatial peak intensity) exposure of excised bovine liver imaged with a Z.One scanner (Zonare Medical Systems Inc., USA) using an 8-MHz linear probe. The FUS beam was incident from the left. Top images are B-scan images obtained with a frame rate of 14 Hz. Middle is a photograph of the lesion. Bottom are subtraction images: left is the result of subtracting the top left B scan from the top right; right is a similar result using a B scan image obtained 3.6 s later. The hyperechogenicity is due to bubbles, probably from boiling, and the reduction with time is due to cooling. Courtesy of Jim McLauglan, Institute of Cancer Research.
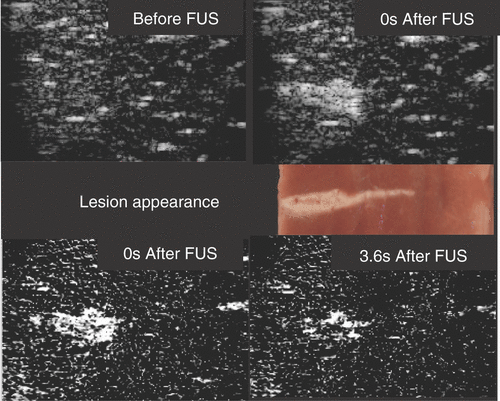
Conventional elastography
Clinicians routinely use palpation to detect tumours that are stiffer than their surrounding tissue. An ultrasonic method of visualising the elastic properties of tissue, called elastography Citation[22] or elastographic imaging, involves imaging tissue before and after compression by an externally applied force. The induced strain (fractional change in length) describes the extension/compression of a material depends on its stiffness (technically, its elastic or Young's modulus) and the applied stress (force per unit area). Elastograms, providing 2D maps of tissue stiffness, were originally developed for tumour diagnosis and are used in mammography. When elastography is performed using ultrasound imaging, the axis of the sound beam should be aligned parallel to the direction of the compression force. The tissue displacement is measured by tracking the displacement of features (speckle) in the ultrasound RF echo data. RF echo data lines can be divided into a series of reference data segments in the pre-compression data. These RF echo data segments are searched for in the post-compression data using a cross-correlation technique Citation[22]. This gives the displacement at each position in the image and, since the gradient of displacement along the direction of the force is proportional to the induced strain, strain images can be computed. The most common way to do this is using a least squares strain estimator Citation[23] which involves fitting a straight line to a number of displacement values or pixels. A more refined technique used multi-step compression Citation[24], allowing strain images to be computed from successive compressions and averaged to improve the signal-to-noise ratio. A range of image filtering and processing techniques can be applied. A free-hand method of applying the compression has been tested Citation[25], Citation[26] but using a motor-driven plate to ensure even compressio Citation[22], Citation[27] (e.g. using adapted mammography compressors) has been shown to give better results. Elastography has been used to visualise the prostate Citation[28] and intravascularly to image plaques Citation[29]. Thermally ablated tissue is typically stiffer than normal tissue, allowing elastography to visualise thermal ablation ex vivo Citation[23], Citation[30] and in vivo, where it has been compared with MR imaging Citation[31] after transrectal FUS treatment of the prostate. Here, inflation of the couplant-filled balloon surrounding the treatment probe provided tissue compression. This study was not particularly successful and concluded that elastography techniques required significant improvement. In other organs, such as the liver, tissue motion caused by cardiovascular and breathing motion leads to partial decorrelation at the RF echo tracking stage. However, ultrasound has been used to measure and quantify motion of the liver in 2D Citation[32]. Work with RF induced lesions in vivo has tried to use the natural compression provided by respiratory movement of the diaphragm Citation[33] and good correlation was observed between tissue pathology and the resulting elastograms.
Radiation force elastography and vibroa-coustography
A technique known as vibroacoustography Citation[34] uses the radiation force from a single focused transducer to cyclically stress a particular location in tissue by modulating the acoustic power at a frequency of a few kHz. The tissue's mechanical response was measured using a hydrophone that was sensitive in this frequency range. Radiation force elastographic imaging (RFEI) uses short millisecond bursts of high intensity ultrasound to push small regions of tissue Citation[35], with the resulting displacement quantified using correlation methods Citation[35], Citation[36]. A variation on the method is to use radiation force to generate shear waves in tissue that can then be monitored Citation[37]. These techniques have an advantage over conventional elastography since they generate strain locally and non-invasively without producing artefacts, which can occur during compression of large volumes of tissue. The strains measured using radiation force generated by a therapy transducer have been shown to be related to Young's modulus in excised liver tissue Citation[38] demonstrating the potential to use this technique for monitoring FUS. The contrast and signal-to-noise ratios in elastograms depend in part on the centre frequency and bandwidth of the imaging transducer, but are also related to the axial resolution which depends on the size of the reference data segment and least squares strain estimator used. This dependence has been modelled Citation[39] and demonstrated in practice Citation[40]. The typical spatial resolution of elastography was found to be slightly worse than 1 mm in the axial and lateral directions for a 5 MHz, 60% bandwidth probe with beamwidth of 1 mm Citation[41]. The lateral resolution is highly dependent on the frequency, bandwidth and transducer f-number Citation[42]. Although they are still some way from clinical use, elastographic techniques have the potential for monitoring and guiding FUS treatment with acceptable spatial resolution.
Acoustic tissue property measurement
Quantitative mapping of acoustic tissue properties such as backscatter, attenuation and B/A (non-linearity) coefficients could be useful for monitoring thermal ablation (speed of sound is discussed separately in the section Non-invasive ultrasound thermometry). In the absence of bubbles, changes in the backscatter properties of excised liver due to FUS exposure are too small compared with local variations to allow monitoring Citation[43]. Furthermore, acoustic tissue characterisation in vivo is far more challenging than measuring it ex vivo. Despite this, acoustic attenuation mapping has been used successfully in vivo to determine the pathological state of liver tissue Citation[44–48]. In degassed excised liver samples, FUS exposure was found approximately to double attenuation Citation[43]. Similar measurements have been made in vivo on the liver, spleen and the abdominal wall of pigs, and increased attenuation was observed following exposure Citation[49]. These measurements were performed using a transmission loss technique, which required an incision to insert the measurement devices into the abdomen. Less invasive measurements have been made in the prostate Citation[50], where changes in the apparent attenuation frequency dependence were found to correlate well with the FUS treated area. Non-invasive backscatter attenuation estimation (BAE) might be possible by implementing a substitution method using an axial beam translation approach where the relative path length of water and tissue between the imaging probe and measurement window is varied. An alternative is an inverse diffraction filtering process where the combined effect of acoustic diffraction and anytime dependent processing of the acquired RF data performed by the scanner, such as time gain compensation, is measured and applied as a filtering function to the depth dependent backscatter from a single fram Citation[51], Citation[52]. These techniques might be useful for characterising tissue attenuation prior to FUS as well as during treatment monitoring. Attempts to quantify the ultrasound non-linearity parameter (B/A) in tissue have been made using both pulse echo Citation[52] and tomographic Citation[53] techniques. The ability to image this parameter is useful because fatty tissues typically exhibit a higher value. However, there are no studies investigating its value for FUS treatment monitoring.
Magnetic resonance monitoring
Magnetic resonance (MR) imaging detects the net magnetic field of unpaired protons, usually of the hydrogen nucleus, 1H. While a detailed review of MR physics is beyond the scope of this paper, a summary of some fundamental concepts may be helpful. Proton spins naturally tend to align in one direction in a magnetic field. This spin direction can be ‘flipped’ (e.g. by 90 or 180°) by applying an external magnetic field. Once this RF perturbation ends, the spins lose their excess energy and relax back to their equilibrium state. The time taken to achieve relaxation via the two mechanisms, spin-lattice relaxation and spin-spin relaxation, are known as T1 and T2 respectively. The series of RF field pulses used to obtain data are known as the MR sequence. Several sequence types have been used with FUS. Spin echo (SE), or fast spin echo (FSE), sequences (a 90° followed by a 180° RF pulse) are usually used to obtain the information related to T2. A gradient echo (GRE), or fast spoiled gradient echo (FSPGR) sequence uses the MR field gradients to obtain data related to T1. Other sequences can be used to obtain different data. Images are usually dominated by the one signal component and therefore are classified as T1 or T2, weighted images, etc. A T2-weighted (T2-w) sequence requires a long echo time (TE) and long repetition time (TR). In a T1-weighted (T1-w) sequence, TR and TE are relatively short, allowing rapid image acquisition. Most of the studies reviewed below used 1.5 Tesla clinical MR scanners, and it is normal practice to use fat suppression sequences, which null out the signals from fat.
T2 weighted imaging
T2-w monitoring, using spin echo (SE) or fast spin echo (FSE) sequences, was identified early on as providing excellent contrast between intact and thermally damaged tissue in both excised and in vivo muscle in rabbiCitation[11], Citation[54], Citation[55] and greyhound Citation[56]. It remains the universally adopted ‘gold standard’ MR method of identifying and quantifying the extent of ultrasound lesions. Immediately after exposure, signal intensity increases with time until a hyperintense (bright) ring appears. Lesions in T2-w images of rabbit thigh muscle have been found to correlate well with the size predicted from thermal dose calculations made using MR thermometry Citation[57], Citation[58], post-mortem evaluation, and histological assessm Citation[59], Citation[60] using Haematoxylin and Eosin (H&E) staining. Similar lesions in the rabbit brai Citation[61], Citation[62] and synovial tissue Citation[63] also showed excellent correlation with size determined histologically using triphenyl tetrazolium chloride staining for cell viability, using both light and electron microscopy Citation[64]. T2-w imaging was also able to detect lesions in an in vivo sheep model of breast tissue Citation[65] and in human breast Citation[66]. Clinical trials also used T2-w imaging to guide the treatment, with the tumour appearing hyperintense relative to the surrounding tissue. One hour after treatment using a relatively low-intensity, long-duration, spiral track to deliver the ultrasound oedema was clearly visible as a hyperintense region in contrast enhanced T2-w (SE) images Citation[67]. Such monitoring has been shown to be robust even in the presence of ultrasound-generated bubbles Citation[68]. Studies in freshly excised pig kidney showed that a T2-w (FSE) sequence was best for detecting cavitation activity Citation[69], because it provided better contrast between air and necrotic tissue than T1, and that the best lesion to tissue contrast was obtained with TE between 16 and 32 ms. Contrast enhanced T2-w imaging (FSE) studies, using clinically licensed contrast agents containing gadolinium (Gd-DTPA) injected intravenously, showed that whilst the signal from normal tissue was enhanced, the signal from lesions was not Citation[59]. This has also been seen in the clinical treatment of breast tumours Citation[66], and is presumed to be due to vascular disruption. Ultrasound exposure after the intravenous injection of contrast agent was found to reduce greatly the rate of contrast washout relative to that seen in normal tissue, again suggesting vascular damage. The reported threshold for an irreversible lesion was 46°C for 30 min Citation[59], which suggested their low-power ‘siting’ shots caused no permanent damage.
T1 weighted imaging
Initially, T1-w FSE and FSPGR sequences were used predominantly for thermometry (see below), because T2-w images offered superior contrast between lesioned and undamaged tissue. Contrast enhanced T1-w imaging established itself as a standard monitoring tool. Studies showed that FUS-damaged rat liver, both with and without implanted tumours, became much less hyperintense than surrounding normal tissue Citation[70], after bolus injection of clinical contrast agent, suggesting reduced blood supply in ultrasound exposed regions. This was confirmed histologically. Contrast enhanced (FSE) T1-w images and subtraction images (where the image obtained prior to contrast injection is subtracted from that obtained afterwards Citation[58], Citation[70]) showed good correlation with pathological tissue damage, and histological lesion size assessment. This has also been observed in rabbit skeletal muscle, with and without tumours Citation[57], Citation[60], synovial tissue on the back of rabbit knees Citation[57], Citation[63], rabbit brain Citation[62], including that targeted through a human skull Citation[71], and human prostate Citation[31], Citation[72]. Where exposures were guided using MR thermometry, the observed regions of damage correlated well with those predicted using thermal dose. The clinical efficacy of T1-w monitoring was demonstrated in invasive breast cancer tissue, which was partially treated prior to surgery. Hypointense regions (indicating a lack of contrast uptake) revealed reduced perfusion in damaged regions of the tumours Citation[73], and histological assessment showed the hyperintense rim seen surrounding the damaged region corresponded to a region of hyperaemia (i.e. congested capillaries and haemorrhage) with reduced proliferative activity. In a study, which aimed to treat entire fibroadenomas prior to surgical resection, FSE imaging was used to assess efficacy immediately after treatment. Evidence of impaired vascular supply to the treated region Citation[66] correlated well with longer-term clinical results, including tumours shrinking and becoming softer. In excised porcine kidneys, optimum T1-w (FSE) imaging was obtained with a TR of 200–400 ms but this was judged to be too slow for clinical treatment monitoring. T1-w FSPGR sequence performance was optimum at flip angles of 75–90°, and a TR as low as 100 ms could be used without significantly reducing the SNR ratio or contrast. The FSGR acquisition time of 5 s was preferred for in vivo use in rabbit kidney Citation[69] to monitor the extent of the heated region Citation[74]. One important safety implication of T1 imaging, identified in this study was the poor contrast between necrotic tissue and air. Thus a layer of air, which appeared over one kidney and prevented lesioning could only be identified using T2-w imaging.
MR elastography
MR elastography (MRE) works on the same principles as ultrasound elastography described earlier. A small compression (typically less than 200 µm) of the tissue is required to be able to compare images before and after this tissue compression and deduce information relating to its elastic properties. In MRE, a phase-contrast MRI technique is used spatially to map and measure displacement patterns corresponding to shear waves with amplitudes of microns or less Citation[75]. Typical acquisition parameters for 2D sequences are TR of 10–300 ms and TE of 10–60 ms resulting in total acquisition times of 20–120 s. The ability to detect shear waves caused by a 5 ms focused ultrasound pulse has been demonstrated in both phantoms and excised muscle Citation[76]. The use of a short, non-damaging exposure offers an opportunity to locate the focal region accurately within tissue for treatment guidance, as well the potential for sizing tumours that are stiffer than the surrounding normal tissue. However, the reliance on a phase contrast MR sequence means that the results will only be indicative of shear wave amplitude if significant temperature rise is avoided. Thus MRE cannot monitor lesion formation but it could be used once the tissue has cooled. The same group went on to evaluate the ability to use MRE (with a mechanical actuator producing shear waves at 500 Hz) to determine lesion size in excised bovine muscle against T1-w, PD-w and T2-w imaging. The mechanical properties of ultrasound-damaged tissue were found to be significantly different to normal tissue. The authors suggested that MRE monitoring of stiffness may be a better indicator of lesion extent than using T2 to look at haemorrhage or using contrast enhanced T1 to look at oedema, but no comparison was attempted with optical or histological Citation[77–79] assessment of lesion size.
Stiffness weighted MRI Citation[80] has been investigated using a gradient echo MRE sequence with shear excitation at 100 Hz (200 µm displacement) or 400 Hz (50 µm displacement) produced using a mechanical actuator. Despite requiring a complex series of image-processing steps, the authors introduce a method of processing a time series of MRE magnitude images with phase dispersion to produce a stiffness-weighted image (SWI) by calculating the minimum signal at each pixel from a small number of temporal samples. Results in 1D simulated breast studies showed this qualitative technique was simple, fast and avoids the need for phase unwrapping for noise reduction. The biggest limitations were the need to avoid the significant depth-dependent wave attenuation, interference, and boundary effects for the shear waves.
Functional imaging (DCE-MRI)
Functional or dynamic contrast enhanced (DCE) MR imaging involves the intravenous injection of contrast agent and the acquisition of MR images as a function of time in order to look at the relative uptake and natural washout of contrast agent. One group has performed dynamic studies 20 min and 2 h after FUS exposure using T1-w (FSPGR) and T2-w (FSE) sequences Citation[81]. They calculated vessel permeability, K trans, and the extravascular, extracellular volume fraction (or leakage space) ve. The results showed that maps of these two functional parameters revealed regions of pathological change that were not visible with conventional MR monitoring. At short times after heating, ve underestimated lesion size, but only by (7 ± 2)% compared with (25 ± 6)% with T2. A region of extensive structural and vascular disruption was indicated only on Ktrans images, with a sharp decrease in Ktrans occurring at a temperature of 47.5 ± 0.5°C. In in vivo rabbit thigh muscle imaged 2 h post-FUS Citation[82], images of Ktrans and ve were found to be able to differentiate changes not visible in T2-w images, and were able to demarcate the border between viable and necrotic tissue identified from histological examination. The extent of damage was shown best by ve, while the inflammatory borders were shown most clearly on Ktrans.
MR angiography for vascular occlusion
Monitoring blood flow is important for assessing the effects of FUS on vasculature. Vascular damage was initially investigated as a potential treatment side effect Citation[83]. The efficacy of a phase contrast imaging sequence (flip angle 20°, TR 32 ms, TE 13.3 ms, slice thickness 1.5 mm and total acquisition time of 11 min) for producing 3D angiograms of rabbit femoral arteries was demonstrated. The therapeutic potential of vascular damage in foetal medicine has been examined by exposing rat femoral vessels to FUS Citation[84]. Successful monitoring was achieved using both an angiogram (FISP, fast imaging with steady-state precision) sequence (flip angle 15°, TR 50 ms, TE 14 ms, slice thickness 1 mm) and a contrast enhanced DESS (dual echo in the steady state) sequence (flip angle 70°, TR 44 ms, TE 9 ms) which combines FISP information with strong T2 weighting.
Other MR monitoring
Limited studies of proton density (PD) weighted imaging have shown that it does not add significantly to information available in T1 and T2 weighted images Citation[56], Citation[59], Citation[69], Citation[74]. Combined with gadolinium contrast agent injection, lesions in PD-w images appeared as bright rings surrounding a dark centre Citation[59]. Diffusion weighted imaging is sensitive to the diffusivity of water within a tissue. It has been investigated for treatment monitoring and follow-up after FUS treatment of uterine leiomyosarcomas (fibroids) Citation[85] by producing apparent diffusion coefficient (ADC) maps before and after treatment. A significant increase in signal intensity was found within treated areas, and the difference between mean pre-treatment ADC values (1504 ± 290 mm6/s2) and mean post-treatment values (1078 ± 293 s2 mm6) was statistically significant. Fluid attenuation inversion recovery (FLAIR) imaging showed better spatial agreement lesion extent than T1-w and identical results to T2-w monitoring of lesions in rabbit brain assessed by optical microscopy of H&E stained sections Citation[61]. Unfortunately, the longer TR of the FLAIR sequence will result in a longer acquisition time even if multi-slice interleaving, necessitated by the slice thickness of inversion pulse being greater than that of the imaging excitation, pulse can be avoided.
Invasive thermometry
Accurate thermometry during thermal therapy allows greater control of the treatment Citation[86]. If the Sapareto & Dewey formulation of thermal dose is relevant to FUS-induced cell death, then dose is exponentially dependent on temperature, and an increase of 1°C will double the dose for temperatures above 43°C. For a target tissue temperature of 57°C (20°C above normal human physiological temperature), this means that an uncertainty in measured temperature rise of only 5% (1°C) results in an uncertainty in the dose of a factor of 2. Dickinson Citation[78] states that an accuracy of about 0.1°C is needed to give an estimate of the biological damage for hyperthermia to better than 10% (this is discussed further in Discussion and conclusion). Thermometry in a therapeutic FUS field presents particular problems owing to the highly localised hotspot, very rapid temperature increase, and possible interactions between the field and the thermometry system. Such interactions include viscous heating and thermal conduction error, discussed below, as well as tissue disruption and acoustic effects such as scattering by the thermocouple and the potential introduction of cavitation nuclei along the track of the thermocouple. However, invasive thermometry is important for both laboratory measurements and the calibration of non-invasive techniques. The most common invasive technique is to use small wire thermocouples. Other devices such as fibre-optic probes and thermistors have been used, but acoustic absorption in the plastic that encases some fibres can cause significant artefacts reducing their potential usefulness despite their inherent MR compatibility Citation[87]. Thermistors have also had limited use owing to their relatively large size and non-linear temperature response Citation[88–90].
Fine-wire thermocouples
Thermocouples consist of two wires of different metals connected at one end to form a junction. The principle is generally attributed to Seebeck (1821), who demonstrated that an electromotive force (e.m.f.) is generated when such a junction is placed in an environment at a temperature that is different to that at the free ends. Fine-wire thermocouples are often the method of choice for temperature measurement in tissue Citation[77], Citation[78], Citation[87], Citation[91–93], and in particular have been used to calibrate less invasive techniques Citation[94]. They consist of two wires, generally between 12 and 50 µm in diameter (), soldered or spot-welded together to form a junction Citation[91] or multiple junctions Citation[87], Citation[95]. Apart from the practical difficulties of inserting the thin wires into tissue, which is usually achieved using a hypodermic needle, the use of metal thermocouples introduces two main problems. First, the thermal conductivity of metals is between 10 and 1000 times greater than soft tissue, leading to a ‘thermal conduction error’. This can be minimised by using small-diameter, low-thermal conductivity wiCitation[78], Citation[79], Citation[96]. Second, the density of metal is between 3 and 10 times greater than that of soft tissue (e.g. copper is 8900 kg m−3). Consequently, as the ultrasound wave passes, there is relative movement of the tissue and the wire. Friction from this movement results in ‘viscous heating’ in a thin region around the wire, and leads to an artificially high temperature measurement Citation[79], Citation[86], Citation[97–99].
Figure 2. This collage depicts three different types of thermocouple: (left) thin-film thermocouple (NPL, UK), (centre) soldered fine-wire thermocouple (ICR, UK), and (right) hypodermic needle thermocouples (Omega, USA).
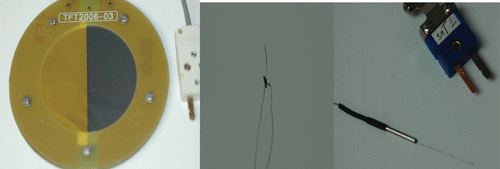
Fry and Fry Citation[79] explored FUS-induced viscous heating in castor oil and, according to their model, the initial phase is dominated by viscous heating (which they expected to reach 55% of its final value within 0.1 s, for a 13 µm diameter wire) and the second phase is dominated by absorptive heating. In the second phase, temperature increase due to viscous heating was assumed to be constant, thus the temperature at the end of the initial phase was simply subtracted in order to correct for the viscous heating effect. This approach has been followed widely Citation[94], Citation[99–101] even though the theoretical basis of the equilibration time in particular is not well justified in the original paper. Morris et al. Citation[93] proposed a refinement to this technique. Instead of assuming an arbitrary time for the end of the first phase, the time was chosen from analysis of the second differential of the temperature with respect to time. For experiments carried out in excised bovine liver with 50 µm manganin and constantan thermocouples (Goodfellows Ltd, UK) this time was 0.8 s, which is considerably longer than the times generally used (). Viscous heating was found to account for up to 70% of the measured temperature rise after 1 s. The error due to viscous heating was determined by applying a linear fit to the temperature rise vs. time curve at 0.8 s and extrapolating to zero time: this final step makes no allowance for a reduction in the rate of temperature increase due to thermal conduction, although the method could be adapted to include this effect.
Figure 3. Comparison of the temperature rise in excised bovine liver during a 150-W cm−2 (free field spatial peak intensity), 5-s exposure measured with a fine-wire thermocouple (FWT) and a thin-film thermocouple (TFT). The FWT suffers from viscous heating artefact whereas the TFT does not; therefore, the subtracted data (FWT-TFT) indicates viscous heating.
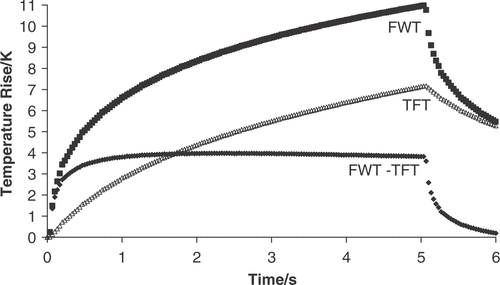
More recent studies Citation[102] have found that the equilibration times for a number of different thermocouples was between 1 s and 4 s. An alternative method of correction has been to measure the temperature rise at a fixed time after the end of an insonatio Citation[94], Citation[103]: this method assumes that the temperature increase due to viscous heating decays much faster than the real temperature rise. Since the solution to the temperature decay curve is essentially the negative of the heating curve, the absorptive heating also decays following the end of exposure. Temperature should be measured as a function of time to allow correction for this decay. Other approaches to evaluating viscous heating have been proposed. Huang et al. Citation[98] carried out thermometry experiments using needle thermocouples (type E, bare junction, 125 µm diameter, Omega Engineering Inc., Stanford, CT) embedded in two different agar-based phantom materials: one was graphite loaded to increase absorption, the other was not. Temperature rise in the non-absorbing material was assumed to arise from viscous heating only. For a −6 dB beam diameter of 2.5 mm over 1 s, the measured temperature rise was 1.9°C, of which 0.65°C was attributed to viscous effects.
Morris et al. Citation[93], Citation[102] have also used thin-film thermocouples as a viscous-free reference, and calculated the viscous effect for a wire thermocouple as a function of time by subtracting the TFT measurement from the wire measurement (). For the exposure condition tested, the percentage contribution of viscous heating (25–60%) to the total temperature rise was almost constant up to 1 mm away from the spatial peak of a 1.8-mm −6 dB diameter FUS field.
A second important artefact of using wire thermocouples is thermal conduction error. Dickinson Citation[78] found that this leads to averaging of the temperature over a region much larger than the junction size. The radius of the point-spread function of a copper-constantan junction of 50 µm diameter wire was measured to be between 1 and 2 mm, but it was only 0.4–0.6 mm for lower thermal conductivity manganin-constantan wires. For some ultrasound fields, even the smaller figure represents a rather large point-spread function, which could have a siginificant effect on tempertaure measurments. Although not reported in the literature, it is worth speculating that there may be acoustic interference effects when using larger needle-type thermocouples. Since acoustic scattering from bubbles is detectable, it seems likely that there may be a significant coherent scattered wave returning to the transducer from a needle thermocouple placed near the focus.
Thin-film thermocouples
The metals making up the junction of a thin-film thermocouple (TFT) are deposited in a thin layer directly onto a substrate. Often they are constructed from the pure metallic element rather than standard thermocouple alloys to avoid variations in the composition of the alloy during vaporisation. They have been used in number of areas where it is necessary to have extremely good thermal contact with a substrate or where minimum disturbance to the measurement environment is essential, for example turbine blades or microprocessors. In general, the TFT is formed on a solid substrate, but a version has been developed for use in ultrasound fields Citation[104]. It consists of 0.2-µm-thick electrodes deposited on a 12-µm-thick mylar film supported by a circular ring of diameter 85 mm; the element size was 0.2 mm square (). TFTs have been shown to avoid viscous heating artefacts during FUS exposure Citation[93] since the film prevents relative movement of the thermocouple junction and the medium, and reduce conduction errors since the thin cross-section ensures that the electrodes are in thermal equilibrium with the medium. They are also robust and have very low noise levels. TFTs have been used in thermal test objects for diagnostic ultrasound Citation[105], Citation[106]. For ex vivo work where tissue dissection is acceptable, TFTs are generally better than fine-wire thermocouples, but in vivo thermometry is currently precluded by their size and shape.
A potential problem with using a TFT is the generation of standing waves caused by specular partial reflection between the TFT membrane and the transducer. The pressure reflection coefficient of the membrane is less than 2% at 1 MHz and 5% at 3 MHz, so the reflected energy is very small. However, some resonant transducers can be highly sensitive to reflections, which change the acoustic impedance seen by the transducer and therefore modify its output power. The authors have found that standing waves can be reduced or eliminated by tilting the TFT by between 5° to 10° so it is not exactly perpendicular to the beam-axis.
Thermochromic liquid crystals
Cholesteric liquid crystals change colour within the temperature band for which they have prepared. This may be as narrow as 0.5°C or as wide as 50°C. Only a thin (∼25 µm) layer of crystals is needed to indicate temperatures visually on a surface. They have a high spatial resolution of up to 20 lines mm−l and a high speed of response of less than 0.2 s. The colour change is very non-linear with respect to temperature but accuracy of between 0.15°C and 0.5°C for a crystal with a 5°C temperature band has been claimed Citation[107]. There are many industrial applications of the thermochromic liquid crystals (TLCs). However, in FUS, they are potentially useful for external surfaces (e.g. the skin) or as a quality assurance tool for visualisation of the ultrasound field distribution. The use of the liquid crystals has been advocated to map the distribution of intensity Citation[108], and the technique has been developed further for the visualisation of physiotherapy fields Citation[109]. They have been used as a tool for localisation/visualisation of a FUS field in degassed water Citation[110], and showed good qualitative correlation between colour maps, hydrophone scans, theoretical modelling and fine-wire thermometry to demonstrate the potential for ribs sparing in FUS treatments. However, since the heat loss mechanism on a surface is primarily convective and therefore not linear with temperature, the indicated temperature rise is not simply related to either intensity or to the temperature inside a tissue region.
Non-invasive MR thermometry
MR thermometry techniques have been reviewed in depth previously Citation[111], this paper does not review any potentially rapid MR techniques for which FUS applications have yet to be published such as echo planar imaging and the use of specialised data reconstruction techniques.
The main parameters that have been used with FUS are: spin-lattice relaxation time (T1), proton resonance frequency (PRF) and proton diffusion (D). Proton diffusion thermometry is potentially the most sensitive and accurate MR technique. Also, all soft tissues except fat have the same mass diffusivity coefficient. Its main disadvantage is the high susceptibility to movement. Sensitivity of around 2.4%/°C can be achieved using GRASS (gradient echo acquired in the steady state), FLASH (fast low-flip-angle shot gradient-recalled echo) and CPMG (Carr Purcell Meiboom Gill) sequences Citation[111]. In order to use the diffusion parameter for GRASS, the T2 of the material must be long, since short T2 (such as in liver) produces low diffusivity. It is important to note that while diffusion effects are dominated by temperature change, they are not necessarily independent of other factors such as pressure, pH, and paramagnetic content. Thus, D-weighted thermometry has not been widely used in-vivo with FUS. T1 and PRF thermometry are reviewed in detail below.
Spin lattice relaxation time (T1)
T1 weighted (T1-w) sequences (such as SE and GRE) are generally insensitive to motion but highly sensitivity to temperature (with T1 relaxation time decreasing by 1–2%/°C) and to the presence of paramagnetic substances and free radicals. Compared with other thermometry sequences, T1-w imaging generally requires a relatively long acquisition time and is therefore not ideal for real-time thermometry. Furthermore, T1 changes (see monitoring section) due to the onset of protein denaturation limit the useful range of temperature measurement to <50°C and, once the temperature exceeds 40°C, hysteresis Citation[112] is a significant problem. MR thermometry for use with FUS was first published in the early 1990s Citation[11], Citation[54], Citation[56], Citation[113], Citation[114] for gel phantoms, excised muscle and in vivo rabbit muscle. The rate of change of T1 with temperature was verified in water-heated muscle by comparison with measurements made using a bare 125-µm diameter copper-constantan thermocouple Citation[11]. A reversible dark (hypointense) region was observed in muscle until denaturation occurred (at about 60°C), at which point an irreversible change was seen on the MR image, and in the specimen. Comparison with diffusion weighted imaging determined the effective thermal diffusion coefficient to be 0.0033 cm2/s in excised bovine and in vivo rabbit muscle Citation[54]. By extrapolation of the thermal dose (discussed above), they suggested that 57°C for 1 s should be sufficient for cell death. In these early studies, in plane resolution of 1 mm and slice thickness of 5 mm was typical. This meant that the FUS focus could be resolved fairly well across the axis, but there was significant averaging along the axis. Similar results were demonstrated for in vivo rabbit kidney Citation[115]. Despite this success, the main early limitation of MR thermometry was the lack of real-time information, which could only be obtained by selective reduction of the data that were assessed Citation[116]. Although using an FSE sequence with a TR of 100 ms outperformed similar T1 sequences in terms of thermal and temporal resolutions Citation[117], practical real-time thermometry remained elusive. More recently, MR thermometry, with temperature resolution better than 1°C, in which one image slice was acquired in just 3 s, was demonstrated in porcine tissue (including fatty tissues) using a fast T1-w sequence Citation[118]. In a study demonstrating the use of ‘real-time’ MR thermometry for feedback-controlled thermal therapy delivered by a stationary source, successful treatment delivery was demonstrated without violating safety constraints of maximum temperature permissible in normal tissue. Post-exposure T2-w images of canine muscle showed good spatial correlation between the exposed area and thermally damaged tissue Citation[119].
Proton resonant frequency
Proton resonant frequency (PRF) shift, also known as water proton chemical shift (CS) imaging, is based on the principle that, as temperature changes, there will be a phase difference in the resonance peaks owing to changes in the strength and lifetime of the hydrogen bonding with temperature. In water, the PRF has been found to be a decrease of 10 ppb/°C Citation[119]. A highly homogeneous Bo field is required to detect this level of change, but in general these measurements can be made to high accuracy. In practice, PRF thermometry, using gradient echo (GRE) or proton density (PD) sequences, requires a reference (baseline) image be taken at a known starting temperature. This reference image is then subtracted from subsequent images obtained at different temperatures. It may also be necessary to reduce noise by phase unwrapping Citation[111]. Since PRF shift is affected by other factors such as pH, ionic strength of the medium and the concentration of the dissolved substances, accurate quantification of temperature is only possible if these parameters are invariant during the exposure: this is assumed to be the case. The biggest disadvantage of PRF thermometry is its susceptibility to motion artefacts. In the late 1990s, there was a shift away from T1-w thermometry to PRF thermometry. The PRF coefficient was measured in rabbit skeletal muscle in vivo, using an SPGR sequence, as −8.76 ± 0.69 ppb/°C Citation[120]. Temperature uncertainty was ±4°C, spatial resolution 2.3 mm, and images were obtained every 3.3 s. In a similar study, using FSPGR sequence with a 3 mm image slice, 0.6 mm in plane resolution and a scan time of 3.4 s, PRF shift was ‘calibrated’ using two ultrasound exposed rabbit thigh muscles in vivo Citation[60] to be 9.09 ± 0.68 ppb/°C. The Brigham and Women's Hospital group also measured the coefficient for in vivo rabbit brain as 10.7 ± 0.9 ppb/°C Citation[62] using the FSPGR sequence. The reported values agree reasonably well with the value for water and with other tissues (which are thought to be relatively invariant Citation[121]). In all these cases, the PRF coefficient was obtained by comparison with temperature measurements made with twisted pair 50-µm diameter bare wire copper-constantan thermocouples. In the muscle study, the thermocouple viscous heating artefacts were avoided by measuring the temperature 2 s after exposure; and in the brain study the effect was corrected for using the methods of Fry and Fry Citation[79] discussed above. Correction using the Frys’ method can lead to overestimating the temperature because of this artefact by as much as 50% Citation[102]. Thermal dose has been shown to be a more reliable predictor of lesion formation than applied acoustic power Citation[62], Citation[122]. PRF thermometry has also been shown to be effective for monitoring lower temperature rises for treatment guidance Citation[123]. As MR imaging technology improved it became possible to obtain PRF thermometry with a temperature resolution of about 0.4°C and temporal resolution of about 0.44 s Citation[124] in excised tissue and in in vivo rat muscle. High spatial resolution (0.63 mm) prediction of damage via PRF obtained thermal dose has also been demonstrated using PRF imaging with a 1-mm slice thickness during 10 s FUS exposures: the suggested threshold (measured at the lesion periphery) for thermal coagulation of excised bovine tissue (which is often used in order to minimise the number of in vivo experiments that are needed) was 54°C for 10s Citation[10]. Preliminary in vivo rabbit muscle experiments confirmed the estimation of necrosis from thermal dose considerations Citation[125]. Temperatures above 50.4°C or thermal dose more than 31.2 equivalent minutes at 43°C produced damage at all locations, while none occurred when the values were less than 47.2°C or 4.3 equivalent minutes. Similarly, for in vivo rabbit brain Citation[126] no lesions were detected using either MR or histology at levels lower than 7.8 min equivalent at 43°C or peak temperatures lower than 46.8°C. The consistency of PRF thermometry both as a function of time (over 6 months) and between 15 different phantoms heated by thermal diffusion from a water bath has been studied in detail Citation[127] and found to be consistent with an average value of 9 ± 1 ppb/°C. It is worth noting that this is an uncertainty of 11%, which, because of the exponential dependence of dose on temperature, equates to an uncertainty in thermal dose of nearly a factor of 8. Furthermore, it seems likely that the uncertainty between the MR properties of tumours in patients would be more than that observed between carefully manufactured gels.
PRF thermometry has been used to determine the thermal conductivity of tissue exposed to focused ultrasound Citation[128] by monitoring the rate of expansion of the (assumed) Gaussian ultrasound beam profile during heating. Values within 10% of the true value were obtained by fitting modelled data to the measured data. Ultrasound absorption coefficients were also obtained from the rate of temperature rise assessed using PRF thermometry obtained from an SPGR sequence Citation[129] in non-biological tissue and the technique should work in tissue.
Aside from the common MR susceptibility to motion artefacts, an important limitation of the technique is the requirement for aggressive suppression of the signals from fat. This has been demonstrated to be problematic when the target tumour is in breast tissue, with temperature monitoring failure rates as high as 60–80% Citation[130]. Tissue swelling during protracted treatments can be a problem if longer than 10-s exposure times are used. Sufficient swelling to cause severe artefacts in 2D temperature maps were shown to occur around 1 min after exposures Citation[131]. However, PRF thermometry was found to be robust with significant gas bubble formation within the lesioned tissu Citation[68], Citation[132].
Simultaneous T1 and PRF thermometry have been demonstrated using a dual echo GRE sequence, with validation using the temperature increase occurring in a polyacrylamide gel in its exothermic setting phase Citation[133]. The PRF coefficient for the gel was measured as 9.98 ± 0.24 ppb/°C and the T1 coefficient was 102.3 ± 2.9 ms/°C. Spatial resolution was 1.2 mm, temperature resolution was 2°C and time resolution was 1.4 s per image slice, although the sequence took a total of 34 s to run and thermometry was achieved offline. Another paper compared T1 relaxation time, diffusion coefficient and PRF thermometry Citation[132]. The sensitivity of each technique was reported as 1.98%/°C for T1, 2.22%/°C for diffusion coefficient, and; 10.1 ppb/°C for PRF. Temperature resolutions measured from the inverse correlation of the data over the whole calibration range were: 2.1 ± 0.6°C for T1 0.93 ± 0.2°C for diffusion, and 1.4 ± 0.3°C for PRF. T1 methods had the advantage of being applicable in fatty tissue and the PRF method was preferred to the diffusion method, which was restricted by the requirement of a long echo time and by anisotropic diffusion in tissue.
Temperature-controlled thermal ablation using MR thermometry for feedback was demonstrated in vivo in rats, using a Bruker 4.7 T animal imaging system, with long (30 min) exposures designed to produce slow (5–30°C) temperature rises rather than rapid ablatio Citation[134], Citation[135]. Later, online temperature correction, again using MR thermometry, was demonstrated Citation[136] in studies in which a PRF sensitivity of 9.4 ppb/°C was assumed. This group went on to demonstrate the production of a spiral of damage requiring a 1000-s exposure duration under PRF thermometry guidance using a clinical sc Citation[137], Citation[138]. The damage was targeted at VX2 tumours implanted in rabbit thigh muscle and complete tumour ablation was achieved in five out of six animals 5 weeks after exposure. Ultimately, they were also able to demonstrate fast 3D thermometry Citation[139]. To date, PRF thermometry guided focused ultrasound has been developed for the clinical treatment of uterine leiomyosarcomas (fibroids) Citation[140], Citation[141], brain tissue Citation[71] and breast tumours Citation[65], Citation[66]. An example of such use is shown in . It also has potential in applications that do not require ablation: for instance, enhancement of drug or gene therapy has been suggested Citation[142].
Figure 4. MRI-based temperature mapping during focused ultrasound thermal ablation of a uterine fibroid using the Exablate 2000 device (InSightec, Haifa, Israel). (a)–(b) Temperature maps acquired at peak temperature rise during two 20-s exposures. Contours show regions that reached thermal dose values of at least 18 and 240 equivalent minutes at 43°C. Based on previous in vivo work, the edge of thermally induced lesions should lie between these contours, yielding a lesion that is approximately 0.8 cm wide and 2.8 cm long. In practice, the ablated volume within the fibroid is often increased, presumably due to vascular occlusion. (a) Coronal imaging in the focal plane. (b) Sagittal imaging along ultrasound beam direction. (c) Spatial temperature distribution at peak temperature rise. (d) Temperature rise vs. time at the focus. (Figure courtesy of Nathan McDannold, PhD, Harvard Medical School, Brigham & Women's Hospital Department of Radiology, Boston, MA, USA.)
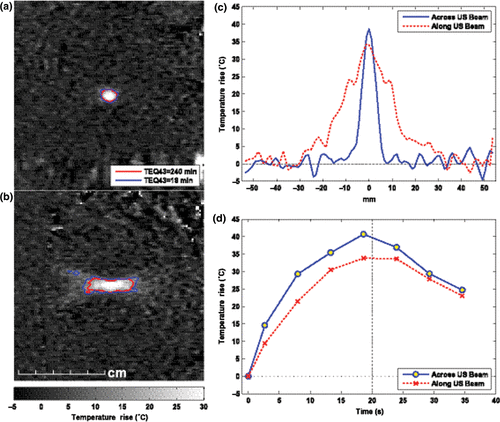
Non-invasive ultrasound thermometry
Probably the most successful use of diagnostic ultrasound to monitor temperature rise for FUS was suggested by Seip et al. Citation[143]. This technique relies on the fact that the speed of sound in non-fatty tissue increases with temperature between 37°C and 50°C Citation[144]. Thus, an ultrasound pulse propagating from an imaging transducer through a heated region of tissue and scattered back through the same acoustic pathway will arrive at the transducer in a shorter time than it would through an unheated region. The displacements of segments of backscattered RF lines between pre- and post-heated images can be determined using cross-correlation techniques similar to those employed in elastography, described above. In thermometry, the rate of change of displacement caused by localised heating produces apparent strain images.
Ultrasound scanners usually assume a fixed speed of sound (cs) to convert the time delay of a reflected echo into a position. For a small length of tissue (δx) in the axial direction of the imaging probe, where the sound speed (c) is a function of temperature (T), we can define the change in time of arrival from a reflected pulse δt due to a difference in temperature between T1 and T2:The change in time of the reflected echo will be converted to an apparent change in position or displacement δy given by:
Since strain, ε, is defined as a relative change in size, the apparent strain induced by a change in sound speed can be defined as the apparent displacement per unit length. In the limit where δx becomes infinitesimally small the strain is given by:
Inducing relatively small temperature rises and therefore changes in the speed of sound allows the definition of a quantity δc that is the difference between the speed of sound at temperatures T1 and T2:
where
if the above approximation is valid, the apparent strain can be estimated as:
It follows, therefore, that over a small temperature range, the apparent strain induced by heating tissue will be linearly related to the temperature rise, provided there is a linear relationship between speed of sound and temperature.
The apparent strain value can be related to the local temperature change if the relationship between speed of sound and temperature is known. Preliminary experiments Citation[143] showed the technique worked both in a rubber phantom and in excised liver tissue for temperature rises of a few degrees. Other researchers have investigated the feasibility of using this technique in hyperthermia by considering how the accuracy of temperature rise measurements depends on assumptions made about the variation of both the speed of sound and tissue expansion with temperature Citation[145–148]. It seems unlikely that accurate temperature measurements in the focal region can be made for FUS as a result of the non-linear dependence of sound speed on temperature over such a large temperature range and of the irreversible changes caused by ablation. However, it has potential applications at sub-lesioning levels to visualise the FUS Citation[149], where a fundamental limitation of the technique was identified as the variation in temperature dependence of the speed of sound between tissues with differing fat content. Subsequent experiments in excised bovine liver Citation[150] concentrated on determining the maximum apparent strain value that could be expected for FUS guidance, based on a starting temperature of 37°C (body temperature) and given that the speed of sound reaches a maximum in human liver at around 50°C, decreasing thereafter Citation[144]. With an imaging transducer mounted adjacent to the tissue, close to the focal plane, quantitative measurements of the apparent strain were obtained in images obtained both across and along the FUS beam. In extracorporeal and transrectal devices, a co-axial arrangement is more likely allowing imaging along the acoustic axis, but the imaging transducer will be further from the region of interest than in the laboratory studies. This alignment will also maximise the thermal lensing effect Citation[151] in which the sharp temperature gradient across the focal region refracts the diagnostic beam leading to diminished correlation between pre- and post-heating RF echo segments. This results in noisier apparent strain/temperature rise estimates at and beyond the focus. This effect was observed in excised liver Citation[151], where it was found that the optimal angle between the therapy and imaging beam axis is 90°. In the co-axial arrangement, a reduction in the strain signal-to-noise ratio in the focal region was found to be significant compared with the perpendicular arrangement, making it difficult to define the posterior edge of the heated region. However, this arrangement was useful for visualising the location of the focal region. As for strain images, noise can be reduced by computing apparent strain from several post-heated sets of images, using 2D tracking windows [152] or by implementing image filtering techniques. The spatial and temperature resolution achievable using diagnostic ultrasound will depend in part on the scanner, and in particular, on the frequency and bandwidth of the imaging probe used. There is also a trade-off between spatial resolution and the signal-to-noise ratio. Generally the technique can detect temperature rises of the order of one degree or less Citation[150].
Discussion and conclusion
Addressing first thermometry, there is a clear need for invasive measurements to validate the non-invasive techniques discussed in this paper under conditions of small beamwidth and rapidly changing temperature. Invasive temperature sensors provide potentially higher temporal, spatial and temperature resolution, but may interfere with FUS fields and change local thermal conduction. Fine-wire thermocouples require careful consideration of viscous heating and thermal conduction artefacts. Thin film devices that largely overcome these problems cannot currently be implanted in vivo but they should be used as reference in ex vivo tissue. The development of an implantable thin film thermocouple would be extremely valuable, as invasive temperature measurement has not changed significantly since the first experiments by Fry and Fry in the 1950s.
MR thermometry yields moderately high spatial resolution temperature mapping (approximately 0.5 mm in plane, and 1 mm slice thickness), using a number of temperature sensitive parameters. Proton diffusion provides the best temperature sensitivity, while PRF thermometry is most widely used because it provides good temperature resolution (about 2°C in vivo) and contrast to noise in soft tissues apart from fatty regions such as breast, where T1 thermometry may have to be used; T1 is also less susceptible to motion artefacts. PRF thermometry is particularly effective for FUS exposure times more than twice the scan time (usually between 3 s and 5 s), hence MR-guided FUS often uses longer exposures than US-guided FUS: longer total treatment time would be considered a disadvantage. New 3 T MR scanners could yield significant improvements, including faster data acquisition and evidence as to whether the PRF coefficient is truly invariant between tissue types. Although real-time FUS thermometry might be easier to achieve with the higher frame rates of ultrasound (typically 30 frames per second), a significant obstacle to achieving this is that the temperature–speed of sound relationship in different tissues is not well characterised, resulting in qualitative rather than absolute temperature imaging, although the thermal resolution of the apparent strain images is relatively high corresponding to a temperature resolution of around 1°C at body and room temperatures. Furthermore, ultrasound thermometry is susceptible to degradation by motion, thus requiring robust motion compensation processes in organs such as the liver.
Treatment monitoring must include consideration of treatment safety as well as efficacy. There are two aspects to treatment efficacy. The first is to know, preferably in real-time, the extent of damage after each FUS exposure in order to proceed or plan the next. The second is to know the extent of damage at the end of all the exposures to ensure complete treatment: in this case real-time assessment is not required, and several seconds or even minutes of analysis may be adequate. In both cases, it is important that the imaging information correlates closely with the extent of damage that would be found in post-mortem or histological assessment. MR monitoring is undoubtedly more advanced than diagnostic ultrasound monitoring. This is due, in part at least, to the FDA requirement in the USA that temperature is measured during thermal therapy. This may also explain the preference for, and investment in, MR image guidance on that continent. By comparison, the development of quantitative ultrasound monitoring has been much slower, with only detection of hyperechogenicity used clinically. The current gold standard for MR monitoring during delivery is to use thermal dose calculated from PRF thermometry to predict the extent of damage. However, care is required in interpreting the thermal dose: any uncertainty in the temperature (whether due to artefacts, drift, spatial or temporal-averaging, or to the fundamental sensitivity of the change in the MR signal to temperature) is greatly magnified when converting to a dose quantity. The appropriate form of the temperature–dose relationship is also open to question. In order to achieve the ideal state of the whole tumour just exceeding a lethal dose whilst allowing peripheral tissue to approach closely to this threshold so that treatment times can be minimised and complex treatment volumes defined to allow tissue-sparing of important structures, much better validation and understanding of the uncertainties is required.
Diagnostic ultrasound, unlike MR, has to be interleaved with FUS to avoid artefacts in the image. While this does diminish the real-time capabilities of ultrasound, it is not difficult to design systems that incorporate this. The variation and non-linear dependence of the speed of sound on temperature in tissue means that ultrasound cannot currently provide accurate absolute temperature values. As a result, clinical FUS monitoring so far relies on observing hyperechoic backscatter due to bubble formation, a surrogate marker of damage which is known to correlate poorly with lesion extent. Ultrasound elastography, and therefore temperature imaging based on the same analysis, is reported to have spatial resolution at least as good as convention B-mode imaging [153]. Therefore, for rapid imaging, ultrasound at 5 MHz and above should have spatial resolution at least comparable to 1.5 T MR, which may be an important advantage in highly focused FUS fields, but only if ultrasound thermometry can be implemented successfully.
T2-w and contrast enhanced T1-w imaging is currently the gold standard MR method for monitoring the final extent of FUS damage, with excellent validation in numerous tissues when compared with gross tissue damage and histology. Of the novel MR techniques investigated, functional imaging, to produce maps of Ktrans and ve, appear to outperform the gold standard when both were compared with histological assessment. However these techniques required additional complex data analysis compared with the ease of standard MR monitoring, and have yet to be independently validated. MR (and ultrasound) elastography imaging, either using tissue compression or the propagation of lateral shear waves, has the advantage of imaging structural changes associated with thermal damage. The neatest solution to the requirement for an external force is to use radiation force from an FUS transducer at a non-damaging exposure level. Although the data analysis is more complex than standard MR monitoring, there appear to be few significant technical obstacles to clinical implementation. The most promising ultrasound monitoring techniques are elastography and attenuation coefficient mapping, but both remain unproven. In principle, ultrasound contrast agents could also be injected immediately after treatment to demonstrate vascular disruption in an analogue to T1-w contrast enhanced MR.
In terms of treatment safety, ultrasound may have advantages over MR since it is inherently more sensitive to bubble formation. Bubble formation at the skin or in overlying tissues will reduce treatment efficacy and greatly increase the risk of side effects such as skin burns. Despite the apparent merits of MR monitoring, the only publication to date related to serious skin burns arising from FUS was as a result of MR-guided treatment [154]. This provides a stark warning to avoid complacency.
In conclusion, it is extremely important to include safety as well as efficacy in monitoring criteria. MR has been demonstrated to monitor tissue change successfully. MRE, T2-w imaging and ve (extravascular, extracellular volume fraction or leakage space) correlate well with histological assessment of damage; the latter potentially producing the best results. Typical spatial resolution for slice thicknesses is approaching 1 mm and in-plane resolution slightly better than this. Ultrasound backscatter imaging does not visualise thermal damage, only the generation of bubbles, which may already indicate that the tissue has been exposed to much higher temperatures or thermal dose than are required for cell death. Ultrasound elastography, especially that obtained from radiation force imaging, and attenuation mapping have the potential to monitor damage but require significant development. MR PRF thermometry, and thermal dose prediction correlate well with tissue damage, in most tissues, the main limitation being in tissues with a high fat content. Further research is required to see if the tissue invariance of the PRF coefficient is real or due to a lack of sufficiently sensitive measurements. Further research is also required to validate the calculated temperature and dose quantities against accurate contact invasive measurements: critical aspects of this will be to validate under conditions of small beamwidth and rapidly changing temperature, and the use of thin-film thermocouples to avoid viscous heating and conduction artefacts. Ultrasound thermometry is currently at least a decade behind MR, and the number of teams developing such techniques is very small. Hopefully, this will change as an increasing number of commercial ultrasound-guided devices come to market.
Acknowledgements
The authors are supported by EPSRC grant no. GR/SR64042, the National Measurement System Directorate of the Department of Trade and Industry, and the Institute of Cancer Research.
References
- Hand JW. Heat delivery and thermometry in clinical hyperthermia. Recent Results Cancer Res 1987; 104: 1–23
- Martin CJ. Temperature measurement in tissues by invasive and non-invasive techniques. Hyperthermia, DJ, Watmough, WM Ross. Blackie & Sons Ltd, London 1986; 154–179
- Bruggmoser G. Locoregional high-frequency hyperthermia and temperature measurement. Springer-Verlag, Berlin 1986
- Kapp DS. Thermal dose response, systemic hyperthermia, and metastases: Old friends revisited. Int J Radiat Oncol Biol Phys 1996; 35: 189–194
- Sapareto DG, Dewey WC. Thermal dose determination in cancer therapy. Br J Radiat Oncol Biol Phys Med 1984; 10: 787–800
- Dewhirst MW, Viglianti BL, Lora-Michiels M, Hanson M, Hoopes PJ. Basic principles of thermal dosimetry and thermal thresholds for tissue damage from hypertherm. Int J Hypertherm 2003; 19: 267–294
- Damianou CA, Hynynen K. The effect of various physical parameters on the size and shape of necrosed tissue volume during ultrasound surgery. J Acoust Soc Am 1994; 95: 1641–1649
- Arora D, Cooley D, Perry T, Skliar M, Roemer RB. Direct thermal dose control of constrained focused ultrasound treatments: Phantom and in vivo evaluation. Phys Med Biol 2005; 50: 1919–1935
- Chung AH, Hynynen K, Colucci V, Oshio K, Cline HE, Jolesz FA. Optimization of spoiled gradient-echo phase imaging for in-vivo localization of a focused ultrasound beam. Magn Reson Med 1996; 36: 745–752
- Graham SJ, Chen L, Leitch M, Peters RD, Bronskill MJ, Foster FS, Henkelman RM, Plewes DB. Quantifying tissue damage due to focused ultrasound heating observed by MRI. Magn Reson Med 1999; 41: 321–328
- Cline HE, Schenck JF, Hynynen K, Watkins RD, Souza SP, Jolesz FA. MR-guided focused ultrasound surgery. J Comput Assist Tomogr 1992; 16: 956–965
- Goldberg SN, Grassi CJ, Cardella JF, Charboneau JW, Dodd GD, III, Dupuy DE, Gervais D, Gillams AR, Kane RA, Lee FT, Jr, et al. Image-guided tumor ablation: Standardization of terminology and reporting criteria. J Vasc Interv Radiol 2005; 16: 765–778
- Goldberg SN, Grassi CJ, Cardella JF, Charboneau JW, Dodd GD, III, Dupuy DE, Gervais D, Gillams AR, Kane RA, Lee FT, Jr, et al. Image-guided tumor ablation: Standardization of terminology and reporting criteria. Radiology 2005; 235: 728–739
- Fry FJ, Sanghvi NT, Morris RF, Smithson S, Atkinson L, Dines K, Franklin TD, Hastings J. A focused ultrasound system for tissue volume ablation in deep seated brain sites. IEEE Ultrason Symp 1986; 1001–1004
- Lizzi FL, Coleman DJ, Driller J, Silverman RH, Lucas BC, Rosado AL. A therapeutic ultrasound system incorporating real-time ultrasonic scanning. IEEE Symp Son Ultrason 1986; 981–984
- Ter Haar GR, Sinnet D, Rivens IH. High intensity focused ultrasound—A surgical technique for the treatment of discrete liver tumours. Phys Med Biol 1989; 34: 1743–1750
- Vaezy S, Shi X, Martin RW, Chi E, Nelson PI, Bailey MR, Crum LA. Real-time visualization of high-intensity focused ultrasound treatment using ultrasound imaging. Ultrasound Med Biol 2001; 27: 33–42
- Rabkin BA, Zderic V, Vaezy S. Hyperecho in ultrasound images of HIFU therapy: Involvement of cavitation. Ultrasound Med Biol 2005; 31: 947–956
- Chan AH, Fujimoto VY, Moore DE, Martin RW, Vaezy S. An image-guided high intensity focused ultrasound device for uterine fibroids treatment. Med Phys 2002; 29: 2611–2620
- Bailey MR, Couret LN, Sapozhnikov OA, Khokhlova VA, Ter Haar G, Vaezy S, Shi X, Martin R, Crum LA. Use of overpressure to assess the role of bubbles in focused ultrasound lesion shape in vitro. Ultrasound Med Biol 2001; 27: 695–708
- Khokhlova VA, Bailey MR, Reed JA, Cunitz BW, Kaczkowski PJ, Crum LA. Effects of nonlinear propagation, cavitation, and boiling in lesion formation by high intensity focused ultrasound in a gel phantom. J Acoust Soc Am 2006; 119: 1834–1848
- Ophir J, Cespedes I, Ponnekanti H, Yazdi Y, Li X. Elastography: A quantitative method for imaging the elasticity of biological tissues. Ultrason Imaging 1991; 13: 111–134
- Kallel F, Stafford RJ, Price RE, Righetti R, Ophir J, Hazle JD. The feasibility of elastographic visualization of HIFU-induced thermal lesions in soft tissues. Image-guided high-intensity focused ultrasound. Ultrasound Med Biol 1999; 25: 641–647
- Varghese T, Ophir J, Cespedes I. Noise reduction in elastograms using temporal stretching with multicompression averaging. Ultrasound Med Biol 1996; 22: 1043–1052
- Bamber JC, Bush NL. Freehand elasticity imaging using speckle decorrelation rate. Acoust Imaging 1995; 22: 285–292
- Doyley MM, Bamber JC, Fuechsel F, Bush NL. A freehand elastographic imaging approach for clinical breast imaging: System development and performance evaluation. Ultrasound Med Biol 2001; 27: 1347–1357
- Garra BS, Cespedes EI, Ophir J, Spratt SR, Zuurbier RA, Magnant CM, Pennanen MF. Elastography of breast lesions: Initial clinical results. Radiology 1997; 202: 79–86
- Souchon R, Soualmi L, Bertrand M, Chapelon JY, Kallel F, Ophir J. Ultrasonic elastography using sector scan imaging and a radial compression. Ultrasonics 2002; 40: 867–871
- de Korte CL, Pasterkamp G, van der Steen AF, Woutman HA, Bom N. Characterization of plaque components with intravascular ultrasound elastography in human femoral and coronary arteries in vitro. Circulation 2000; 102: 617–623
- Stafford RJ, Kallel F, Price RE, Cromeens DM, Krouskop TA, Hazle JD, Ophir J. Elastographic imaging of thermal lesions in soft tissue: A preliminary study in vitro. Ultrasound Med Biol 1998; 24: 1449–1458
- Curiel L, Souchon R, Rouviere O, Gelet A, Chapelon JY. Elastography for the follow-up of high-intensity focused ultrasound prostate cancer treatment: Initial comparison with MRI. Ultrasound Med Biol 2005; 31: 1461–1468
- Kolen AF, Miller NR, Ahmed EE, Bamber JC. Characterization of cardiovascular liver motion for the eventual application of elasticity imaging to the liver in vivo. Phys Med Biol 2004; 49: 4187–4206
- Varghese T, Shi H. Elastographic imaging of thermal lesions in liver in-vivo using diaphragmatic stimuli. Ultrason Imaging 2004; 26: 18–28
- Fatemi M, Greenleaf JF. Vibro-acoustography: An imaging modality based on ultrasound-stimulated acoustic emission. Proc Natl Acad Sci USA 1999; 96: 6603–6608
- Nightingale KR, Palmeri ML, Nightingale RW, Trahey GE. On the feasibility of remote palpation using acoustic radiation force. J Acoust Soc Am 2001; 110: 625–634
- Nightingale K, Soo MS, Nightingale R, Trahey G. Acoustic radiation force impulse imaging: In vivo demonstration of clinical feasibility. Ultrasound Med Biol 2002; 28: 227–235
- Barannik EA, Girnyk A, Tovstiak V, Marusenko AI, Emelianov SY, Sarvazyan AP. Doppler ultrasound detection of shear waves remotely induced in tissue phantoms and tissue in vitro. Ultrasonics 2002; 40: 849–852
- Melodelima D, Bamber JC, Duck FA, Shipley JA, Xu L. Elastography for breast cancer diagnosis using radiation force: System development and performance evaluation. Ultrasound Med Biol 2006; 32: 387–396
- Srinivasan S, Righetti R, Ophir J. Trade-offs between the axial resolution and the signal-to-noise ratio in elastography. Ultrasound Med Biol 2003; 29: 847–866
- Righetti R, Ophir J, Ktonas P. Axial resolution in elastography. Ultrasound Med Biol 2002; 28: 101–113
- Srinivasan S, Righetti R, Ophir J. An experimental characterization of elastographic spatial resolution: Analysis of the trade-offs between spatial resolution and contrast-to-noise ratio. Ultrasound Med Biol 2004; 30: 1269–1280
- Righetti R, Srinivasan S, Ophir J. Lateral resolution in elastography. Ultrasound Med Biol 2003; 29: 695–704
- Bush NL, Rivens I, Ter Haar GR, Bamber JC. Acoustic properties of lesions generated with an ultrasound therapy. Ultrasound Med Biol 1993; 19: 789–801
- Garra BS, Shawker TH, Nassi M, Russell MA. Ultrasound attenuation measurements of the liver in vivo using a commercial sector scanner. Ultrason Imaging 1984; 6: 396–407
- Kuc R, Taylor KJ. Variation of acoustic attenuation coefficient slope estimates for in vivo liver. Ultrasound Med Biol 1982; 8: 403–412
- Maklad NF, Ophir J, Balsara V. Attenuation of ultrasound in normal liver and diffuse liver disease in vivo. Ultrason Imaging 1984; 6: 117–125
- Parker KJ, Asztely MS, Lerner RM, Schenk EA, Waag RC. In-vivo measurements of ultrasound attenuation in normal or diseased liver. Ultrasound Med Biol 1988; 14: 127–136
- Zagzebski JA, Lu ZF, Yao LX. Quantitative ultrasound imaging: In vivo results in normal liver. Ultrason Imaging 1993; 15: 335–351
- Zderic V, Keshavarzi A, Andrew MA, Vaezy S, Martin RW. Attenuation of porcine tissues in vivo after high-intensity ultrasound treatment. Ultrasound Med Biol 2004; 30: 61–66
- Ribault M, Chapelon JY, Cathignol D, Gelet A. Differential attenuation imaging for the characterization of high intensity focused ultrasound lesions. Ultrason Imaging 1998; 20: 160–177
- Civale J, Bamber JC, Rivens I, Ter Haar G. Optimising HIFU lesion formation with backscatter attenuation estimation (BAE). AIP Conference Proceedings. Proceedings of the 5th International Symposium on Therapeutic Ultrasound. 2006. 829: 176–180
- Fatemi M, Greenleaf JF. Real-time assessment of the parameter of nonlinearity in tissue using ‘nonlinear shadowing’. Ultrasound Med Biol 1996; 22: 1215–1228
- Zhang D, Gong XF. Experimental investigation of the acoustic nonlinearity parameter tomography for excised pathological biological tissues. Ultrasound Med Biol 1999; 25: 593–599
- Cline HE, Schenck JF, Watkins RD, Hynynen K, Jolesz FA. Magnetic resonance-guided thermal surgery. Magn Reson Med 1993; 30: 98–106
- Hynynen K, Freund WR, Cline HE, Chung AH, Watkins RD, Vetro JP, Jolesz FA. A clinical, noninvasive, MR imaging-monitored ultrasound surgery method. Radiographics 1996; 16: 185–195
- Hynynen K, Darkazanli A, Unger E, Schenck JF. MRI guided non-invasive ultrasound surgery. Med Phys 1993; 20: 107–115
- McDannold N, Hynynen K, Wolf D, Wolf G, Jolesz F. MRI evaluation of thermal ablation of tumors with focused ultrasound. J Magn Reson Imaging 1998; 8: 91–100
- McDannold NJ, Jolesz FA, Hynynen KH. Determination of the optimal delay between sonications during focused ultrasound surgery in rabbits by using MR imaging to monitor thermal buildup in vivo. Radiology 1999; 211: 419–426
- Hynynen K, Darkazanli A, Damianou CA, Unger E, Schenck JF. The usefulness of a contrast agent and gradient-recalled acquisition in a steady-state imaging sequence for magnetic resonance imaging-guided noninvasive ultrasound surgery. Invest Radiol 1994; 29: 897–903
- Chung AH, Jolesz FA, Hynynen K. Thermal dosimetry of a focused ultrasound beam in vivo by magnetic resonance imaging. Med Phys 1999; 26: 2017–2026
- Chen L, Bouley D, Yuh E, D’Arceuil H, Butts K. Study of focused ultrasound tissue damage using MRI and histology. J Magn Reson Imaging 1999; 10: 146–153
- Vykhodtseva N, Sorrentino V, Jolesz FA, Bronson RT, Hynynen K. MRI detection of the thermal effects of focused ultrasound on the brain. Ultrasound Med Biol 2000; 26: 871–880
- Foldes K, Hynynen K, Shortkroff S, Winalski CS, Collucci V, Koskinen SK, McDannold N, Jolesz F. Magnetic resonance imaging-guided focused ultrasound synovectomy. Scand J Rheumatol 1999; 28: 233–237
- Chen L, Bouley DM, Harris BT, Butts K. MRI study of immediate cell viability in focused ultrasound lesions in the rabbit brain. J Magn Reson Imaging 2001; 13: 23–30
- Bohris C, Jenne JW, Rastert R, Simiantonakis I, Brix G, Spoo J, Hlavac M, Nemeth R, Huber PE, Debus J. MR monitoring of focused ultrasound surgery in a breast tissue model in vivo. Magn Reson Imaging 2001; 19: 167–175
- Hynynen K, Pomeroy O, Smith DN, Huber PE, McDannold NJ, Kettenbach J, Baum J, Singer S, Jolesz FA. MR imaging-guided focused ultrasound surgery of fibroadenomas in the breast: A feasibility study. Radiology 2001; 219: 176–185
- Salomir R, Palussiere J, Vimeux FC, De Zwart JA, Quesson B, Gauchet M, Lelong P, Pergrale J, Grenier N, Moonen CTW. Local hyperthermia with MR-guided focused ultrasound: Spiral trajectory of the focal point optimized for temperature uniformity in the target region. J Magn Reson Imaging 2000; 12: 571–583
- Sokka SD, King R, Hynynen K. MRI-guided gas bubble enchanced ultrasound heating in in vivo rabbit thigh. Phys Med Biol 2003; 48: 223–241
- Damianou C, Pavlou M, Velev O, Kyriakou K, Trimikliniotis M. High intensity focused ultrasound ablation of kidney guided by MRI. Ultrasound Med Biol 2004; 30: 397–404
- Rowland IJ, Rivens IH, Chen L, Lebozer CH, Collins DJ, Ter Haar GR, Leach MO. MRI study of hepatic tumours following high intensity focused ultrasound surgery. Br J Radiol 1997; 70: 144–153
- Hynynen K, Clement GT, McDannold N, Vykhodtseva N, King R, White PJ, Vitek S, Jolesz FA. 500-element ultrasound phased array system for noninvasive focal surgery of the brain: A preliminary rabbit study with ex vivo human skulls. Magn Reson Med 2004; 52: 100–107
- Rouviere O, Lyonnet D, Raudrant A, Colin-Pangaud C, Chapelon JY, Bouvier R, Dubernard JM, Gelet A. MRI appearance of prostate following transrectal HIFU ablation of localized cancer. Eur Urol 2001; 40: 265–274
- Huber PE, Jenne JW, Rastert R, Simiantonakis I, Sinn H-P, Strittmatter H-J, Von Fournier D, Wannenmacher MF, Debus J. A new noninvasive approach in breast cancer therapy using magnetic resonance imaging-guided focused ultrasound surgery. Cancer Res 2001; 61: 8441–8447
- Damianou C. MRI monitoring of the effect of tissue interfaces in the penetration of high intensity focused ultrasound in kidney in vivo. Ultrasound Med Biol 2004; 30: 1209–1215
- Fatemi M, Manduca A, Greenleaf JF. Imaging elastic properties of biological tissues by low-frequency harmonic vibration. Proc IEEE 2003; 91: 1503–1518
- Wu T, Felmlee JP, Greenleaf JF, Riederer SJ, Ehman RL. MR imaging of shear waves generated by focused ultrasound. Magn Reson Med 2000; 43: 111–115
- Clarke RL, Ter Haar GR. Temperature rise recorded during lesion formation by high-intensity focused ultrasound. Ultrasound Med Biol 1997; 23: 299
- Dickinson RJ. Thermal conduction errors of manganin-constantan thermocouple arrays. Phys Med Biol 1985; 30: 445–453
- Fry WJ, Fry RB. Determination of absolute sound levels and acoustic absorption coefficients by thermocouple probes. J Acoust Soc Am 1954; 26: 311–317
- Glaser KJ, Felmlee JP, Manduca A, Mariappan YK, Ehman RL. Stiffness-weighted magnetic resonance imaging. Magn Reson Med 2006; 55: 59–67
- Cheng H-LM, Purcell CM, Bilbao JM, Plewes DB. Prediction of subtle thermal histopathological change using a novel analysis of Gd-DTPA kinetics. J Magn Reson Imaging 2003; 18: 585–598
- Cheng HLM, Purcell CM, Bilbao JM, Plewes DB. Usefulness of contrast kinetics for predicting and monitoring tissue changes in muscle following thermal therapy in long survival studies. J Magn Reson Imaging 2004; 19: 329–341
- Hynynen K, Chung AH, Colucci V, Jolesz FA. Potential adverse effects of high-intensity focused ultrasound exposure on blood vessels in vivo. Ultrasound Med Biol 1996; 22: 193–201
- Denbow ML, Rivens IH, Rowland IJ, Leach MO, Fisk NM, Ter Haar GR. Preclinical development of noninvasive vascular occlusion with focused ultrasonic surgery for fetal therapy. Am J Obstet Gynecol 2000; 182: 387–392
- Jacobs MA, Herskovits EH, Kim HS. Uterine fibroids: Diffusion-weighted MR imaging for monitoring therapy with focused ultrasound surgery—Preliminary study. Radiology 2005; 236: 196–203
- Hand JW. Guidelines for thermometry in clinical hyperthermia. Front Med Biol Eng 1992; 4: 99–104
- Hynynen K, Edwards DK. Temperature measurements during ultrasound hyperthermia. Am Assoc Phys Med 1989; 16: 618–626
- Chan AK, Myrer JW, Measom GJ, Draper DO. Temperature changes in human patellar tendon in response to therapeutic ultrasoun. J Athl Train 1998; 33: 130–135
- Levine D, Millis DL, Mynatt T. Effects of 3.3-MHz ultrasound on caudal thigh muscle temperature in dogs. Vet Surg 2001; 30: 170–174
- Sommer FG, Sumanaweera TS, Glover G. Tissue ablation using an acoustic waveguide for high-intensity focused ultrasound. Med Phys 1997; 24: 537–538
- Carnochan P, Dickinson RJ, Michael CJ. The practical use of thermocouples for temperature measurement in clinical hyperthermia. Int J Hyperthermia 1985; 2: 1–19
- Hynynen K. The threshold for thermally significant cavitiation in dog's thigh muscle in vivo. Ultrasound Med Biol 1991; 17: 157–169
- Morris HJ, Shaw A, Rivens IH, Ter Haar GR. Temperature measurement in ex vivo bovine liver using fine-wire and thin-film thermocouples. AIP Conference Proceedings. 5th International Symposium on Therapeutic Ultrasound. 2006. 829: 338–342
- Vykhodtseva N. MRI detection of the thermal effects of focused ultrasound on the brain. Ultrasound Med Biol 2000; 26: 871–880
- Ter Haar GR, Dunn F. Linear thermocouple arrays for in vivo observation of ultrasonic hyperthermia fields. Br J Radiol 1984; 57: 257–258
- Drewniak JL, Frizzell LA, Dunn F. Errors resulting from finite beamwidth and sample dimensions in the determination of the ultrasonic-absorption coefficient. J Acoust Soc Am 1990; 88: 967–977
- Holt RG, Roy RA. Measurements of bubble-enhanced heating from focused, MHz-frequency ultrasound in a tissue-mimicking material. Ultrasound Med Biol 2001; 27: 1399–1412
- Huang J, Holt RG, Cleveland RO, Roy RA. Experimental validation of a tractable numerical model for focused ultrasound heating in flow-through tissue phantoms. J Acoust Soc Am 2004; 116: 2451–2458
- Hynynen K, Martin CJ, Watmough DJ, Mallard JR. Errors in temperature measurement by thermocouple probes during ultrasound induced hyperthermia. Br J Radiol 1983; 56: 969–970
- Goss SA, Frizzell LA, Dunn F. Ultrasonic absorption and attenuation in mammalian tissues. Ultrasound Med Biol 1979; 5: 181–186
- Dunn F. Temperature and amplitude dependence of acoustic absorption in tissue. J Acoust Soc Am 1962; 34: 1545–1547
- Morris HJ, Shaw A, Rivens IH, Ter Haar GR. Investigation of temperature measurements using thermocouples in a focused ultrasound field. AIP Conference Proceedings. 6th International Symposium on Therapeutic Ultrasound. 2006, 633–637
- Parker KJ. The thermal pulse decay technique for measuring ultrasonic absorption coefficients. J Acoust Soc Am 1983; 74: 1356–1361
- Bacon DR, Shaw A. Experimental validation of predicted temperature rises in tissue mimicking materials. Phys Med Biol 1993; 38: 1647–1659
- Shaw A, Pay NM, Preston RC. Assessment of the likely thermal index values for pulsed Doppler ultrasonic equipment—Stage II: Experimental assessment of scanner/transducer combinations. NPL Report CMAM 12. 1998
- Shaw A. Ultrasonics-field characterisation—Test objects for determining temperature elevation in diagnostic ultrasound fields. International Technical Commission, Geneva 2006, ed. IEC TS 62306
- Stasiek JA, Kowalewski TA. Thermoliuid crystals applied to heat transfer research. Optoelectron Rev 2002; 10: 1–10
- Cook BD, Werchan RE. Mapping ultrasonic fields with cholesteric liquid crystals. Ultrasonics 1971; 9: 101–102
- Martin K, Fernandez R. A thermal beam-shape phantom for ultrasound physiotherapy transducers. Ultrasound Med Biol 1997; 23: 1267–1274
- Quesson B, De Zwart JA, Moonen CT. Magnetic resonance temperature imaging for guidance of thermotherapy. J Magn Reson Imaging 2000; 12: 525–533
- Cline HE, Hynynen K, Hardy CJ, Watkins RD, Schenck JF, Jolesz FA. MR temperature mapping of focused ultrasound surgery. Magn Reson Med 1994; 31: 628–636
- Hynynen K, Darkazanli A, Damianou CA, Unger E, Schenck JF. Tissue thermometry during ultrasound exposure. Eur Urol 1993; 23: S12–S16
- Hynynen K, Damianou CA, Colucci V, Unger E, Cline HH, Jolesz FA. MR monitoring of focused ultrasonic surgery of renal cortex: Experimental and simulation studies. J Magn Reson Imaging 1995; 5: 259–266
- Hardy CJ, Cline HE, Watkins RD. One-dimensional NMR thermal mapping of focused ultrasound surgery. J Comput Assist Tomogr 1994; 18: 476–483
- Matsumoto R, Mulkern RV, Hushek SG, Jolesz FA. Tissue temperature monitoring for thermal interventional therapy: Comparison of T1-weighted MR sequences. J Magn Reson Imaging 1994; 4: 65–70
- Bohris C, Schreiber WG, Jenne J, Simiantonakis I, Rastert R, Zabel H-J, Huber P, Bader R, Brix G. Quantitative MR temperature monitoring of high-intensity focused ultrasound therapy. Magn Reson Imaging 1999; 17: 603–610
- Arora D, Cooley D, Perry T, Guo J, Richardson A, Moellmer J, Hadley R, Parker D, Skliar M, Roemer RB. MR thermometry-based feedback control of efficacy and safety in minimum-time thermal therapies: Phantom and in-vivo evaluations. Int J Hyperthermia 2006; 22: 29–42
- Kuroda K, Chung AH, Hynynen K, Jolesz FA. Calibration of water proton chemical shift with temperature for noninvasive temperature imaging during focused ultrasound surgery. J Magn Reson Imaging 1998; 8: 175–181
- Ishihara Y, Calderon A, Watanabe H, Okamoto K, Suzuki Y, Kuroda K, Suzuki Y. A precise and fast temperature mapping using water proton chemical shift. Magn Reson Med 1995; 34: 814–823
- McDannold N, Vykhodtseva N, Raymond S, Jolesz FA, Hynynen K. MRI-guided targeted blood–brain barrier disruption with focused ultrasound: Histological findings in rabbits. Ultrasound Med Biol 2005; 31: 1527–1537
- Smith NB, Buchanan MT, Hynynen K. Transrectal ultrasound applicator for prostate heating monitored using MRI thermometry. Int J Radiat Oncol Biol Phys 1999; 43: 217–225
- De Zwart JA, Vimeux FC, Delalande C, Canioni P, Moonen CTW. Fast lipid-suppressed MR temperature mapping with echo-shifted gradient-echo imaging and spectral-spatial excitation. Magn Reson Med 1999; 42: 53–59
- McDannold NJ, King RL, Jolesz FA, Hynynen KH. Usefulness of MR imaging-derived thermometry and dosimetry in determining the threshold for tissue damage induced by thermal surgery in rabbits. Radiology 2000; 216: 517–523
- Vykhodtseva N, McDannold N, Martin H, Bronson RT, Hynynen K. Apoptosis in ultrasound-produced threshold lesions in the rabbit brain. Ultrasound Med Biol 2001; 27: 111–117
- Wu T, Kendell KR, Felmlee JP, Lewis BD, Ehman RL. Reliability of water proton chemical shift temperature calibration for focused ultrasound ablation therapy. Med Phys 2000; 27: 221–224
- Cheng H-LM, Plewes DB. Tissue thermal conductivity by magnetic resonance thermometry and focused ultrasound heating. J Magn Reson Imaging 2002; 16: 598–609
- Wang Y, Plewes DB. An MRI calorimetry technique to measure tissue ultrasound absorption. Magn Reson Med 1999; 42: 158–166
- Hynynen K, McDannold N, Mulkern RV, Jolesz FA. Temperature monitoring in fat with MRI. Magn Reson Med 2000; 43: 901–904
- McDannold N, Hynynen K, Jolesz F. MRI monitoring of the thermal ablation of tissue: Effects of long exposure times. J Magn Reson Imaging 2001; 13: 421–427
- Rademaker G, Jenne JW, Rastert R, Roder D, Schad L-R. Comparison of noninvasive MRT-procedures for the temperature measurement for the application during medical thermal therapies [Vergleich nichtinvasiver MRT-verfahren zur Temperaturmessung fuer den Einsatz bei medizinischen Thermotherapien]. Zeitschrift fur Medizinische Physik 2003; 13: 183–187
- Ong JT, d’Arcy JA, Collins DJ, Rivens IH, Ter Haar GR, Leach MO. Sliding window dual gradient echo (SW-dGRE): T1 and proton resonance frequency (PRF) calibration for temperature imaging in polyacrylamide gel. Phys Med Biol 2003; 48: 1917–1931
- Salomir R, Vimeux FC, De Zwart JA, Grenier N, Moonen CTW. Hyperthermia by MR-guided focused ultrasound: Accurate temperature control based on fast MRI and a physical model of local energy deposition and heat conduction. Magn Reson Med 2000; 43: 342–347
- Vimeux FC, De Zwart JA, Palussieüre J, Fawaz R, Delalande C, Canioni P, Grenier N, Moonen CTW. Real-time control of focused ultrasound heating based on rapid MR thermometry. Invest Radiol 1999; 34: 190–193
- De Zwart JA, Vimeux FC, Palussiere J, Salomir R, Quesson B, Delalande C, Moonen CTW. On-line correction and visualization of motion during MRI-controlled hyperthermia. Magn Reson Med 2001; 45: 128–137
- Palussiere J, Salomir R, Le Bail B, Fawaz R, Quesson B, Grenier N, Moonen CTW. Feasibility of MR-guided focused ultrasound with real-time temperature mapping and continuous sonication for ablation of VX2 carcinoma in rabbit thigh. Magn Reson Med 2003; 49: 89–98
- Quesson B, Vimeux F, Salomir R, De Zwart JA, Moonen CTW. Automatic control of hyperthermic therapy based on real-time fourier analysis of MR temperature maps. Magn Reson Med 2002; 47: 1065–1072
- Mougenot C, Salomir R, Palussiere J, Grenier N, Moonen CTW. Automatic spatial and temporal temperature control for MR-guided focused ultrasound using fast 3D MR thermometry and multispiral trajectory of the focal point. Magn Reson Med 2004; 52: 1005–1015
- Hindley J, Gedroyc WM, Regan L, Stewart E, Tempany C, Hynyen K, McDannold N, Inbar Y, Itzchak Y, Rabinovici J, et al. MRI guidance of focused ultrasound therapy of uterine fibroids: Early results. Am J Roentgenol 2004; 183: 1713–1719
- Tempany CMC, Stewart EA, McDannold N, Quade BJ, Jolesz FA, Hynynen K. MR imaging-guided focused ultrasound surgery of uterine leiomyomas: A feasibility study. Radiology 2003; 226: 897–905
- Moonen CTW, Quesson B, Salomir R, Vimeux FC, De Zwart JA, Van Vaals JJ, Grenier N, Palussiere J. Thermal therapies in interventional MR imaging: Focused ultrasound. Neuroimaging Clin N Am 2001; 11: 737–747
- Seip R, Van Baren P, Cain CA, Ebbini ES. Noninvasive real-time multipoint temperature control for ultrasound phased array treatments. IEEE Trans Ultrason Ferroelectr Freq Control 1996; 43: 1063–1073
- Bamber JC, Hill CR. Ultrasonic attenuation and propagation speed in mammalian tissues as a function of temperature. Ultrasound Med Biol 1979; 5: 149–157
- Maass-Moreno R, Damianou CA, Sanghvi NT. Noninvasive temperature estimation in tissue via ultrasound echo-shifts. Part II. In vitro study. J Acoust Soc Am 1996; 100: 2522–2530
- Maass-Moreno R, Damianou CA. Noninvasive temperature estimation in tissue via ultrasound echo-shifts. Part I. Analytical model. J Acoust Soc Am 1996; 100: 2514–2521
- Sun Z, Ying H. A multi-gate time-of-flight technique for estimation of temperature distribution in heated tissue: Theory and computer simulation. Ultrasonics 1999; 37: 107–122
- Miller NR, Bamber JC, Meaney PM. Fundamental limitations of noninvasive temperature imaging by means of ultrasound echo strain estimation. Ultrasound Med Biol 2002; 28: 1319–1333
- Miller NR, Bamber JC, Ter Haar GR. Imaging of temperature-induced echo strain: Preliminary in vitro study to assess feasibility for guiding focused ultrasound surgery. Ultrasound Med Biol 2004; 30: 345–356
- Miller NR, Bograchev KM, Bamber JC. Ultrasonic temperature imaging for guiding focused ultrasound surgery: Effect of angle between imaging beam and therapy beam. Ultrasound Med Biol 2005; 31: 401–413
- Simon C, Van Baren P, Ebbini ES. Two-dimensional temperature estimation using diagnostic ultrasound. IEEE Trans Ultrason Ferroelectr Freq Control 1998; 45: 1088–1099
- Bamber JC. Ultrasound elasticity imaging: Definition and technology. Eur Radiol 1999; 9: 327–330
- Leon-Villapalos J, Kaniorou-Larai M, Dziewulski P. Full thickness abdominal burn following magnetic resonance guided focused ultrasound therapy. Burns 2005; 31: 1054–1055