Abstract
Purpose: This study was designed to assess pelvic bone temperature during typical treatment regimens of transurethral ultrasound thermal ablation of the prostate to establish guidelines for limiting bone heating.
Methods: Treatment with transurethral planar, curvilinear, and sectored tubular applicators was simulated using an acoustic and biothermal pelvic model that accommodates applicator sweeping, boundary temperature control, and changes in perfusion and attenuation with thermal dose to more accurately model ultrasound energy penetration. The effects of various parameters including power and frequency (5–10 MHz) on bone heating were assessed for a range of prostate cross-sections (3–5 cm) and bone distances (1–3 cm).
Results: All devices can produce significant bone heating (temperatures >50°C, thermal dose >240 EM43°C) without optimization of applied frequency or power for bone <3 cm from the prostate boundary. In small glands (∼3 cm) increasing operating frequency of curvilinear and planar devices can increase bone temperatures, whereas the tubular applicator can be used at 10 MHz to avoid likely bone damage. In larger prostates (4–5 cm wide) increasing frequency reduces bone heating but can substantially increase treatment time. Lowering power can reduce bone temperature but may increase thermal dose by increasing treatment duration. All applicators can be used to treat glands 4–5 cm with limited bone heating by selecting appropriate power and frequency.
Conclusions: Pubic bone heating during ultrasound thermal therapy of the prostate can be substantial in certain situations. Successful realization of this therapy will require patient-specific treatment planning to optimally determine power and frequency in order to minimize bone heating.
Introduction
Prostate thermal therapy is becoming more acceptable as a minimally invasive alternative to surgery for treating prostate cancer or benign prostatic hyperplasia (BPH) Citation[1–3]. Prominent technologies for delivering heat to the prostate include laser, thermal conduction or hot source devices, microwave, radiofrequency (RF), and high-intensity focused ultrasound (HIFU) Citation[1], Citation[4]. The clinical safety and efficacy of transurethral, interstitial, and transrectal approaches with these technologies have been demonstrated Citation[5–10]. Their success depends on achieving complete thermal coagulation of target zones within the prostate while avoiding damage to non-targeted tissue. Ultrasound technology has advantages over other energy modalities in that energy deposition and heating patterns may be more controllable Citation[11], leading to more accurate targeting. Transrectal HIFU offers precise control over thermal coagulation and has been effective in treating recurrent or advanced prostate cancer Citation[12], Citation[13]. Long treatment times may be required for larger prostate volumes Citation[13–15], however, and the anterior portion of the gland may remain untreated due to limitations in accessibility Citation[16].
Catheter-based transurethral ultrasound devices are being developed that may offer increased access to the entire gland, more extensive penetration and spatial control than microwave and RF transurethral technology, and potentially decreased treatment times with respect to transrectal HIFU techniques. The ability of transurethral ultrasound devices to produce precise coagulation zones in vivo under guidance from MR thermal imaging has been demonstrated Citation[17–21]. The pattern of energy deposition from these applicators can be controlled by selecting the shape, size, and frequency of the transducer arrays. Applicators based on planar, curvilinear, and tubular transducers have all shown success in conformal thermal therapy of the prostate tissue in vivo Citation[17], Citation[19], Citation[21–23]. A general schematic of transurethral devices with these three transducer configurations as being developed by our group is shown in . Transducers used in experimental prostate treatments have typically been 3.5–4 mm wide, 6–20 mm in length and have operated at a frequency between 4.7 and 10 MHz Citation[19–21], Citation[23–25]. Planar and curvilinear devices each produce narrow heating zones and utilize mechanical sweeping to treat a large portion of the prostate. Directional tubular transducers, designed with 90- to 270-degree active sectors Citation[20], Citation[22], Citation[25], Citation[26] can be used either without rotation to coagulate sector zones or rotated to treat larger prostate regions. The transducer shape and frequency affect the penetration of ultrasound energy; the planar transducer has a narrow, penetrating unfocused beam, the curvilinear transducer lightly focuses ultrasound energy, producing a narrower beam compared to the planar transducer, and the tubular transducer exhibits a radial decay (1/r) of energy emitted over a pre-defined sector with distance. Recent efforts by Chopra et al. Citation[19], Citation[27] have developed a planar transducer configuration that allows frequency modulation between 4.7 MHz and 10 MHz to adjust the radial depth of average energy deposition. The specific applicator configuration and corresponding energy penetration must be carefully considered in order to properly target specific treatment zones, reduce treatment duration, and avoid the accumulation of thermal dose in sensitive structures beyond the prostate.
Figure 1. Generalized schematic of the catheter-based transurethral ultrasound applicator capable of mechanical rotation within an inflatable urethral cooling balloon, with cross-sections of planar, curvilinear, and sectored tubular transducers (Single active 90° sector). Heating profiles for the three transducer types taken from temperature contours during simulated treatments are shown.
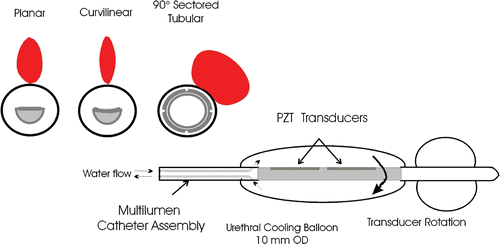
The thermal treatment of soft tissue by ultrasound can be complicated by the presence of bone, causing unwanted heating. High temperature rises at the soft tissue/bone interface can be produced by ultrasound, primarily due to preferential energy absorption Citation[28] resulting from a 20–30 fold greater attenuation in bone than in soft tissue Citation[29]. This can cause patient pain Citation[30], soft tissue necrosis in regions adjacent to bone, and possibly compromise long term bone integrity by inducing osteocyte necrosis Citation[31]. Ultrasound treatments in sites such as the chest wall, brain, and neck necessitate treatment planning and carefully considered applicator design to achieve therapeutic temperature rises in the target tissue while avoiding excessive temperature rises in adjacent bone Citation[32–35]. In contrast, HIFU can be used specifically to target bone for palliation of pain in patients with bone metastases, taking advantage of high bone attenuation to preferentially localize treatment to nerves at the cortical shell of the bone Citation[36]. In addition, there is anecdotal evidence of preferential heating (∼50°C or greater) of bone interfaces, located within 1 cm proximity of the canine prostate, occurring during some in vivo evaluations of transurethral planar ultrasound devices under rotation control, as noted from inspection of previously reported temperature distributions Citation[37], Citation[38].
An inspection of pelvic anatomy reveals that the pubic bones are often in close proximity to the prostate, as seen in , and they should therefore be considered when planning transurethral ultrasound treatments. The position of the pubic bones varies widely among patients, but they can be less than 1 cm from portions of the gland Citation[39–42]. Treatment limiting pain has been documented in ultrasound hyperthermia of the prostate Citation[43] and chest wall Citation[44], highlighting a need to identify and implement strategies to avoid bone temperature rises that may either prevent successful treatment delivery or produce unanticipated tissue damage outside targeted regions.
Figure 2. Transverse CT sections depicting example cases of two seperate patients, one with a 5 cm gland (left) and the other with a 3 cm gland (right), that show the pubic bones in close proximity to the prostate.
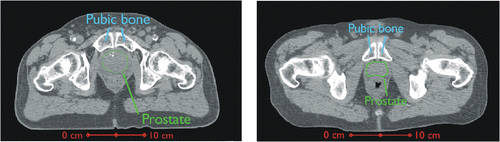
The objective of this study is to investigate the potential impact that pelvic bone may have on applicator design and treatment strategies specific for the three general configurations of transurethral ultrasound devices currently under development. An acoustic and biothermal model of these devices in prostate Citation[21] that incorporates pubic bone was used for this analysis. Ranges of prostate diameter (3–5 cm) and pubic bone distance from the prostate (1–3 cm) were considered in order to model natural patient variation. Bone temperature rises were assessed during simulated treatment of a portion of the prostate with planar, curvilinear, and tubular applicators for various operating parameters: power, frequency, transducer size, radius of curvature (curvilinear), and sweeping rate (planar and curvilinear). The results of this study were used to identify applicator and treatment parameters that dominantly affect bone heating, determine criteria to establish when treatment planning around bone may be necessary, and provide recommendations for applicator design and treatment strategies to limit bone heating during transurethral ultrasound thermal therapy. The intent is to provide general guidelines for the various applicator types, and not to provide a critical assessment of any one approach for applying transurethral ultrasound to the prostate.
Methods
Acoustic and biothermal solver
Theoretical simulations of prostate ablation with transurethral ultrasound devices were performed using a transient acoustic and biothermal model Citation[21], Citation[38], Citation[45] modified to include pelvic bone. The model employs finite difference methods to solve for temperature distributions using the Pennes bioheat transfer equation Citation[46]:where ρt is the tissue density (kg/m3), ct is the tissue heat capacity (J/kg/°C), Tt is the tissue temperature (°C), t is time (s), kt is the conductive heat transfer coefficient (W/m/°C) in the tissue, Tb is the blood temperature (°C), ωb is the mass flow rate of the blood (kg/m3/s), and
is the power deposition (W/m3). At time t = 0, Tb = Tt = 37°C. The tissues within the model are assumed to be isotropic, which reduces the ∇kt term in Equation 1 to kt. The ultrasound power deposition in the tissue was calculated by:
where α is the acoustic absorption coefficient (Np/m/MHz) and v is the ultrasound propagation velocity (m/s). The acoustic pressure distribution
was calculated by the Rayleigh–Sommerfield diffraction integral for the planar and curvilinear transducers using the rectangular radiator method that accounts for wave interactions Citation[47]. The acoustic pressure distribution for the tubular transducer is calculated by a proven geometric method that accounts for transducer shape and tissue attenuation Citation[38], Citation[45]. The Crank–Nicholson method involving mixed implicit and explicit integration is used to improve numerical stability in time with fine grid spacing Citation[45].
In order to accurately model the large change in attenuation between soft tissue and bone, and to achieve stable solutions at tissue interfaces, a radial spacing of 0.05 mm, an angular spacing of 1°, and a temporal spacing of 0.2 s were applied. The large discrete increase in attenuation between bone and periprostatic soft tissue was modelled as a linear increase over 0.5 mm as a means of improving the numerical stability of the finite difference solver. For increased spatial and temporal resolution to achieve higher accuracy with decreased simulation time, a 2D model was considered appropriate. This model is expected to provide a reasonable approximation for determining the extent of potential bone heating and evaluating the impact on applicator design and treatment delivery strategies.
The biothermal model uses cumulative thermal dose thresholds to dynamically change perfusion and acoustic attenuation within soft tissue during heating. Thermal dose is calculated by the method of Sapareto and Dewey Citation[48], Citation[49]. The perfusion is reset from its baseline normothermic value to zero above a thermal dose of 300 equivalent minutes at 43°C (EM43°C), with this threshold substantiated by in vivo measurements of blood flow and vessel damage obtained in rodents and human tumours during thermal exposures Citation[50], Citation[51]. Tissue attenuation is increased linearly to twice its original value as the cumulative thermal dose increases logarithmically from 102 to 107 EM43°C, above which the attenuation no longer increases. This thermal dose–attenuation relationship is substantiated directly by the in vivo studies of Damianou et al. within perfused muscle, liver, and kidney tissue Citation[52], and also supported by numerous in vitro studies which demonstrated a 1.5-2 fold or more increase of attenuation due to heating Citation[53–56]. These changes in perfusion and attenuation with thermal dose, observed during in vivo experiments as a result of tissue necrosis and coagulation, are incorporated to improve the accuracy of the model, particularly in terms of energy penetration and time required to coagulate tissue out to the prostate boundary. Simulations incorporating these changes have been performed by our group and lesion sizes matched to in vivo and ex vivo studies Citation[21], Citation[38], Citation[45], Citation[56]. The threshold for complete soft tissue necrosis is considered in this study to be 240 EM43°C Citation[57], Citation[58], although tissue damage can still occur at lower values Citation[48].
Model geometry and properties
The model consists of a tranverse section through the mid-gland of the prostate including the rectum and pubic bones (). The shape of the prostate was approximated by a cardioid function with the cusp at the posterior margin of the gland, and was varied from 3–5 cm wide at mid-gland to effectively bracket dimensions anticipated in the clinic. The urethra is placed at the centre of the cardioid. The ultrasound applicator is located within the urethra and surrounded by an inflated cooling balloon contiguous with the urethral wall for coupling and protection of the urethral mucosa. The diameter of the balloon is 10 mm and cooling flow is applied at 23°C with a convective heat transfer coefficient (h) = 1000 W/m2/°C. The rectal wall is displaced about 5 mm from the posterior margin of the prostate and is 4 mm thick. An endorectal balloon provides convective cooling flow for rectal protection within the rectum (diameter = 18.5 mm, h = 1000 W/m2/°C, 23°C). Periprostatic tissue is modelled as a mixture of adipose, muscle, and connective tissue. For purposes of this study, two pubic bones were modelled as ovoids with a short axis of 1 cm and a long axis of 2 cm orthogonal to a radial line extending from the centre of the urethra. They are placed anteriolateral to the prostate at a distance of 1, 2, or 3 cm from the gland. The size and shape of the bone in cross-section will vary greatly, and the ovoid shape used in the model is intended solely as a simple approximation to assess bone temperature elevation in a straightforward manner as a sweeping ultrasound applicator rotates from soft tissue and aims directly at bone. The pubic bones have acoustic and thermal properties similar to cortical bone, but unlike soft tissue, the attenuation and perfusion of bone remain unchanged during heating. The acoustic and thermal properties used for the prostate, bone, rectum, and periprostatic tissue within this model are shown in Citation[26], Citation[28], Citation[29], Citation[52], Citation[55], Citation[59–63].
Figure 3. Geometry of the biothermal simulation model with a transurethral ultrasound applicator within the prostatic urethra, the prostate of the variable dimensions the rectum with endorectal cooling, and a simplified representation of pubic bones at variable distance from the prostate capsule. The intended target zone for the simulations is demarcated as a ∼90° sector quadrant of the prostate centered around a peluic bone.
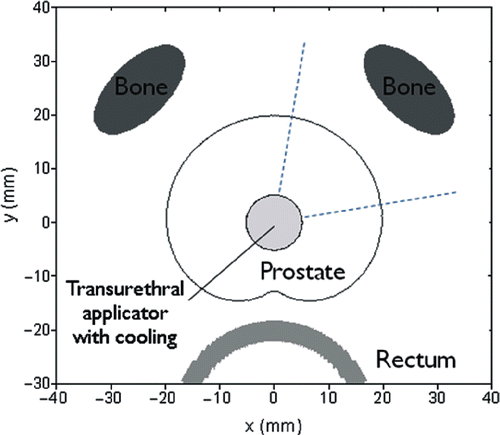
Table I. Thermal and acoustic parameters used for different tissue types in the simulations.
Ultrasound interaction with bone
The absorption, reflection, and transmission of ultrasound energy at a soft-tissue/bone interface is complex Citation[28], Citation[32], Citation[33], Citation[62], Citation[64–66]. When ultrasound is aimed directly at bone, ∼65% of the incident energy is transmitted into the bone in the form of longitudinal waves Citation[28], Citation[62]. As the incident angle of ultrasound on bone increases, the transmission of longitudinal waves decreases but shear waves with a higher attenuation coefficient than longitudinal waves are generated Citation[66]. The generation of shear waves depends strongly on angle; at an incident angle near 40°, the absorption of shear waves can cause temperature rises nearly twice as great as when the angle of incidence is 0° Citation[28]. Marked ultrasound energy absorption in bone results from an extremely high attenuation of 150–350 Np/m/MHz compared to 5–10 Np/m/MHz typical of soft tissues Citation[59], Citation[67]. For this effort, the propagation of ultrasound energy at the soft-tissue/bone interface was approximated using two different absorption models to effectively bracket temperature rises that may occur at the pubic bone: (1) a full transmission model where all energy is transmitted and absorbed into the bone independent of incidence angle, thus approximating the complex absorption of longitudinal and shear waves over all angles; and (2) a partial absorption model where 65% of the energy is transmitted assuming normal incidence and no shear waves. Both of these models assume that the reflected absorbed energy in soft tissue local to the bone interface has a small contribution to the local temperature elevation in bone, indicated by the extreme differences in reported attenuation and absorption Citation[59], Citation[67]. By Equation 2, using values of α, ρ, and v typical of bone and soft tissue, the energy absorbed by the periprostatic tissue is only about 6% of that absorbed by the pubic bone.
Treatment simulations
The targeted treatment zone for all simulations was defined as a 90° sectored portion or quadrant of the prostate gland, centred on a pubic bone. The thermal goal of the treatment is to achieve a temperature of 52°C at the outer prostate boundary, which is sufficient to deliver a lethal thermal dose and coagulation over the target zone Citation[68–70]. Applied power levels were controlled from preset initial values using a bang-bang power controller that reduces applied power as necessary to maintain temperatures within the prostate target below a preset maximum threshold. The maximum temperature threshold was set at 85°C for the 3 cm and 4 cm gland, but increased to 90°C in the 5 cm gland in order to lower treatment time. Pilot-point treatment control was implemented at the target boundary Citation[71]. For the planar and curvilinear applicators, power is applied and regulated as above to treat a narrow zone of the prostate, and successively rotated to the next scan position when the outer target boundary reaches the 52°C threshold. The 90° sectored tubular applicator is centred on the target zone and power is applied as above until 52°C is achieved at the outer boundary - rotation is not required. For each treatment, the maximum temperature and cumulative thermal dose reached in the bone is recorded along with the time required to treat the target zone.
During this simulation study a number of parameters were investigated to determine their influence on temperature elevation at the bone produced with each of the transurethral applicator configurations: prostate width was varied between 3, 4, and 5 cm; separation distance of bone from the prostate was varied between 1, 2, and 3 cm; applied maximum power levels were varied from 3 W to 20 W (with 50% transducer efficiency); frequency was varied between 5, 6.5, 7, 7.5, 8.5, and 10 MHz; transducer width ranged between 3.5–5.0 mm (default width is 3.5 mm, length is 10 mm); radius of curvature for the curvilinear transducers varied from 15–22 mm (default is 15 mm); and sequential rotation step size varied between 2° and 20°, with a default step of 10°.
Results
Temperature and thermal dose distributions
Temperature and cumulative thermal dose distributions resulting from the three different applicator test configurations in the prostate/bone model were calculated for the various applicator design and prostate treatment parameters under consideration. The heating profiles associated with the planar, curvilinear, and tubular applicators are demonstrated for typical cases in . Snapshots of temperature contours during the thermal ablation sequence are shown for a 4 cm wide prostate, with bone at 1 and 2 cm distance (Power = 10 W). In a and b the curvilinear applicator (6.5 MHz, radius of curvature = 15 mm) generates a narrow heating zone that quickly penetrates to the outer capsule of the prostate and is sequentially rotated (10° increments) under temperature feedback control (52°C at boundary) to closely contour the treatment to the target zone boundary. Maximum temperatures calculated from the full absorption model at the bone surface during scanning were 57°C and 54°C for 1 cm and 2 cm bone cases, respectively. The zone of preferential bone heating tracks the applicator sweeping pattern over the bone surface. As shown in c, increasing the operating frequency to 10 MHz reduces peak bone temperature to 45°C for the 1 cm case but increases the relative treatment duration from 14 min to 55 min.
Figure 4. Snapshots of temperature contour profiles for the curvilinear (a,b,c), planar (d,e,f) and tubular (g,h,i), applicators. The planar and corvilinear devices will sweep and produce temperature elevations along the entire bone surface (arrow indicates direction of sweeping). Bone heating is compared at 10 W with an applicator frequency of 6.5 MHz and bone 1 cm from the prostate (a,d,g) or 2 cm from the prostate (b,e,b) to heating with an applicator frequency of 10 MHz (c,f,i). The 52°C contour is bolded in red. A 48°C bolded contour is shown in green for the sectored tubular applicator at 6.5 MHz (g) at an earlier point when bone temperature is higher.
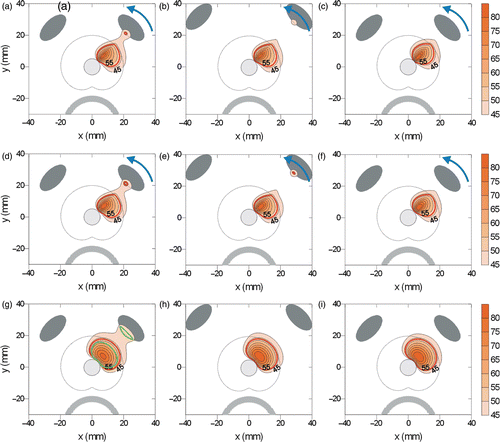
Similarly, a planar applicator (6.5 MHz) contours a selective heating zone for thermal treatment to the outer target boundary, and generates maximum temperatures along the bone surface of 54° for 1 cm bone separation and 50°C for 2 cm separation (e). Increasing the frequency to 10 MHz decreases bone heating to 44°C (f), while increasing treatment time from 9 min to 24 min.
i depicts the typical heating patterns of a stationary sectored tubular applicator (90° sector). A sectored ablation zone extending to the target boundary is produced without applicator rotation. The maximum temperatures obtained along the bone surface in the full absorption model with the 6.5 MHz applicator were 50°C for the 1 cm bone and 42°C for 2 cm bone. Increasing the frequency to 8.5 MHz for the 1 cm case reduces peak bone temperature to 44°C (i) and only slightly increases treatment time from 4.7 min to 6.4 min. In contrast to rotating or sweeping devices, the preferential heating in bone extends along the entire exposed surface over the course of treatment.
Power and frequency effects
The maximum bone temperature and cumulative thermal dose delivered to the pelvic bone during the simulated treatment for the parametric studies is summarized in and . Temperature and thermal dose values plotted in are from the full absorption model, while corresponding values from the 65% absorption model are given in . The results are stratified according to prostate dimension and distance of adjacent pelvic bone. Results for each applicator configuration within the 3 cm prostate are shown in . When bone is 1 cm from the prostate, large temperature rises at the bone interface can occur, particularly when the gland is treated with the planar or curvilinear applicator. At 8 W and 8.5 MHz deleterious bone temperatures of nearly 70°C and necrotic thermal doses of 106 EM43°C are predicted for the planar and curvilinear devices, while for the tubular device peak bone temperature is 49°C and accrued thermal dose at the bone is only slightly above 100 EM43°C. In the 3 cm prostate model, increasing the frequency from 8.5 to 10 MHz for the planar and curvilinear devices causes an increase in bone temperature and thermal dose, but for the tubular device bone temperature decrease and dose decrease (46°C, 10 EM43°C). Power has notable effects in the small 3 cm gland. The influence of power on thermal dose accrued at the bone is shown in b. When the power is reduced from 8 W to 4 W, bone temperature is reduced for all transducer types and thermal dose in the bone is also reduced for the planar and curvilinear applicators. For the tubular applicator, this reduction in power pushes the bone dose above the threshold for necrosis (102 to 320 EM43°C) as required treatment time is increased from 2 min to 11 min. With bone 2 cm away, bone temperatures of 47–51°C and 45–48°C occur with the planar and curvilinear applicators, respectively, but thermal doses are <20 EM43°C due to short treatment duration (<12 min). Bone heating is negligible for the tubular applicator when bone is 2 cm away and for all applicator types when bone is 3 cm away.
Figure 5. Peak temperatures (a) and maximum cumulative thermal dose (b) at the bone surface for a 3 cm prostate treated with a planar, curvilinear, or tubular applicator at 4–8 W and 8.5–10 MHz.
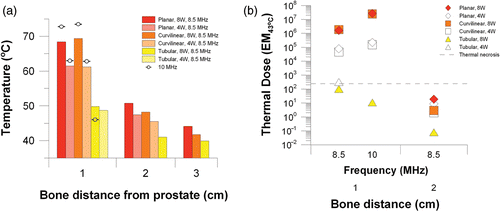
As depicted in , for the 4 cm prostate with bone 1 cm away, all applicator configurations may produce deleterious heating or necrosis. At 6.5 MHz and 10 W, bone temperatures for the planar, curvilinear, and tubular applicators reach 57°C, 53°C, and 50°C, respectively, with respective accompanying thermal doses of 6297 EM43°C, 976 EM43°C, and 370 EM43°C. In the 4 cm prostate, increasing the applicator frequency can reduce bone heating for all applicators. illustrates that bone necrosis can be avoided if the tubular applicator frequency is increased to 8.5 MHz (44°C, 7 EM43°C) and the planar and curvilinear applicator frequencies are increased to 10 MHz (planar–45°C, 5 EM43°C; curvilinear–44°C, 6 EM43°C). Increasing the frequency increases treatment time slightly for the tubular applicator from 4.7 min to 6.4 min (6.5 MHz versus 8.5 MHz) and dramatically for the planar and curvilinear applicators from 9 min to 24 min and from 14 min to 55 min, respectively (6.5 MHz versus 10 MHz). Reducing the power from 10 to 4 W at 6.5 MHz slightly lowers bone temperature but increases thermal dose to the bone due to increased treatment time (planar–9 min to 31 min, curvilinear–14 min to 35 min, tubular–5 min to 49 min). Bone necrosis is still predicted with 2 cm bone separation for the planar applicator at 6.5 MHz, but can be avoided by increasing the operating frequency to 8.5 MHz.
Figure 6. Peak temperatures (a) and maximum cumulative thermal dose (b) at the bone surface for a 4 cm prostate treated with a planar, curvilinear, or tubular applicator at 4–8 W and 8.5–10 MHz.
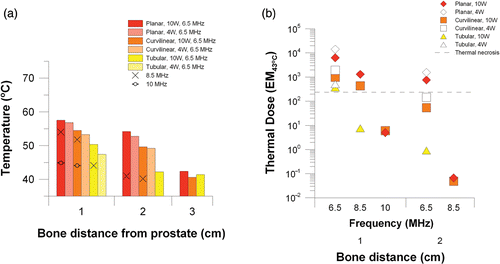
Calculations for the 5 cm prostate model are depicted in , indicating the lower frequency range employed to obtain adequate penetration to the prostate boundary and reduce treatment duration. With 1 cm bone separation, at a 5 MHz drive frequency, treatment is complete for the planar and curvilinear applicators within 22 and 29 minutes, respectively, but bone temperatures exceed 65°C and thermal doses to the bone exceed 106 EM43°C. At 6.5 MHz bone heating is lower but still potentially problematic for these applicators (planar–55°C, 3524 EM43°C; curvilinear–52°C, 1082 EM43°C) and treatment times have increased to 35 minutes for the planar device and 67 minutes for the curvilinear device. With a small frequency increase to 7 MHz, bone temperatures below 50°C and doses below the necrosis threshold of 240 EM43°C are obtained, but the treatment times increase further to 42 minutes for the planar device and to 90 minutes for the curvilinear device. Simulations for the curvilinear device at higher frequency were not performed due to clinically impractical treatment durations. The planar device can be operated at 7.5 MHz to reduce thermal dose at the bone to 22 EM43°C in a 62.2 minute treatment and operated at 8 MHz to further reduce the thermal dose at the bone to 14 EM43úC but the treatment time is 104 minutes. The tubular applicator at 6.5 MHz produces non-lethal temperature elevations at the 1 cm bone interface (45°C, 20 EM43°C) with a treatment time of 15 min. The bone heating and thermal dose exposures were minimal for the 2 cm bone case and negligible for the 3 cm bone case for all applicator configurations.
Other parameters
Increasing the rotation step size greater than 10° can cause marked increases in bone temperature but decreasing it can only slightly reduce bone heating. When rotation step size is increased above 10°/turn (planar applicator, 10W, 5 MHz, 5 cm prostate, bone 1 cm from prostate boundary), bone temperature is maximal when the applicator stops at one step to aim directly at bone (10°/turn–70.3°C, 15°/turn–75.4°C, 20°/turn–80.9°C) and lesser but still notably increased with respect to the 10°/turn step (that does stop to aim directly at bone) when the applicator never aims directly at bone (15°/turn–74.1 °C, 20°/turn–73.7°C). If the applicator is not aimed directly at bone for a 10°/turn step the bone temperature is only reduced from 70.3 to 70.1°C. Bone temperature decreases with decreasing sweep step below 10°/turn by only ∼1°C at most (5°/turn–69.8°C, 2°/turn–69.3°C). Treatment times were not greatly increased or reduced by changing step size (2°/turn–23.4 min, 5°/turn–22.6 min, 10°/turn–21.5 min, 15°/turn–20.8 min, 20°/turn–18.0 min).
Table II. Simulation results comparing power, frequency, and absorption model in their effects on bone temperature, treatment time, and thermal dose with bone 1 cm from the prostate boundary.
Increasing the radius of curvature or applicator width for the curvilinear applicator had small effects on bone heating. When the radius of curvature was increased from the default of 15 mm to 22 mm (6.5 MHz, 10 W, 5 cm wide prostate, bone 1 cm from prostate boundary), peak bone temperature increased from 52.1°C to 53.7°C while treatment time decreased from 67 min to 55 min. When the transducer width was increased from the default of 3.5 mm to 5 mm, peak bone temperature decreased from 52.1°C to 50.4°C while treatment duration decreased to 60 min.
Full absorption and partial transmission models
summarizes a comparison between maximum temperature and thermal dose calculated for example cases using both absorption models for bone located within 10 mm of the prostate capsule. Maximum temperatures and thermal dose exposures using the partial absorption model, which only accounts for partial transmission of the incident ultrasound, are lower than values predicted with the full absorption model for each case as anticipated, but still predictive of trends in producing potentially lethal bone heating (>49°C) and thermal damage (>50 EM43°C) for all applicator types and prostate dimensions for bone 1 cm away. An exception is the 6.5 MHz tubular applicator in the 4 cm wide prostate, which produced potentially non-deleterious bone heating (47°C, <36 EM43°C). The change in treatment times calculated between the two models is less than 1%.
Also shown in is the marked effect that changing the attenuation within the prostate as described earlier can have on simulation results. When the attenuation does not increase with increasing thermal dose, treatment duration is dramatically reduced and bone temperatures are dramatically increased. For the planar applicator at 6.5 MHz in the 5 cm gland, treatment duration is reduced from 35 min to 7 min and the bone temperature increases from 55°C to 90°C.
Discussion
Recent experimental and theoretical studies have demonstrated the potential advantages of applying transurethral ultrasound for prostate thermal therapy compared to other clinical modalities, most notably speed of treatment, a high degree of spatial selectivity or targeting, and compatibility with MR guidance Citation[17], Citation[19–23], Citation[25], Citation[72]. The three general types of transducer configurations being considered (planar, curvilinear, tubular) each have positive performance data supporting continued development and eventual clinical implementation. Explicit study regarding the potential for bone heating and recommendations for avoiding heating with these approaches is important prior to finalizing designs and implementation in the clinic. Although these devices can be integrated with MR temperature monitoring for therapy control, limitations of these monitoring techniques at bone-tissue interfaces reduce the ability to effectively monitor in areas adjacent to and within bone as a means to avoid or control bone heating. The results herein clearly demonstrate that bone heating can be significant and should be considered in applying any of the three techniques. If bone is not properly accounted for, unwanted temperature elevations could potentially generate bone necrosis, treatment limiting pain, and extension or generation of unpredictable soft tissue damage zones. The presence of pelvic bone is most problematic for these transurethral techniques when the bone interface is 2 cm or less from the outer boundary of the prostate or target region. Proper selection of frequency and applied power with a concomitant increase in treatment duration can be applied a priori to reduce unwanted heating in most cases.
The amount of bone heating generated with these transurethral devices is related to the transducer shape as well as to the bone distance, prostate size, and applicator frequency Figures (, ). The curvilinear applicator has the narrowest treatment zone, the planar applicator has a slightly wider treatment zone, and the sectored tubular device can heat over an entire 90° section of the prostate (). The curvilinear and planar devices, while under temperature feedback control, will rotate in sequence over the scan time and produce temperature rises along the bone surface in discrete steps, typically aligned with the centreline direction of the transducer at each step. Varying the rotation step size to 10° or smaller (2°, ∼continuous) was found to produce insignificant differences in the extent of predicted bone heating. However, if the rotation step size was greater than 10°, the amount of bone heating was dramatically increased. In comparison, the small step sizes (≤10°) produce less bone heating since lower applied power and/or duration is required at each position for achieving 52°C at the prostate boundary; this is due to more significant pre-heating of the current target zone from thermal spreading or overlap from the adjacent previously targeted zone, which is in closer proximity when small step sizes are implemented. The sectored tubular applicator is stationary and remains aimed directly at the bone during the complete treatment sequence. Decreasing power can reduce temperatures in bone, but the resultant increase in treatment duration (and timing between rotation movement for curvilinear and planar) can increase thermal dose accumulation. This effect is most dominant in 3–4 cm glands, and less so for the larger 5 cm gland Figures ().
Some of the treatment times calculated in this work appear greater than those predicted for planar applicators in the work of Chopra et al. Citation[19]. These differences can partly be attributed to slight variations in applicator and tissue parameters and control mechanisms between the studies, but the dominant factor is likely the incorporation of attenuation changes in the prostate with accumulated thermal dose in these simulation studies. Coagulation and thermal fixation of prostate tissue at high temperature will cause an increase in ultrasound absorption, effectively mimicking a higher frequency transurethral applicator during a portion of the therapy delivery and therefore limiting energy penetration. It will then become increasingly difficult to extend the coagulation zone to the outer prostate boundary and will require longer treatment duration if the tissue temperature is to be maintained below a maximum threshold. As shown in , these differences can be considerable, and not taking attenuation changes in the prostate into account may underestimate treatment duration and are also predictive of much greater bone temperatures.
The sectored tubular transducer device proved advantageous with respect to the planar and curvilinear device in its ability to treat a predefined or prescribed zone of prostate tissue underlying the pubic bone at lower frequencies with notably less bone heating and shorter treatment times. This was particularly evident when bone was closest; in the 3 cm gland with bone 1 cm from the prostate boundary, necrotic thermal doses in the bone could only be avoided by treatment with the tubular applicator. Reduced temperature elevation at the bone with the tubular applicator is related to the radial decay and divergence of ultrasound energy from the transducer. This ensures that there is lower ultrasound intensity outside of the prostate so that unwanted thermal doses are less likely to develop in structures such as the bladder, rectum, and pubic bones. However, since the tubular applicator is stationary and remains aimed directly at the bone during the complete treatment sequence, bone temperature may be lower but the thermal dose exposure can be considerable and on a level with the curvilinear or planar devices. The tubular applicator does have less spatial selectivity compared to curvilinear and planar configurations, so in practice this advantage could be diminished in situations where boundary control outweighs overall treatment duration and bone heating.
Frequency is the predominant applicator parameter for a given transducer shape that affects the amount of bone heating. Increasing frequency for the tubular applicator reduced bone temperatures in all cases. High frequency operation of the planar and curvilinear applicators enabled a dramatic reduction in bone heating, but only in the 4 and 5 cm glands. In the 3 cm gland, selecting a higher operating frequency for the same applied power level increases bone heating due to a combination of close proximity of the bone to the applicator and higher absorption of the propagated energy within the bone. In larger glands, bone heating is reduced at higher frequency (i.e. 7–10 MHz versus 5–6.5 MHz) because the bone is sufficiently far from the applicator and the ultrasound intensity is sufficiently attenuated by increased absorption within the prostate to compensate for increased absorption by the bone. Higher frequency can be used to limit energy penetration outside the target region, but comes at a cost–increased treatment duration due to limited energy penetration out to the prostate boundary. A novel design configuration of planar transurethral applicators has been developed that utilizes transducers which are capable of emitting multiple distinct frequencies (4.7 MHz and 10 MHz) that can be modulated or blended during treatment delivery Citation[27], Citation[73] as a means of controlling the depth of power penetration. Based upon the results of our study, such a device could prove advantageous to a single frequency device in treating a 4 cm prostate. In the simulations performed in this study, a planar applicator at 10 W and 10 MHz produced bone temperatures of 44.9°C but took 24.4 min to treat a 90° portion of the prostate. Operating at 6.5 MHz, the target zone could be treated in 9.4 min but a much higher peak bone temperature of 57.5°C was produced. A dual frequency device could be operated at 10 MHz adjacent to bone and at 4.7 MHz away from bone to minimize treatment duration and bone temperature. In the 3 cm gland, high frequency operation does not limit bone heating, and in the 5 cm gland, treatment duration may be prohibitively long at higher frequency, and so the utility of this type of device to avoid bone heating in small or large glands is not as clear.
A thermal dose of 240 EM43°C and a bone temperature of 50°C were used in this study as thresholds for deleterious effects on the bone and surrounding tissue. These values are well established for predicting extensive necrosis in soft tissue, including prostate Citation[74], Citation[75]. Thermal necrosis in bone has been observed at 50°C with exposure times from 30 seconds to 6 minutes, which corresponds to thermal doses of 64 to 768 EM43°C Citation[76–79]. While a thermal dose of 240 EM43°C is used as a threshold for complete necrosis, the thresholds for less complete tissue damage may be much lower Citation[48]. Energy accumulated within the cortical bone can contribute to damaging adjacent soft tissue due to thermal redistribution (thermal conduction and blood flow mechanisms). Unwanted temperature elevations near pelvic bone are of particular concern for adjacent nerves, which have a high thermal sensitivity with low thermal dose thresholds for permanent or transient damage Citation[48], Citation[80–82].
Results from the full absorption model are indicative of worst case bone heating. The full absorption model accounts for longitudinal wave transmission and shear wave generation over a large range of incident angles, while the partial absorption model neglects shear waves and can underestimate the resultant bone temperature elevations and thermal dose accrual. The angle of incidence of ultrasound waves on bone affects their reflection, absorption, and generation of shear waves, leading to different amounts of bone heating. These effects, rather than being specifically addressed in the simulations, were approximated by bracketing potential temperature rises with a 65% and a full absorption model of a simplified ‘pubic bone’. A more precise mathematical consideration of wave propagation effects with full anatomically correct models could make the estimates of bone heating and validation of treatment strategies more accurate. Since bone position varies greatly between patients, a pretreatment determination of individual patient anatomy and treatment planning systems Citation[83] could be used to avoid bone heating, and could be used to choose appropriate treatment parameters specific for each patient Citation[83–85].
In summary, the potential exists for producing unwanted temperature rises at the pubic bone interface during transurethral ultrasound thermal therapy of the prostate using tubular, curvilinear, or planar transducer configurations when the bone is less than 3 cm of the outer target boundary. These temperature elevations and cumulative thermal dose exposures can compromise the integrity of the bone tissue, cause treatment limiting patient pain, and potentially damage sensitive or non-targeted tissue adjacent to the bone. Temperature rises may be mitigated by the selection of appropriate ultrasound parameters such as transducer shape, power, frequency, and treatment duration. Treatment planning that uses individual patient anatomy as a guide to selecting these parameters is indicated for the successful administration of transurethral thermal ablation in the prostate for cases where the bone is within 3 cm from the outer target boundary.
Acknowledgements
This work was supported by NIH grants R01CA111981 and R01CA122276.
References
- Shinohara K. Thermal ablation of prostate diseases: Advantages and limitations. Int J Hyperthermia 2004; 20(7)679–697
- Gillett MD, Gettman MT, Zincke H, Blute ML. Tissue ablation technologies for localized prostate cancer. Mayo Clin Proc 2004; 79(12)1547–1555
- Larson TR. Rationale and assessment of minimally invasive approaches to benign prostatic hyperplasia therapy. Urology 2002; 59(2 Suppl 1)12–16
- Diederich CJ. Thermal ablation and high-temperature thermal therapy: Overview of technology and clinical implementation. Int J Hyperthermia 2005; 21(8)745–53
- Hoffman RM, MacDonald R, Monga M, Wilt TJ. Transurethral microwave thermotherapy versus transurethral resection for treating benign prostatic hyperplasia: A systematic review. BJU Int 2004; 94(7)1031–1036
- Gravas S, Melekos MD. Transurethral microwave thermotherapy: From evidence-based medicine to clinical practice. Curr Opin Urol 2007; 17(1)12–16
- Barmoshe S, Zlotta AR. How do I treat and follow my TUNA patients. World J Urol 2006; 24(4)397–404
- Sherar MD, Trachtenberg J, Davidson SR, Gertner MR. Interstitial microwave thermal therapy and its application to the treatment of recurrent prostate cancer. Int J Hyperthermia 2004; 20(7)757–768
- Rewcastle JC. High intensity focused ultrasound for prostate cancer: A review of the scientific foundation, technology and clinical outcomes. Technol Cancer Res Treat 2006; 5(6)619–625
- Poissonnier L, Chapelon JY, Rouviere O, Curiel L, Bouvier R, Martin X, et al. Control of prostate cancer by transrectal HIFU in 227 patients. Eur Urol 2007; 51(2)381–388q7
- Deardorff DL, Diederich CJ, Nau WH. Control of interstitial thermal coagulation: comparative evaluation of microwave and ultrasound applicators. Med Phys 2001; 28(1)104–117
- Chaussy C, Thuroff S, Bergsdorf T. [Local recurrence of prostate cancer after curative therapy. HIFU (Ablatherm) as a treatment option]. Urologe A 2006; 45(10)1271–1275
- Uchida T, Sanghvi NT, Gardner TA, Koch MO, Ishii D, Minei S, et al. Transrectal high-intensity focused ultrasound for treatment of patients with stage T1b-2n0m0 localized prostate cancer: A preliminary report. Urology 2002; 59(3)394–8, discussion 398–399
- Beerlage HP, Thuroff S, Debruyne FM, Chaussy C, de la Rosette JJ. Transrectal high-intensity focused ultrasound using the Ablatherm device in the treatment of localized prostate carcinoma. Urology 1999; 54(2)273–277
- Vallancien G, Prapotnich D, Cathelineau X, Baumert H, Rozet F. Transrectal focused ultrasound combined with transurethral resection of the prostate for the treatment of localized prostate cancer: Feasibility study. J Urol 2004; 171(6 Pt 1)2265–2267
- Rouviere O, Lyonnet D, Raudrant A, Colin-Pangaud C, Chapelon JY, Bouvier R, et al. MRI appearance of prostate following transrectal HIFU ablation of localized cancer. Eur Urol 2001; 40(3)265–274
- Diederich CJ, Stafford RJ, Nau WH, Burdette EC, Price RE, Hazle JD. Transurethral ultrasound applicators with directional heating patterns for prostate thermal therapy: In vivo evaluation using magnetic resonance thermometry. Med Phys 2004; 31(2)405–413
- Pauly KB, Diederich CJ, Rieke V, Bouley D, Chen J, Nau WH, et al. Magnetic resonance-guided high-intensity ultrasound ablation of the prostate. Top Magn Reson Imaging 2006; 17(3)195–207
- Chopra R, Burtnyk M, Haider MA, Bronskill MJ. Method for MRI-guided conformal thermal therapy of prostate with planar transurethral ultrasound heating applicators. Phys Med Biol 2005; 50(21)4957–4975
- Ross AB, Diederich CJ, Nau WH, Gill H, Bouley DM, Daniel B, et al. Highly directional transurethral ultrasound applicators with rotational control for MRI-guided prostatic thermal therapy. Phys Med Biol 2004; 49(2)189–204
- Ross AB, Diederich CJ, Nau WH, Rieke V, Butts RK, Sommer G, et al. Curvilinear transurethral ultrasound applicator for selective prostate thermal therapy. Med Phys 2005; 32(6)1555–1565
- Diederich CJ, Nau WH, Burdette EC, Bustany IS, Deardorff DL, Stauffer PR. Combination of transurethral and interstitial ultrasound applicators for high-temperature prostate thermal therapy. Int J Hyperthermia 2000; 16(5)385–403
- Lafon C, Koszek L, Chesnais S, Theillere Y, Cathignol D. Feasibility of a transurethral ultrasound applicator for coagulation in prostate. Ultrasound Med Biol 2004; 30(1)113–122
- Kinsey AM, Diederich CJ, Tyreus PD, Nau WH, Rieke V, Pauly KB. Multisectored interstitial ultrasound applicators for dynamic angular control of thermal therapy. Med Phys 2006; 33(5)1352–1363
- Diederich CJ, Nau WH, Ross AB, Tyreus PD, Butts K, Rieke V, et al. Catheter-based ultrasound applicators for selective thermal ablation: Progress towards MRI-guided applications in prostate. Int J Hyperthermia 2004; 20(7)739–756
- Diederich CJ, Burdette EC. Transurethral ultrasound array for prostate thermal therapy: initial studies. IEEE Transactions on Ultrasonics, Ferroelectrics and Frequency Control 1996; 43(6)1011–1022
- Chopra R, Luginbuhl C, Foster FS, Bronskill MJ. Multifrequency ultrasound transducers for conformal interstitial thermal therapy. IEEE Trans Ultrason Ferroelectr Freq Control 2003; 50(7)881–889
- Fujii M, Sakamoto K, Toda Y, Negishi A, Kanai H. Study of the cause of the temperature rise at the muscle-bone interface during ultrasound hyperthermia. IEEE Trans Biomed Eng 1999; 46(5)494–504
- Duck F. Physical properties of tissue: A comprehensive reference book. Academic Press Limited, London 1990
- Hynynen K, DeYoung D. Temperature elevation at muscle-bone interface during scanned, focused ultrasound hyperthermia. Int J Hyperthermia 1988; 4(3)267–279
- Smith NB, Temkin JM, Shapiro F, Hynynen K. Thermal effects of focused ultrasound energy on bone tissue. Ultrasound Med Biol 2001; 27(10)1427–1433
- Moros EG, Fan X, Straube WL. Ultrasound power deposition model for the chest wall. Ultrasound Med Biol 1999; 25(8)1275–1287
- Connor CW, Hynynen K. Patterns of thermal deposition in the skull during transcranial focused ultrasound surgery. IEEE Trans Biomed Eng 2004; 51(10)1693–1706
- Hynynen K, Jolesz FA. Demonstration of potential noninvasive ultrasound brain therapy through an intact skull. Ultrasound Med Biol 1998; 24(2)275–283
- Tu SJ, Hynynen K, Roemer RB. Simulation of bidirectional ultrasound hyperthermia treatments of neck tumours. Int J Hyperthermia 1994; 10(5)707–722
- Catane R, Beck A, Inbar Y, Rabin T, Shabshin N, Hengst S, et al. MR-guided focused ultrasound surgery (MRgFUS) for the palliation of pain in patients with bone metastases–preliminary clinical experience. Ann Oncol 2006
- Chopra R, Baker N, Choy V, Boyes A, Tang K, Appu S, et al. Targeted treatment of localized regions within the prostate gland using MRI-guided transurethral ultrasound therapy (http://cds.ismrm.org/protected/07Presentations/0158). Proc Intl Soc Mag Reson Med 2007; 15: 158
- Ross AB, Diederich CJ, Nau WH, Tyreus PD, Gill H, Bouley D, et al. Biothermal modeling of transurethral ultrasound applicators for MR-guided prostate thermal therapy. SPIE-Int Soc Opt Eng Proceedings of the SPIE - The International Society for Optical Engineering 2005; 5698(1)220–7
- Ginestet C, Malet C, Cohen A, Lafay F, Carrie C. Impact of tissues heterogeneities on monitor units calculation and ICRU dose point: Analysis of 30 cases of prostate cancer treated with 18-MV photons after three-dimensional planning. Int J Rad Oncol Biol Phys 2000; 48(2)529–534
- Henderson A, Laing RW, Langley SE. Identification of pubic arch interference in prostate brachytherapy: Simplifying the transrectal ultrasound technique. Brachytherapy 2003; 2(4)240–245
- Strang JG, Rubens DJ, Brasacchio RA, Yu Y, Messing EM. Real-time US versus CT determination of pubic arch interference for brachytherapy. Radiology 2001; 219(2)387–393
- vanHerk M, Bruce A, Kroes APG, Shouman T, Touw A, Lebesque JV. Quantification of organ motion during conformal radiotherapy of the prostate by three dimensional image registration. Int J Rad Oncol Biol Phys 1995; 33(5)1311–1320
- Shimm DS, Hynynen KH, Anhalt DP, Roemer RB, Cassady JR. Scanned focussed ultrasound hyperthermia: Initial clinical results. Int J Radiat Oncol Biol Phys 1988; 15(5)1203–1208
- Corry PM, Barlogie B, Tilchen EJ, Armour EP. Ultrasound-induced hyperthermia for the treatment of human superficial tumors. Int J Radiat Oncol Biol Phys 1982; 8(7)1225–12259
- Tyreus PD, Diederich CJ. Theoretical model of internally cooled interstitial ultrasound applicators for thermal therapy. Phys Med Biol 2002; 47(7)1073–1089
- Pennes H. Analysis of tissue and arterial blood temperatures in the resting human forearm. J App Physiol 1948; 1(2)93–122
- Ocheltree KB, Frizzell LA. Sound field calculation for rectangular sources. IEEE Trans Ultrason Ferroelec Frequency Control 1989; 36(2)242–248
- Dewey WC. Arrhenius relationships from the molecule and cell to the clinic. Int J Hyperthermia 1994; 10(4)457–483
- Sapareto SA, Dewey WC. Thermal dose determination in cancer therapy. Int J Rad Oncol Biol Phys 1984; 10(6)787–800
- Brown SL, Hunt JW, Hill RP. Differential thermal sensitivity of tumor and normal tissue microvascular response during hyperthermia. Int J Hyperthermia 1992; 8(4)501–514
- Lyng H, Monge OR, Bohler PJ, Rofstad EK. Relationships between thermal dose and heat-induced tissue and vascular damage after thermoradiotherapy of locally advanced breast carcinoma. Int J Hyperthermia 1991; 7(3)403–415
- Damianou CA, Sanghvi NT, Fry FJ, Maass-Moreno R. Dependence of ultrasonic attenuation and absorption in dog soft tissues on temperature and thermal dose. J Acoust Soc Am 1997; 102(1)628–634
- Gertner MR, Wilson BC, Sherar MD. Ultrasound properties of liver tissue during heating. Ultrasound Med Biol 1997; 23(9)1395–1403
- Clarke RL, Bush NL, Ter Haar GR. The changes in acoustic attenuation due to in vitro heating. Ultrasound Med Biol 2003; 29(1)127–135
- Worthington AE, Trachtenberg J, Sherar MD. Ultrasound properties of human prostate tissue during heating. Ultrasound Med Biol 2002; 28(10)1311–1318
- Tyreus PD, Diederich C. Two-dimensional acoustic attenuation mapping of high-temperature interstitial ultrasound lesions. Phys Med Biol 2004; 49(4)533–546
- Damianou C, Hynynen K. Focal spacing and near-field heating during pulsed high temperature ultrasound therapy. Ultrasound Med Biol 1993; 19(9)777–787
- Peters RD, Chan E, Trachtenberg J, Jothy S, Kapusta L, Kucharczyk W, et al. Magnetic resonance thermometry for predicting thermal damage: An application of interstitial laser coagulation in an in vivo canine prostate model. Magn Reson Med 2000; 44(6)873–883
- Hynynen KH. Biophysics and technology of ultrasound hyperthermia. Methods of external hyperthermic heating, M Gautherie. Springer-Verlag, Berlin 1990; 61–115
- Liu Z, Lobo SM, Humphries S, Horkan C, Solazzo SA, Hines-Peralta AU, et al. Radiofrequency tumor ablation: Insight into improved efficacy using computer modeling. AJR Am J Roentgenol 2005; 184(4)1347–1352
- Biyikli S, Modest MF, Tarr R. Measurements of thermal properties for human femora. J Biomed Mater Res 1986; 20(9)1335–1345
- Lin WL, Liauh CT, Chen YY, Liu HC, Shieh MJ. Theoretical study of temperature elevation at muscle/bone interface during ultrasound hyperthermia. Med Phys 2000; 27(5)1131–1140
- Moros EG, Straube WL, Myerson RJ, Fan X. The impact of ultrasonic parameters on chest wall hyperthermia. Int J Hyperthermia 2000; 16(6)523–538
- Clement GT, White PJ, Hynynen K. Enhanced ultrasound transmission through the human skull using shear mode conversion. J Acoust Soc Am 2004; 115(3)1356–1364
- Mast TD, Hinkelman LM, Metlay LA, Orr MJ, Waag RC. Simulation of ultrasonic pulse propagation, distortion, and attenuation in the human chest wall. J Acoust Soc Am 1999; 106(6)3665–3677
- White PJ, Clement GT, Hynynen K. Longitudinal and shear mode ultrasound propagation in human skull bone. Ultrasound Med Biol 2006; 32(7)1085–1096
- Goss SA, Johnston RL, Dunn F. Comprehensive compilation of empirical ultrasonic properties of mammalian tissues. J Acoust Soc America 1978; 64(2)423–457
- Graham SJ, Chen L, Leitch M, Peters RD, Bronskill MJ, Foster FS, et al. Quantifying tissue damage due to focused ultrasound heating observed by MRI. Magn Reson Med 1999; 41(2)321–328
- Chen YY, Lin WL, Liou HL, Yen JY, Shieh MJ. Self-tuning fuzzy logic control for ultrasound hyperthermia with reference temperature based on objective functions. Med Phys 1999; 26(5)825–833
- Chopra R, Luginbuhl C, Weymouth AJ, Foster FS, Bronskill MJ. Interstitial ultrasound heating applicator for MR-guided thermal therapy. Phys Med Biol 2001; 46(12)3133–3145
- Liu HL, Chen YY, Yen JY, Lin WL. Pilot point temperature regulation for thermal lesion control during ultrasound thermal therapy. Med Biol Eng Comput 2004; 42(2)178–188
- Chopra R, Wachsmuth J, Burtnyk M, Haider MA, Bronskill MJ. Analysis of factors important for transurethral ultrasound prostate heating using MR temperature feedback. Phys Med Biol 2006; 51(4)827–844
- Lu BY, Lin WL, Chen YY, Yang RS, Kuo TS, Wang CY. A multifrequency driving system for ultrasound hyperthermia. IEEE Eng Med Biol Mag 1999; 18(5)106–111
- Dewhirst MW, Viglianti BL, Lora-Michiels M, Hanson M, Hoopes PJ. Basic principles of thermal dosimetry and thermal thresholds for tissue damage from hyperthermia. Int J Hyperthermia 2003; 19(3)267–294
- Hazle JD, Diederich CJ, Kangasniemi M, Price RE, Olsson LE, Stafford RJ. MRI-guided thermal therapy of transplanted tumors in the canine prostate using a directional transurethral ultrasound applicator. J Magn Reson Imaging 2002; 15(4)409–417
- Nelson CG, Krishnan EC, Neff JR. Consideration of physical parameters to predict thermal necrosis in acrylic cement implants at the site of giant cell tumors of bone. Med Phys 1986; 13(4)462–468
- Lundskog J. Heat and bone tissue. An experimental investigation of the thermal properties of bone and threshold levels for thermal injury. Scand J Plast Reconstr Surg 1972; 9: 1–80
- Bonfield W, Li CH. The temperature dependence of the deformation of bone. J Biomechan 1968; 1(4)323–329
- Eriksson RA, Albrektsson T. The effect of heat on bone regeneration: An experimental study in the rabbit using the bone growth chamber. J Oral Maxillofac Surg 1984; 42(11)705–711
- Willems S, Chen X, Kottkamp H, Hindricks G, Haverkamp W, Rotman B, et al. Temperature-controlled radiofrequency catheter ablation of manifest accessory pathways. Eur Heart J 1996; 17(3)445–452
- Langberg JJ, Calkins H, el-Atassi R, Borganelli M, Leon A, Kalbfleisch SJ, et al. Temperature monitoring during radiofrequency catheter ablation of accessory pathways. Circulation 1992; 86(5)1469–1474
- Monafo WW, Eliasson SG. Sciatic nerve function following hindlimb thermal injury. J Surg Res 1987; 43(4)344–350
- McGough RJ, Kessler ML, Ebbini ES, Cain CA. Treatment planning for hyperthermia with ultrasound phased arrays. IEEE Trans Ultrasonics Ferroelectrics Frequency Control 1996; 43(6)1074–1084
- Moros EG, Novak P, Straube WL, Kolluri P, Yablonskiy DA, Myerson RJ. Thermal contribution of compact bone to intervening tissue-like media exposed to planar ultrasound. Phys Med Biol 2004; 49(6)869–886
- Lagendijk JJ. Hyperthermia treatment planning. Phys Med Biol 2000; 45(5)R61–R76