Abstract
Purpose: To determine the effects of applied current, distance from an RF electrode and baseline tissue temperature upon thermal dosimetry requirements to induce coagulation in ex vivo bovine liver and in vivo porcine muscle models.
Materials and methods: RF ablation was performed in ex vivo liver at varying baseline temperatures–19–21°C (n = 114), 8–10°C (n = 27), and 27–28°C (n = 27)–using a 3-cm tip electrode and systematically varied current 400–1,300 mA, to achieve defined diameters of coagulation (20, 30 and 40 ± 2 mm), and in in vivo muscle (n = 18) to achieve 35 mm ± 2 mm of coagulation. Thermal dose required for coagulation was calculated as the area under the curve and cumulative equivalent minutes at 43°C.
Results: Thermal dose correlated with current in a negative exponential fashion for all three diameters of coagulation in ex vivo experiments (p < 0.001). The temperatures at the end of RF heating at the ablation margin were not reproducible, but varied 38°C–74.7°C, for 30 mm coagulation in ex vivo liver, and 59.8°C–68.4°C in the in vivo experiment. CEM43 correlated with current as a family of positive exponential functions (r2 = 0.76). However, a very wide range of CEM43 values (on the order of 1015) was noted. Although baseline temperatures in the ex vivo experiment did not change required thermal dose, the relationships between end temperature at the ablation margin and RF current were statistically different (p < 0.001) as analysed at the 400 mA intercept.
Conclusions: In both models, thermal dosimetry required to achieve coagulation was not constant, but current and distance dependent. Hence, other formulas for thermal dose equivalence may be needed to predict conditions for thermal ablation.
Introduction
Image-guided percutaneous procedures, such as radiofrequency, microwave and cryoablation have in recent years become viable treatment options for solid focal malignancies in multiple organ sites, like liver Citation[1–3], kidney Citation[4] or lung Citation[5] yielding favorable clinical outcomes and cost effectiveness Citation[6]. Among these options RF ablation is by far the most widely used and well studied. However, completeness of ablation and predictability of coagulation at the tumor periphery, which is of critical clinical importance for reducing probability for local failure, is still challenging to achieve, particularly for tumors over 3–5 cm Citation[7]. Although combining RF with various adjuvants, such as liposomal doxorubicin preparations Citation[8] or intratumor saline Citation[9] or ethanol injection Citation[10] has mitigated this problem somewhat by enabling gains in volume of coagulation, lack of predictability of the amount of coagulation that will be achieved from a single RF application still remains a barrier to more effective therapy. Indeed, reducing variability of ablation outcome, particularly for larger tumors, is challenging given the complexity of potentially influencing parameters including tissue thermal sensitivity Citation[11], electrical and thermal conductivity Citation[12], and perfusion Citation[13] of both tumor and surrounding background tissue Citation[14]. Thus, further gains in increasing coagulation size and improving completeness and predictability of ablation could likely be achieved via a better understanding of how heat interacts with tissue to cause ablation and by applying these principles to clinical practice.
In the field of hyperthermia it has been shown that isoeffects of tissue damage, such as thresholds for necrosis, can be related to a normalized parameter of thermal history, which includes time and temperature. For temperatures up to 48°C, there is a well defined relationship between the rate of cell killing or rate of tissue damage and temperature Citation[10]. In particular, one can normalize thermal history data to an equivalent number of minutes at a standard temperature; usually 43°C. For temperatures above 43°C, the rate of cell killing doubles for each 1.0°C increase in temperature. For temperatures below 43°C, the rate may be somewhat slower, but this point is controversial. In rodent tissues it has been shown that the rate of cell killing drops by a factor of 4 for each 1°C temperature drop below 43°C Citation[15]. Data from human tumor cells suggest that there is not really a change in slope below 43°C; that it remains a slope of 2. The fact that there is a well-defined relationship between rate of tissue damage and temperature gave rise to the idea that any time–temperature history could be converted to an equivalent number of minutes at 43°C. The dose equivalent unit has classically been referred to as cumulative equivalent minutes at 43°C, or CEM43. The practical application for CEM43 would be to permit normalization of prediction of thermal injuries across quite variable thermal histories which are often encountered clinically. In an exhaustive analysis of data on tissue responses to thermal injury, Dewhirst et al. Citation[15] showed that CEM43 works over a wide range of thermal histories to predict everything from minor injuries, such as erythema, to frank thermal injuries, such as burns. Virtually all normal tissues were included in this analysis, based on several hundred publications.
Based on these prior studies, we reasoned that CEM43 could be used to prescribe the thermal history needed to coagulate a tissue, irrespective of how the thermal history was achieved. Thus, if this is correct, the threshold for thermal coagulation for a very high power over a short period of time (as occurs for RF ablation) could be isoeffective of a lower power over a longer period of time (as occurs for hyperthermia) if both thermal histories were converted to CEM43. Yet, thermal data from at least one RF ablation study only partially followed the classic equations derived for the hyperthermia field, as exponential relationships between thermal dose (represented by both AUC and CEM43) and coagulation were noted–indicating that ablative coagulation could potentially be achieved at different CEM43s Citation[17].
To account for the failure of classic thermal dosimetry to predict for injury from thermal ablation, we postulated that the threshold for thermal injury may be influenced by a rate of heat transfer and tissue thermal characteristics, in addition to the thermal history Citation[18]. This led us to perform a set of experiments to determine thermal dosimetry for fixed diameters of coagulation over a range of RF currents. We hypothesized that the thermal history to create ablation would depend upon the radial distance from the electrode, but not the current of RF applied, as opposed to classic hyperthermic concepts that rely only on time–temperature relationships.
Materials and methods
Overview of experimental methods and design
This study was divided into three phases.
Phase one
examined the quantification of thermal dosimetry in ex vivo bovine liver at room temperature (baseline temperature 19–21°C) by creating ablation zones of three specified diameters of coagulation (DOC) of 20, 30 and 40 ± 2 mm using a wide range of RF currents (). Ten doses of RF current (400–1,300 mA in 100 mA intervals) were systematically applied using the CC-1 500 kHz generator (Valleylab, Boulder, CO) and a 3 cm internally cooled electrode. The duration of RF application was varied from 3 to 60 minutes to achieve defined diameters of coagulation. This enabled us to construct sets of curves describing the relationships between thermal parameters and different RF currents to achieve coagulation at specified, well-defined distances from the active electrode from 114 zones of coagulation that matched the specified DOC parameters.
Figure 1. Overview of the experimental design. (a) An internally cooled 3 cm RF electrode (arrow) has been inserted into a sample of bovine liver placed in a 0.9% saline bath. The RF generator and a temperature measurement device can be seen in the background. Thermocouple probes (arrowheads) are placed at defined distances (5, 10, 15, and 20 mm diameter from the RF electrode (arrow), so the end temperature at the ablation margin can be registered. (b) In this case coagulation margin (white arrow) extends to the third thermocouple 15 mm from the electrode. Thermal dose represented as area under the curve for the relationship between temperature and time. (c) Representative temperature–time tracings for multiple currents are shown. With higher RF currents the rate of temperature increase was much higher than with lower currents.
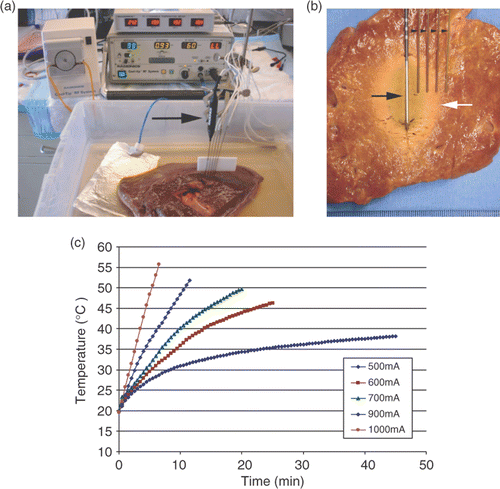
Phase two
investigated the effect of varying baseline tissue temperatures on the outcome of RF ablation and its thermal characteristics by constructing two additional sets of curves (for a 30 ± 2 mm diameter of coagulation), using the same range of RF currents as in phase one. Twenty-seven ablations were created in tissue samples at baseline temperature of 8° to 10°C (achieved by performing RF in a cold-room) and the same number of ablations were created in tissue samples at baseline temperature of 27° to 28°C (achieved by performing RF in a water-bath) varying current from 400 to 1,200 mA, in 100 mA intervals, and duration of RF application from 2 to 70 min.
Phase three:
in vivo studies were performed to confirm our results. Approval of the Institutional Animal Care and Use Committee was obtained before the initiation of these studies. RF ablation was applied to in vivo porcine paraspinal muscles (baseline temperatures 34°C) in two Yorkshire pigs using the CC-1 500 kHz generator and a 3.0 cm internally cooled electrode (n = 18 trials). As in previous phases, duration of RF energy application was varied for the purpose of generating thermal maps for a defined coagulation diameter of 35 mm ± 2 mm. Currents ranging from 700 to 1,200 mA were applied (in 100 mA intervals) with the duration of RF application varied between 4.5 and 12 min.
RF energy deposition
The RF source used for the experiments was a 500 kHz monopolar RF generator (model CC-1; Radionics, Burlington, MA) capable of delivering 2,000 mA. The tissue samples were placed in a normal saline bath (0.9% NaCl solution in distilled water) at: (1) room-temperature (19°–21°C) in phase one and additionally (2) cold-room temperature (8°–10°C) or water-bath (27°–28°C), in phase two. The RF electrode and thermocouples were placed vertically to a depth of 4 to 5 cm within the tissue. The electrical circuit was completed by the way of a standardized 12.5 by 8.0 cm metal grounding pad (for 112 cm2 of grounding return surface area), which was placed horizontally in the bath approximately 20 cm from the electrode (). Continuous RF energy was applied without pulsing at the selected currents for the defined time duration via 17-gauge internally cooled RF electrodes (model CC-1020; Valleylab, Boulder, CO) with a tip exposure of 3 cm. During RF energy application, electrode tip temperatures were maintained at below 10°C by means of continuous perfusion of the internal lumina of the electrode with ∼ 4°C water. High current RF energy was applied according to a previously designed algorithm that has been shown to maximize energy deposition and tissue coagulation for a single applicator Citation[19].
Tissue thermometry
Temperatures were monitored continuously throughout the RF application by means of four thermocouples (Radionics, Burlington, MA) placed at distances of 5, 10, 15 and 20 mm away from the active electrode at the level of midpoint of the active tip and were recorded at 30 s intervals. The thermocouples had a sensitivity of 0.1°C and were connected to an electronic thermal monitor (Radionics TC 4, Burlington, MA) integrated into the RF generator.
Data analyses
Primary endpoints for all experiments included coagulation diameter, duration of RF application, and the isoeffective dose at the margin of the ablation zone. Temperature data were used to calculate thermal history parameters. Minimal threshold temperature for coagulation at the ablation margin was determined by interpolation of actual recorded temperatures. Metrics of thermal dosimetry included area under the curve (AUC, calculated as the integral of temperature minus baseline temperature over time; ), and cumulative equivalent minutes at 43°C (CEM43), using the formula:t = time interval, T = average temperature during time interval t, R = number of minutes needed to compensate for a 1° temperature decrease either above or below the break point (43°C), R is 0.5 for T above 43°C and 0.25 for T below 43°C Citation[20].
Current and distance were analyzed vs. duration of ablation, minimal threshold temperature at the ablation margin, AUC, and CEM43. Given multiple groups, calculated values were correlated to the ablations size to determine best-fit equations using linear and higher order regression analysis. Goodness of fit was determined by the conventional sum of squared errors using proc-NLIN in SAS version 9.1. Then comparison was performed within each experimental group and between groups using multivariate analysis of variance (MANOVA) with SAS software (SAS, Cary, NC) corrected for multiple tests; p < 0.05 was considered statistically significant.
Results
Thermal dose vs. RF current
Duration of RF application vs. RF current
The relationship between duration of RF ablation and current applied was best described by a family of negative power functions of high r2 value of 0.98–0.99 (, ), with high currents requiring significantly shorter duration of energy application (for example for DOC of 30 mm, RF current 400 mA required 60 min, and 1,200 mA required 3.5 min. Functions defining duration of RF ablation for each specified distance likewise showed a statistically significant difference between the ablation diameters of 20, 30, and 40 mm (p < 0.001).
Figure 2. Relationship between duration of RF application and current applied. RF current determined the duration of RF application to achieve specified diameter of coagulation, corresponding with thermal dose requirement in a negative power fashion (p < 0.01). For this and all subsequent figures each trial of the experiments run in triplicate are represented as a data point.
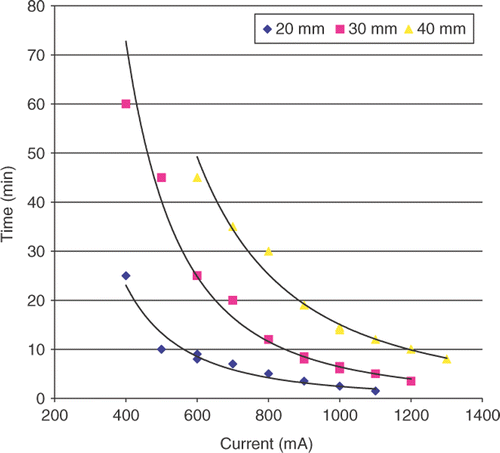
Table I. Summary of the relationships between thermal parameters, distance and current at room temperature for RF coagulation. For all equations ‘x’ represents RF current (mA).
The relationship between AUC (area under the curve) to achieve ablation and current
This was best described by a set of negative exponential functions following the form of: y = α e−βx (, ). Each diameter of coagulation (DOC) produced its own curve and statistically significant differences (both for α and β) were observed between functions for the three different diameters of coagulation (p < 0.001). Correspondingly, the DOCs obtained at low currents required significantly larger thermal dose to create than DOCs achieved with higher currents. For example 30 mm of coagulation achieved at a current of 400 mA required an AUC of 130,817.8°C-sec, whereas a current of 1,200 mA required AUC of 8,850.9°C-sec (). Thus both RF current and distance from the electrode were substantial factors determining overall thermal dose. Similarly, the relationship between CEM43 and current exhibited a correlation, described by a family of positive exponential functions (, ). However a very wide range of CEM43 values (on the order of 1015) needed to achieve ablation was observed.
End temperature at the ablation margin vs. RF current
A correlative relationship between end temperature at the margin and current was demonstrated by a set of positive linear functions (, ). Thus, for each diameter of coagulation, the end temperatures were not constant, but showed wide variability. For example the overall range for the DOC of 30 mm varied 36.7°C from 38°C to 74.7°C with the highest and lowest values recorded at currents of 400 mA and 1,200 mA, respectively. Overall, the duration of heating to achieve thermal coagulation were correlated linearly with thermal dose (r2 = 0.90 − 0.97), whereas the relationship between end temperature and AUC did not correlate as well (r2 = 0.45 − 0.61).
Effects of baseline temperature upon thermal dosimetry
There was no statistically significant difference between the functions describing the relationship between thermal dose (AUC) and current (, ) for all baseline temperatures (p > 0.1 for all comparisons of α and β). Similarly, the analysis of the relationship between duration of RF energy application and current produced a set of three negative power functions () that were not statistically different (p > 0.1). On the other hand, although the end temperatures at the ablation margin showed a linear relationship with the current applied, these relationships were all significantly statistically different (p < 0.001) as analyzed at the 400 mA intercept. Additionally, the slope for the functions representing ablation at room temperature was significantly different from ablation at 8°C and 27°C (; P < 0.05).
Figure 6. Effect of baseline temperature on thermal dose requirement in ex vivo experiment for DOC of 30 ± 2 mm. (a) Negative exponential relationships of thermal dose and current are presented for three different baseline temperatures. (b) Linear relationships of temperature at the ablation margin and current for three investigated baseline temperatures are presented. Different maximum temperatures are noted. (c) Negative power relationships of duration of RF application and current for three investigated baseline temperatures.
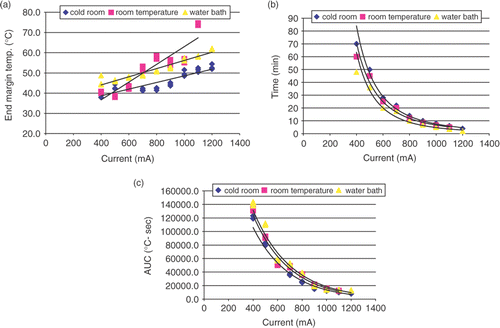
Table II. Summary of relationships between thermal parameters, distance and current at cold-room (8°C) and water-bath (27°C) temperatures for RF coagulation. For all equations ‘x’ represents RF current (mA).
In vivo thermal dosimetry studies
All comparisons were performed for DOC of 35 ± 2 mm. Both the relationship between thermal dose (AUC) and current applied () and between duration of RF ablation and current () were described by negative exponential functions with high correlation (r2 = 0.94 and R2 = 0.98, respectively). A correlative relationship between end temperature at the margin and current was demonstrated by a negative linear function (r2 = 0.68), but showed a narrower range of 8.6°C (59.8°C at 1200 mA vs. 68.4°C at 700 mA) at the end of RF application () compared to that seen in the ex vivo experiment (36.7°C). Similarly to the ex vivo experiment the analysis of CEM43 values needed to achieve ablation showed a wide range of values (in the order of 1011).
Figure 7. Results of in vivo muscle experiment for DOC of 35 ± 2 mm. (a) Negative exponential relationship of AUC and current (r2 = 0.94). (b) Negative exponential relationship between duration of RF application and current (r2 = 0.98). (c) The range of end temperatures at the ablation margin was lower than in ex vivo experiment (8.6°C vs. 36.7°C). (d) CEM43 vs. current showed wide range of values with exponential correlation (r2 = 0.90).
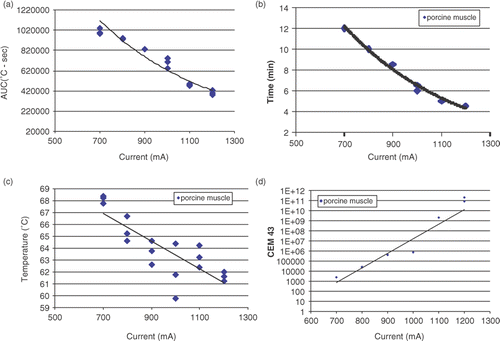
Discussion
During RF ablation procedures, the operator usually imparts very intense thermal doses with temperatures markedly higher (often reaching the boiling point of the tissue) than those used in hyperthermia applications, while energy is applied to tissue for a much shorter period of time (usually below 15–30 min) Citation[21]. Moreover, while in hyperthermia applications once a thermal steady state is achieved (typically within 10–15 min) temperatures do not change appreciably throughout the tumor volume for the rest of the several hours of treatment, during RF ablation the temperature profile is constantly changing across the volume of tissue surrounding a point source radiofrequency field Citation[22]. Thus it is not totally surprising that recently generated data for RF ablation suggested differences in the utility of CEM43 as compared to hyperthermia Citation[16]. Specifically, the very wide range of enormous (>1010) CEM43 values observed in this ( and ) and prior studies, combined with heterogeneity of tumors and energy settings used in clinical applications suggest that CEM43 will not be very useful for RF ablation for many reasons including the fact that it will at a minimum require a level of precision in temperature measurements that is unlikely to be realized in clinical practice in the near future.
Our study therefore sought to determine potential causes of the discrepancy in thermal dosimetry between previous hyperthermia studies and RF ablation. We performed experiments to determine whether thermal dosimetry methods that were originally derived from classic hyperthermia research could be used to predict the degree of tissue coagulation at the margin of ablated zones. To do this we varied the applied RF current in a systematic fashion and measured the time–temperature data at defined radial distances from the RF electrode. In this way, our approach was different from the usual one, where coagulation diameters for specific durations of energy application rather than end-diameters are defined as end-points. Nevertheless, by eliminating the variability of DOC, our approach enabled us to study the effect of thermal dose as effected by RF current applied and coagulation diameter. We found that the classic methods of AUC and CEM43 did not work to predict isoeffects, indicating that these methods were not applicable for predicting the size of thermal ablation zones.
Thermal doses calculated in phase one of our experiment proved to be significantly statistically different (p < 0.01) for different diameters of coagulation (i.e. distances from the electrode) over the investigated current range (, , and ). This relationship could be expected if thermal transfer over distance plays a role in determining ablation outcome. The further away one is from the heating source, the longer one might have to heat to achieve thermal ablation and the rate of heating would be slower the further away one is from the electrode. Nevertheless, our first hypothesis–that variation of thermal dosimetry is caused simply by a delay in thermal transfer over distance–is not supported given the different α and β of the negative exponential relationships generated for each distance from the RF electrode. Had our hypothesis been correct, one would expect thermal dosimetry at each distance to be identical. Additionally, our data revealed that for each current applied, greater thermal dose (as measured by AUC) is required as distance is increased from the electrode.
In phase two of our experiment, we constructed two additional sets of curves describing the relationship of thermal parameters for a coagulation diameter of 30 ± 2 mm to specifically determine the effect of baseline temperature on the ablation outcome and its thermal characteristics (). Although baseline tissue temperature influenced the end temperature at the ablation margin, the overall thermal dose was unchanged. This, coupled with poorer correlation of end temperature to AUC compared to the tighter correlation of duration vs. AUC, suggest that the quite wide range of end temperatures observed in general in our study could be ‘artifactual’, and that the end-temperature at the ablation margin may be viewed as a ‘by-product’ of ablation rather than a reliable predictor of its outcome.
Our findings in the in vivo portion of this study () confirmed the results obtained in ex vivo tissue in that we saw the similar form functions describing the relationships between analyzed parameters suggesting that our results are likely more generalizable to multiple tissue types. Yet noticeable differences included the fact that end-temperatures at the ablation margin for in vivo experiment showed a narrower range in vivo (8.6°C) vs. ex vivo (36.7°C) experiment and that the slope of the relationship between end-temperature and current was negative as compared to the ex vivo where positive slope was noted. We speculate that the narrower range may be due to the narrower range of currents and shorter application times studied in vivo or may be influenced by the low, but present, levels of perfusion in vivo. Alternatively, this and the change in direction of the temperature slope may be due to as yet incompletely characterized differences between liver and muscle tissue.
While Sapareto and Dewey's proposal to convert time–temperature data to an equivalent number of minutes at 43°C (CEM43) termed the ‘thermal isoeffective dose’ Citation[12], is considered to work well for hyperthermia applications, our present study supports the previously noted relationship of time and thermal dosimetry. Yet, all phases of our study demonstrated correlative relationships between RF current and duration, thermal dose, and CEM43 rather than identical results. Indeed, we demonstrated that the thermal dose required at each distance was not constant, but current dependent. Two phenomena could contribute to this observation: (a) the rate of heat transfer from the electrode through the tissue (which varies with the current applied), or (b) possibly an effect of the RF electromagnetic field altering thermal dose. During RF ablation, we are dealing with a temperature gradient that rapidly changes over a volume of tissue exposed to RF field as opposed to hyperthermia, which usually has much more uniform heating over longer period of time with significantly smaller changes in temperature gradients. Yet, the rate of heating has been previously documented as a factor changing how heat interacts with tissues even for a hyperthermia range of temperatures. For example, in one study, increasing the rate of heating decreased the threshold temperature for both arteriolar and venular stasis in normal tissues Citation[23]. The difference in tissue damage was attributed to the development of thermotolerance during slower heating rates. Additionally, careful analysis of older hyperthermia studies also point to the ‘non-constant’ nature of CEM43 at higher temperatures. For example, our reanalysis of data published as early as 1967 by Linke Citation[24] working with liver tissue in rabbits showed a positive linear relationship between temperature and CEM43 from 46°C to 50°C. Similarly, exponentially increasing CEM43 for temperatures from 43°C to 49°C was noted in data from a study published by Braakman Citation[25] in 1989 using high intensity focused ultrasound on retinas in rabbits. We have found that for RF ablation, where the temperatures are even higher, these relationships were exponentially increasing and the difference was of a much greater magnitude (in the order of 1015) ().
Our findings have further potential clinical implications for RF ablation. Effective RF ablation may no longer simply be assumed when temperatures have reached 50°C (or any other arbitrarily selected temperature endpoint) Citation[26]. This is of particular importance as many clinical devices currently use temperature or time as procedure endpoints, assuming that these endpoints are the same for all tissues. Yet prior work notes wide variations in temperature sensitivity for a defined RF dose, and the current study demonstrates that the temperature endpoint varies even in the same tissue at different distances from the RF electrode. Thus, more robust endpoints are needed, and in the interim more conservative endpoints such as longer duration of ablation at higher threshold temperatures should likely be considered.
Based on the above observations, published phantom modeling and studies of finite element modeling of the bio-heat equation may also not be as accurate for predicting ablation outcome as previously assumed. Indeed, since the output is usually based on thermal endpoints, discrepancies between predicted and actual clinical results are likely due in part to selection of a single thermal outcome (such as time to achieve the 50°C isotherm). Clearly, better definitions of endpoints will be necessary to achieve the predictive power of modeling Citation[27]. Thus, these results demonstrate a need for further research on the thermal and electrical components of RF ablation. Understanding the true complexity of the thermal dosimetry of various tissues, at the critical ablation margin is essential for establishing a robust thermal dosimetry method for thermal ablation. Additionally, more work should be performed in vivo using multiple organ sites, to better address wide range of clinical applications. Future investigation should include comparison between different RF current frequencies and various energy sources, such as laser (radiant heat source), ultrasound and microwave ablation, as this may also allow for greater discrimination between the effects of both the electrical and thermal components of ablation thermal dosimetry.
In conclusion, in the two models investigated in our present study, thermal dosimetry required to achieve coagulation, represented by both AUC and CEM43 was not constant, but current- and distance-dependent. While further research is necessary, we postulate that alternative formulas for thermal dose equivalence may be needed, particularly ones that take into account potential modifying effects of the rate of heat transfer and/or the intensity of electromagnetic energy during thermal ablation. Accordingly, our next step will be to conduct experiments that will enable us to determine the roles of these parameters.
Declaration of interest:The authors report no conflicts of interest. The authors alone are responsible for the content and writing of the paper.
References
- Solbiati L, Livraghi T, Goldberg SN, Ierace T, Meloni F, Dellanoce M, Cova L, Halpern EF, Gazelle GS. Percutaneous radio-frequency ablation of hepatic metastases from colorectal cancer: Long-term results in 117 patients. Radiology 2001; 221(1)159–166
- Livraghi T, Goldberg SN, Lazzaroni S, Meloni F, Solbiati L, Gazelle GS. Small hepatocellular carcinoma: Treatment with radio-frequency ablation versus ethanol injection. Radiology 1999; 210(3)655–661
- Lencioni R, Della Pina C, Crocetti L, Cioni D. Percutaneous ablation of hepatocellular carcinoma. Recent Res Cancer Res 2006; 167: 91–105
- Gervais DA, Arellano RS, Mueller P. Percutaneous ablation of kidney tumors in nonsurgical candidates. Oncology (Williston Park) 2005; 19(11Suppl.4)6–11
- Dupuy DE, DiPetrillo T, Gandhi S, Ready N, Ng T, Donat W, Mayo-Smith WW. Radiofrequency ablation followed by conventional radiotherapy for medically inoperable stage I non-small cell lung cancer. Chest 2006; 129(3)738–745
- Tanabe KK, Curley SA, Dodd GD, Siperstein AE, Goldberg SN. Radiofrequency ablation: The experts weigh in. Cancer 2004; 100: 641–650
- Livraghi T, Meloni F, Goldberg SN, Lazzaroni S, Solbiati L, Gazelle GS. Hepatocellular carcinoma: Radio-frequency ablation of medium and large lesions. Radiology 2000; 214: 761–768
- Ahmed M, Liu Z, Lukyanov AN, Signoretti S, Horkan C, Monsky WL, Torchilin VP, Goldberg SN. Combination radiofrequency ablation with intratumoral liposomal doxorubicin: Effect on drug accumulation and coagulation in multiple tissues and tumor types in animals. Radiology 2005; 235: 469–477
- Lobo SM, Afzal KS, Ahmed M, Kruskal JB, Lenkinski RE, Goldberg SN. Radiofrequency ablation: Modeling the enhanced temperature response to adjuvant NaCl pretreatment. Radiology 2004; 230(1)175–182
- Monchik JM, Donatini G, Iannuccilli J, Dupuy DE. Radiofrequency ablation and percutaneous ethanol injection treatment for recurrent local and distant well-differentiated thyroid carcinoma. Ann Surg 2006; 244: 296–304
- Duck FA. Thermal properties of tissues. Physical properties of tissues, FA Duck. Academic Press, San Diego, CA 1990; 167–223
- Liu Z, Ahmed M, Weinstein Y, Yi M, Mahajian RL, Goldberg SN. Characterization of the RF ablation-induced ‘oven effect’: The importance of background tissue thermal conductivity on tissue heating. Int J Hyperther 2006; 22: 327–342
- Horkan C, Ahmed M, Liu Z, Gazelle GS, Solazzo SA, Kruskal JB, Goldberg SN. Radiofrequency ablation: Effect of pharmacologic modulation of hepatic and renal blood flow on coagulation diameter in a VX2 tumor model. J Vasc Interv Radiol 2004; 15(3)269–274
- Solazzo SA, Liu Z, Lobo SM, Ahmed M, Hines-Peralta AU, Lenkinski RE, Goldberg SN. Radiofrequency ablation: Importance of background tissue electrical conductivity–an agar phantom and computer modeling study. Radiology 2005; 236: 495–502
- Dewhirst MW, Viglianti BL, Lora-Michiels M, Hanson M, Hoopes PJ. Basic principles of thermal dosimetry and thermal thresholds for tissue damage from hyperthermia. Int J Hyperther 2003; 19(3)267–294
- Viglianti BL, Lora-Michiels M, Hanson M, Hoopes PJ. Basic principles of thermal dosimetry and thermal thresholds for tissue damage from hyperthermia. Int J Hyperther 2003; 19: 267–294
- Mertyna P, Hines-Peralta A, Liu Z, Halpern E, Goldberg W, Goldberg SN. Radiofrequency ablation: Variability in heat sensitivity in tumors and tissues. J Vasc Interv Radiol 2007; 18: 647–654
- Sapareto SA, Dewey WC. Thermal dose determination in cancer therapy. Int J Radiat Oncol Biol Phys 1984; 10: 787–800
- Goldberg SN, Stein MC, Gazelle GS, Sheiman RG, Kruskal JB, Clouse ME. Percutaneous radiofrequency tissue ablation: Optimization of pulsed-RF technique to increase coagulation necrosis. J Vasc Interv Radiol 1999; 10(7)907–916
- Hall EJ. Hyperthermia. Radiobiology for the radiologist, EJ Hall, AJ Giaccia. Lippincott Williams Wilkins, Philadelphia 2006; 469–488
- Dupuy DE, Goldberg SN. Image-guided radiofrequency tumor ablation: Challenges and opportunities–part II. J Vasc Interv Radiol 2001; 10: 1135–1148
- Cosman Jr ER, Cosman ER Sr. Electric and thermal field effects in tissue around radiofrequency electrodes. Pain Med 2005; 6: 405–424
- Dewhirst MW, Gross JF, Sim D, Arnold P, Boyer D. The effect of rate of heating or cooling prior to heating on tumor and normal tissue microcirculatory blood flow. Biorheology 1984; 21: 539–558
- Linke C, Lounsberry W, Goldschmidt V. Localized heating of tissue by electric and non-electric means. Invest Urol 1967; 4: 586–599
- Braakman R, van der Valk P, van Delft JL, de Wolff-Rouendaal D, Oosterhuis JA. The effects of ultrasonically induced hyperthermia on experimental tumors in the rabbit eye. Invest Ophtalmol Vis Sci 1989; 30: 835–844
- Liu Z, Ahmed M, Sabir A, Humphries S, Goldberg SN. Computer modeling of the effect of perfusion on heating patterns in radiofrequency tumor ablation. Int J Hyperther 2007; 23: 49–58
- Liu Z, Lobo SM, Humphries S, Horkan C, Solazzo SA, Hines-Peralta AU, Lenkinski RE, Goldberg SN. Radiofrequency tumor ablation: Insight into improved efficacy using computer modeling. Am J Roentgenol 2005; 184: 1347–1352