Abstract
Magnetic fluids are increasingly used for clinical applications such as drug delivery, magnetic resonance imaging and magnetic fluid hyperthermia. The latter technique that has been developed as a cancer treatment for several decades comprises the injection of magnetic nanoparticles into tumors and their subsequent heating in an alternating magnetic field. Depending on the applied temperature and the duration of heating this treatment either results in direct tumor cell killing or makes the cells more susceptible to concomitant radio- or chemotherapy. Numerous groups are working in this field worldwide, but only one approach has been tested in clinical trials so far. Here, we summarize the clinical data gained in these studies on magnetic fluid induced hyperthermia.
Introduction
Numerous biomedical and bioengineering applications for superparamagnetic iron oxide nanoparticles have been widely used for a variety of in vivo applications such as magnetic resonance imaging, tissue repair, immunoassay, drug delivery, detoxification of biological fluids, cell separation and hyperthermia Citation[1].
Hyperthermia offers an attractive approach for the treatment of cancer because, as a local therapy, it is associated with fewer side effects in comparison to chemo- and radiotherapy, and it can be used in combination with all conventional treatment modalities. Various clinical trials have demonstrated the efficacy of such combinations Citation[2–6]. However, despite the promising results, hyperthermia has not yet been established in clinical routine. This is most probably not due to a general lack of efficacy but rather to the limitations of the currently available techniques with respect to selectively targeting the tumor region and to homogeneously distributing the heat within the tumor tissue.
Magnetically induced interstitial hyperthermia addresses these shortcomings, especially for deep-seated and poorly accessible tumors. Superparamagnetic biocompatible nanoparticles are directly injected into the tumor tissue where they are stimulated by an alternating magnetic field to produce heat due to Brownian and Néel relaxation processes.
All magnetic nanoparticles used so far in vivo are composed of the iron oxides magnetite (Fe3O4) and maghemite (γ-Fe2O3) due to their low toxicity and their known pathways of metabolism. The crystal structures of both oxides are based on a cubic dense packing of oxygen atoms differing only in the distribution of Fe ions within the crystal lattice. Pure iron oxide nanoparticles tend to agglomerate and hence to build larger structures that strongly influence their biomedical and magnetic properties, even in the absence of a magnetic field. To prevent agglomeration, particles are coated with protective shells (e.g. polymers like dextran, starch or polyethylene glycol). In addition, the shell is responsible for the interaction of the particles with cells and it can provide functional groups useful for the coupling of biomolecules or drugs.
The history of the use of magnetic particles for selective heating of tumors started in 1957 when Gilchrist et al. used particles of a few µm in size for inductive heating of lymph nodes in dogs Citation[7]. More than 20 years later, Gordon et al. used a magnetic fluid (‘dextran-magnetites’ with a core size of up to 6 nm) for hyperthermia after systemic application Citation[8]. Injection of micro-scaled ferromagnetic particles into renal carcinomas of rabbits and subsequent heating was reported by Rand et al. in 1981 Citation[9]. Direct injection of dextran-coated magnetite nanoparticles with a core size of approximately 3 nm into tumors was first reported by our group in 1997 Citation[10]. Hilger et al. injected colloidal suspensions of coated nanoparticles (particle sizes of 10 nm and 200 nm) into human carcinomas implanted into mice and described ‘magnetic thermal ablation’ Citation[11]. Ohno et al. inserted stick-type carboxymethylcellulose magnetite containing nanoparticles into gliomas and described a three-fold prolongation of survival time Citation[12].
Other groups in Japan developed ‘magnetic cationic liposomes’ (MCLs) with improved adsorption and accumulation properties within tumors and demonstrated the efficacy of their technique in several animal tumor models: rat glioma Citation[13–15], melanoma (in combination with immunotherapy) Citation[16], Citation[17] and prostate cancer Citation[18].
A different approach of using magnetic nanoparticles for heating tumor cells is termed ferromagnetic embolization hyperthermia. This technique, which uses a feeding artery to carry nanoparticles into the tumor, seems to be especially well suited for the treatment of hepatic malignancies due to the differences in blood supply between hepatic tumor cells and normal liver parenchyma (liver tumors mainly derive their blood supply from the hepatic arterial system) Citation[19], Citation[20]. Potential advantages are a very selective local heat deposition and a more homogeneous tissue temperature distribution compared to other hyperthermia techniques. Several preclinical studies on arterial embolization hyperthermia of liver cancer used lipiodol suspensions Citation[21–25]. Moroz and co-workers concluded from their data that for a given tumor iron concentration, larger tumors heat at a greater rate than small tumors due to the poorer tissue cooling and better heat conduction in the necrotic regions of large tumors Citation[22].
In a study performed by Minamimura et al. Citation[26] a novel preparation of microspheres incorporating a dextran magnetite complex was used for arterial embolization and inductive hyperthermia of liver tumors in rats. Jones et al. Citation[27] achieved positive temperature differences between tumor and normal liver and subsequent therapeutic responses in experimental rabbit liver tumors after arterially infused ferromagnetic microspheres. Although encouraging results have been achieved in the above-mentioned preclinical studies on arterial embolization hyperthermia, only Granov and co-workers reported on clinical use Citation[28].
Interstitial hyperthermia following direct injection of nanoparticles has also been proven successful in many animal models. A variety of magnetic fluids have been applied using related techniques (differing mostly in the formulation of the nanoparticles), but so far only one of these approaches has been successfully translated from research to clinical stage.
Magnetic fluid hyperthermia
Pre-clinical experience
Magnetic fluid hyperthermia (MFH) also termed ‘Nano-Cancer® therapy’ has been developed by our group over the last 15 years Citation[29]. Preclinical studies demonstrated the feasibility and efficacy of this approach and the potential to improve heating of certain cancer types being previously inaccessible to hyperthermia, and thereby extenuating the existing objections against hyperthermia for cancer therapy.
In a rat model of intracerebral glioblastoma animals received two thermotherapy treatments within 48 h after a single stereotactic injection of the magnetic fluid. Intratumoral treatment temperatures of 43°–47°C correlated well with an up to 4.5-fold prolongation of survival Citation[30]. Thermotherapy was carried out using an alternating magnetic field applicator system for small animals operating at a frequency of 100 kHz and variable field strength of 0–18 kA/m.
To explore the potential of magnetic nanoparticle thermotherapy for prostate cancer we carried out experiments using the Dunning R3327 tumor model Citation[31]. Animals received two thermotherapy treatments carried out 48 hours apart to avoid heat shock protein-induced thermoresistance. Intraprostatic temperatures of 70°C were achieved following injection of up to 0.5 ml of magnetic fluid per cm3 tumor volume. At a constant field strength of 12.6 kA/m, mean minimum intratumoral temperatures during the first and second thermotherapy sessions were 41.2°C and 41.4°C, respectively, whereas mean maximum temperatures were 54.8°C and 54.2°C. Further studies investigated the effect of combined magnetic nanoparticle thermotherapy and external irradiation in the Dunning tumor model Citation[32]. Combined thermotherapy and radiation with 20 Gy was significantly more effective than radiation with 20 Gy alone, and reduced tumor growth by 88% vs. controls, achieving tumor growth inhibition equivalent to irradiation with 60 Gy alone.
Clinical trials
The magnetic fluid MFL AS (Nanotherm®, MagForce Nanotechnologies AG, Berlin, Germany) applied in the MFH studies consists of superparamagnetic iron oxide nanoparticles dispersed in water with an iron concentration of 112 mg/ml (). The iron oxide core is covered by an aminosilane type shell and measures approximately 15 nm in diameter. The particles generate heat in an alternating magnetic field by Brownian and Néel relaxation processes. This fluid was selected due to the high specific absorption rate (SAR) of the particles and the excellent local stability of the deposits within the tumor. It is manufactured according to European medical device regulations and has been approved for clinical trials by a German notified body (approval and licensing authority for medical devices). Quality of the product has been guaranteed by a certified quality assurance system.
Figure 1. Thermotherapy treatment of the pelvic region after intratumoral injection of magnetic nanoparticles using the alternating magnetic field applicator MFH 300F® (MagForce Nanotechnologies AG, Berlin, Germany).
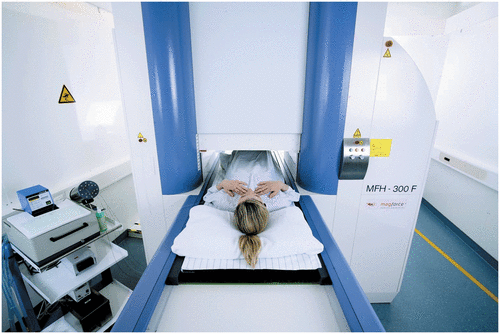
The required magnetic field of 100 kHz and a variable field strength of 0–18 kA/m is generated in the applicator MFH®300F, (MagForce Nanotechnologies AG, Berlin, Germany). The applicator complies with the safety criteria for medical use imposed by the respective European authorities. Due to its universal design, it can be used for the treatment of malignancies at almost every location of the human body. Fiberoptic thermometry probes are integrated for minimally invasive measurements of the treatment temperatures.
Treatment planning is based on thin-sliced CT or MRI scans. Using the newly developed software module Nanoplan® (MagForce Nanotechnologies AG, Berlin, Germany) in combination with the visualization package AMIRA® (Mercury Computer Systems, Berlin, Germany), 3-dimensional reconstruction images are obtained. The number and positions of the magnetic fluid deposits required for sufficient heat deposition are calculated on the basis of the known SAR of the magnetic nanoparticles and the estimated perfusion Citation[33], Citation[34]. The objective is to spread the magnetic fluid within the tumor as homogeneously as possible (with an upper limit between the trajectories of 8–10 mm).
To supersede invasive temperature measurements during future treatments, another objective of the studies was to establish a method for the estimation of intra-tumoral temperatures. Using the same algorithms as for the treatment planning, the calculations were based on the density distribution of the nanoparticles observed in CT and the perfusion data in a process termed post-instillation analysis (PIA). Verification of the calculated data was performed by comparison of the resulting simulated temperature curves with the directly measured temperatures. Therefore, each patient had a thermometry catheter installed into the tumor directly after injection of the nanoparticles, and temperatures were measured along the catheter track during the first two hyperthermia sessions by means of fiberoptic probes.
The total thermal dose was expressed as the cumulative equivalent minutes at 43°C achieved in 90% of the tumor tissue (CEM 43°C T90), summed up for all thermotherapy sessions during the course of treatment (method proposed by Sapareto & Dewey Citation[35]) ().
Figure 2. Postoperative CT scan of a glioblastoma patient showing magnetic nanoparticles deposits as hyperdense areas within the tumor tissue (left hand). Isothermal lines indicate calculated intratumoral temperatures for a magnetic field strength of 8.0 kA/m corresponding to an SAR of 718 W/kg (derived from the Hounsfield units in the CT scan). The brown line (right hand) represents the tumor area identified by preoperative MRI as fused with the CT scan.
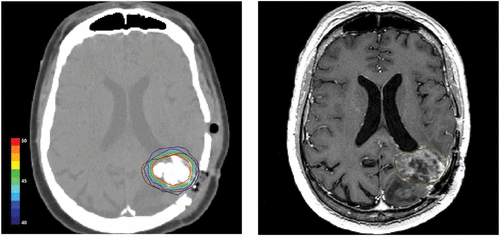
Magnetic fluid hyperthermia in glioblastoma multiforme
In March 2003, the first clinical feasibility study on magnetic nanoparticle hyperthermia was started with 14 patients suffering from glioblastoma multiforme Citation[36]. All patients (2 with primary tumors and 12 with recurrences) received a neuro-navigationally guided injection of the magnetic fluid into the tumor. The preoperatively planned procedure led to almost atraumatic instillation of the particles and was tolerated without complications. The rise of intracranial pressure was avoided by injecting the fluid very slowly.
Patients received 4 to 10 (median 6.5) thermotherapy sessions of 1 hour duration each (twice a week) in combination with external beam radiation. The median injected volume of magnetic fluid was 3 ml (range; 1.0–5.5) corresponding to 0.1–0.7 ml (median: 0.2) of fluid per ml tumor volume.
Thermotherapy was well tolerated at magnetic field strengths of 3.8 to 13.5 kA/m (median: 8.5). Only minor side effects were observed. Maximum intratumoral temperatures of 42.4–49.5°C were measured. In 90% of the tumor volumes temperatures of 39.3°–45.5°C were reached (median 40.5°C). Coverage of the tumors with temperatures above 42°C was approx. 55%. Median CEM 43 T90 was 7.7 minutes (range: 3.2–502).
Magnetic fluid hyperthermia in recurrent and residual tumors
Another prospective feasibility study (start February 2004) enrolled 22 patients with proven recurrences and residual tumors (non-resectable and pre-treated, e.g. prostate and cervix carcinoma, soft tissue sarcoma). All patients received additional radio- (percutaneous, 125I seeds, HDR-brachytherapy) and/or chemotherapy Citation[37].
Depending on pre-treatment and location of the tumors, basically two different techniques for the application of the magnetic fluid were evaluated. One strategy comprised either CT-or TRUS guided and X-fluoroscopy guided (for prostate carcinoma) infiltration after prospective planning of the magnetic fluid distribution on the basis of 3-dimensional CT, MRI or sonography datasets. The other technique consisted of intra-operative injection under visual control when prospective planning was not possible because the degree of non-resectable rests or areas at risk after tumor debulking (R1/2-situation) could not be predicted. Cervical cancer recurrences at the pelvic wall were typical clinical examples for the latter procedure.
Instillation of the nanoparticles was well tolerated by all patients. However, in a few cases pre-irradiated tumor tissue offered considerable mechanical resistance to the injection and probably also to the intratumoral diffusion of the magnetic fluid. Median volumes of CT- and TRUS-guided infiltration were 3 ml (range 1.5–5) and 8.5 ml (range 6–12.5), respectively, corresponding to approximately 0.3–0.4 ml of magnetic fluid per ml tumor volume. The median volume of intra-operatively infiltrated fluid was 7 ml per patient (range 2.3–10). Tolerated H-fields were limited by local discomfort (at skin folds or at bone surfaces) to 3–5 kA/m in the pelvic region and up to 8.5 kA/m in the upper thorax resulting in a median CEM 43 T90 of 10.5 minutes (range 1–106). The heat treatments were well tolerated with only minor to moderate adverse events (e.g. subjective feelings of heat, superficial skin burns, increased pulse rate and rise of blood pressure). In two patients (with additional implantation of 125I seeds) grade 1 to 2 perineal pain persisted for up to 4 months.
Magnetic fluid hyperthermia in recurrent prostate carcinoma
Ten patients with locally recurrent prostate cancer following primary therapy with curative intent were entered into a feasibility trial at the Department of Urology of the Charité–University Medicine Berlin. The magnetic fluid was injected transperineally under transrectal ultrasound and fluoroscopy guidance. Patients received six thermal treatments of 60 minutes duration at weekly intervals Citation[38]. Thermometry in the prostate was carried out invasively during the first and last thermotherapy session and intraluminally in the urethra and in the rectum during each treatment.
Maximum temperatures of up to 55°C were achieved in the prostates. Median temperatures in 90% of the prostates were 40.1°C (range 38.8–43.4) at field strengths of 4 to 5 kA/m. Median urethral and rectal temperatures were 40.5°C (range 38.4–43.6) and 39.8°C (range 38.2–43.4). The median thermal dose was 7.8 (range 3.5–136.4) cumulative equivalent minutes at 43°C in 90% of the prostates Citation[39].
CT scans showed that nanoparticles were retained in the prostate beyond the treatment interval of 6 weeks demonstrating that a single application of the magnetic fluid is sufficient. Nanoparticle deposits were still detectable in the prostates even one year after instillation. No systemic toxicity was observed at a median follow-up of 17.5 months. Acute urinary retention occurred in four patients with previous history of urethral stricture. Treatment-related morbidity was moderate and quality of life was only slightly and temporarily impaired Citation[40].
Discussion
Dispersions of biocompatible iron oxide nanoparticles (magnetic fluids) can be injected into tumors and subsequently heated in an externally applied alternating magnetic field. The large number and overall surface of the magnetic elements within such fluids result in excellent power absorption capabilities and make them particularly suitable for selective interstitial heating of tumors.
The pathways developed so far for hyperthermia using magnetic nanoparticles have in common that they deliver the magnetic material directly into or adjacent to the tumor tissue. The magnetic fluid can be applied in very small portions and therefore almost continuously within the targeted area, leading to high concentrations and homogeneous heat distribution. This addresses the major requirement for hyperthermia, namely that the heat is selectively targeted to the tumor region while sparing neighboring healthy tissue. The local application and the inductive excitation of the nanoparticles result in very precise heating of almost any part of the body. Variation of the magnetic field strength enables an almost free choice of the treatment temperature, rendering both hyperthermia and thermoablation (>46°C) possible.
Nevertheless, most of the techniques in this field applied so far have only been evaluated in preclinical studies.
Magnetic fluid hyperthermia (MFH) has been undergoing clinical testing. In three clinical studies the safety and the feasibility of the technique has been proven. Injection of the nanoparticles using different techniques (stereotactically, CT or ultrasound guided, intraoperatively under visual control) was excellently tolerated.
One particular advantage of this method is the option to plan the magnetic fluid distribution prospectively and to calculate the heat distribution to a highly reliable degree thereafter, due to the density distribution of the nanoparticles in post-instillation CT and the known SAR of the particles. This was confirmed by experimental data showing that about 90% of the injected amount of iron was detectable by CT in tissue samples Citation[39]. We speculate that the remaining 10% represent areas of low iron concentration, presumably in the periphery of larger deposits or needle tracks. A contribution of very low concentrations of iron to the heating process is physically negligible and the lack of detection does not significantly affect the thermal analysis based on CT data.
The stability of the nanoparticle deposits is regarded as another advantage of the method. In an animal model of prostate cancer it was demonstrated that 10 days after injection of the magnetic fluid almost 90% of the dose of ferrites was still detectable in the tumor Citation[32]. Even one year after application, the particles are still detectable by CT. Thus, repeated treatments over a long time period (several weeks) without additional injection of the magnetic fluid are possible, enabling the inclusion into multimodal treatment concepts. The heat treatments were well tolerated with only minor to moderate side effects. However, the H-fields tolerated were limited by local discomfort in the pelvic region and in the upper thorax. The origin of these complaints (pain at bone surfaces and in soft tissue) was not completely understood but could be attributed to two distinct phenomena: Firstly, boundary effects between tissues of different dielectric constants and conductivity (much more pronounced in E-fields during radiofrequency hyperthermia) and secondly skin reactions due to current density increases caused by the narrowing of the current path in skin folds. These effects were only observed during treatments of pelvic and thoracic tumors, i.e. where the cross-section of the body is relatively high. In contrast, the magnetic field strengths tolerated during treatment of brain tumors were much higher.
Another inconvenience of the method is that patients with metallic implants (e.g. artificial hip joints) in less than 30 cm distance from the treatment area have to be excluded from treatment. Teeth's amalgam fillings or gold crowns have to be replaced by ceramics (for head and neck cancer). Metallic clips or seeds of some mm lengths and less than one mm in diameter are permitted due to their very low power absorption capacities. Cardiac pacemakers and implanted defibrillators are exclusion criteria as well.
Conclusion
Magnetic fluid hyperthermia is feasible and can be safely applied in humans. Mean CEM 43°C T90 values achieved in our clinical studies compare favorably to the results of other groups using the same thermal dose definition Citation[41–43].
It is still too early to claim therapeutic advantages for the applied method because survival benefit or time to progression were not defined endpoints of the finished feasibility studies. However, the ongoing Phase II trials will provide an initial indication whether MFH can improve survival and/or quality of life.
Several strategies are available to further improve the effectiveness of treatment. Since field strengths of up to 18 kA/m can be applied with the applicator used and given the quadratic increase of SAR with increasing magnetic field strength, significantly higher temperatures should be achievable by elevating the H-field (after possible modifications of the applicator). Improvement of the instillation techniques resulting in higher concentrations and a more homogeneous distribution of the nanoparticles within the target area are further ways to optimize this promising approach. Furthermore, non-invasive temperature calculations based on the analysis of post-instillation CT data represent another area of ongoing research.
This new treatment approach is currently being investigated in two efficacy trials, namely recurrences of glioblastoma multiforme (in combination with radiotherapy) and prostate cancer (intermediate risk patients, in combination with LDR-brachytherapy). The results of these trials will be of great help in the assessment of thermotherapy as an alternative or extension to the current standard procedures in cancer treatment.
Current new developments include the implementation of target functions on the surface of iron oxide nanoparticles, which will be able to bind specifically to certain tumor cell epitopes or vascular target molecules or accumulate in lymph nodes after systemic administration. New classes of similar targeted particles have been developed for MR-imaging. Therefore specific iron oxide nanoparticles could lead to advancements in thermotherapy, thermo-chemotherapy and diagnostic imaging or combined as so called theranostic approaches Citation[44]. The controlled release of drugs from heat-sensitive particle-drug-conjugates has the potential to reduce side effects of conventional chemotherapeutic regimen.
Declaration of interest: Burghard Thiesen is manager, Andreas Jordan is CSO of MagForce Nanotechnologies AG.
References
- Gupta AK, Gupta M. Synthesis and surface engineering of iron oxide nanoparticles for biomedical applications. Biomaterials 2005; 26: 3995–4021
- Overgaard J. The current and potential role of hyperthermia in radiotherapy. Int J Radiation Oncology Biol Phys 1989; 16: 535–549
- Herman TS, Teicher BA, Jochelson M, Clark J, Svensson G, Coleman CN. Rationale for use of local hyperthermia with radiation therapy and selected anticancer drugs in locally advanced human malignancies. Int J Hyperthermia 1988; 4: 143–158
- Anderson RL, Kapp DS. Hyperthermia in cancer therapy: Current status. Med J Aust 1990; 152: 310–315
- Sneed PK, Stauffer PR, McDermott MW, Diederich CJ, Lamborn KR, Prados MD, Chang S, Weaver KA, Spry L, Malec MK, et al. Survival benefit of hyperthermia in a prospective randomized trial of brachytherapy boost +/− hyperthermia for glioblastoma multiforme. Int J Radiat Oncol Biol Phys 1998; 40: 287–295
- Wust P, Hildebrandt B, Sreenivasa G, Rau B, Gellermann J, Riess H, Felix R, Schlag PM. Hyperthermia in combined treatment of cancer. Lancet Oncology 2002; 3: 487–497
- Gilchrist RK, Shorey WD, Hanselman RC, Parrott JC, Taylor CB, Medal R. Selective Inductive Heating of Lymph Nodes. Annals of Surgery 1957; 146: 596–606
- Gordon RT, Hines JR, Gordon D. Intracellular hyperthermia. A biophysical approach to cancer treatment via intracellular temperature and biophysical alterations. Med Hypotheses 1979; 5: 83–102
- Rand RW, Snow HD, Brown WJ. Thermomagnetic Surgery for Cancer. Journal Of Surgical Research 1981; 33: 177–183
- Jordan A, Scholz R, Wust P, Fahling H, Krause J, Wlodarczyk W, Sander B, Vogl T, Felix R. Effects of magnetic fluid hyperthermia (MFH) on C3H mammary carcinoma in vivo. Int J Hyperthermia 1997; 13: 587–605
- Hilger I, Hiergeist R, Hergt R, Winnefeld K, Schubert H, Kaiser WA. Thermal ablation of tumors using magnetic nanoparticles: An in vivo feasibility study. Invest Radiol 2002; 37: 580–586
- Ohno T, Wakabayashi T, Takemura A, Yoshida J, Ito A, Shinkai M, Honda H, Kobayashi T. Effective solitary hyperthermia treatment of malignant glioma using stick type CMC-magnetite. In vivo study. J Neurooncol 2002; 56: 233–239
- Yanase M, Shinkai M, Honda H, Wakabayashi T, Yoshida J, Kobayashi T. Antitumor immunity induction by intracellular hyperthermia using magnetite cationic liposomes. Jpn J Cancer Res 1998; 89: 775–782
- Shinkai M, Yanase M, Suzuki M, Honda H, Wakabayashi T, Yoshida J, Kobayashi T. Intracellular hyperthermia for cancer using magnetite cationic liposomes. J Magnetism Magnetic Materials 1999; 194: 176–184
- Le B, Shinkai M, Kitade T, Honda H, Yoshida J, Wakabayashi T, Kobayashi T. Preparation of tumor-specific magnetoliposomes and their application for hyperthermia. J Chem Eng Japan 2001; 34: 66–72
- Ito A, Tanaka K, Kondo K, Shinkai M, Honda H, Matsumoto K, Saida T, Kobayashi T. Tumor regression by combined immunotherapy and hyperthermia using magnetic nanoparticles in an experimental subcutaneous murine melanoma. Cancer Sci 2003; 94: 308–313
- Tanaka K, Ito A, Kobayashi T, Kawamura T, Shimada S, Matsumoto K, Saida T, Honda H. Intratumoral injection of immature dendritic cells enhances antitumor effect of hyperthermia using magnetic nanoparticles. Int J Cancer 2005; 116: 624–633
- Kawai N, Ito A, Nakahara Y, Futakuchi M, Shirai T, Honda H, Kobayashi T, Kohri K. Anticancer effect of hyperthermia on prostate cancer mediated by magnetite cationic liposomes and immune-response induction in transplanted syngeneic rats. Prostate 2005; 64: 373–381
- Archer SG, Gray BN. Vascularization of small liver metastases. Br J Surg 1989; 76: 545–548
- Archer SG, Gray BN. Comparison of portal vein chemotherapy with hepatic artery chemotherapy in the treatment of liver micrometastases. Am J Surg 1990; 159: 325–329
- Moroz P, Jones SK, Winter J, Gray BN. Targeting liver tumors with hyperthermia: Ferromagnetic embolization in a rabbit liver tumor model. J Surg Oncol 2001; 78: 22–29, discussion 30–21
- Moroz P, Jones SK, Gray BN. The effect of tumour size on ferromagnetic embolization hyperthermia in a rabbit liver tumour model. Int J Hyperthermia 2002; 18: 129–140
- Mitsumori M, Hiraoka M, Shibata T, Okuno Y, Masunaga S, Koishi M, Okajima K, Nagata Y, Nishimura Y, Abe M, et al. Development of intra-arterial hyperthermia using a dextran-magnetite complex. Int J Hyperthermia 1994; 10: 785–793
- Mitsumori M, Hiraoka M, Shibata T, Okuno Y, Nagata Y, Nishimura Y, Abe M, Hasegawa M, Nagae H, Ebisawa Y. Targeted hyperthermia using dextran magnetite complex: A new treatment modality for liver tumors. Hepatogastroenterology 1996; 43: 1431–1437
- Moroz P, Jones SK, Metcalf C, Gray BN. Hepatic clearance of arterially infused ferromagnetic particles. Int J Hyperthermia 2003; 19: 23–34
- Minamimura T, Sato H, Kasaoka S, Saito T, Ishizawa S, Takemori S, Tazawa K, Tsukada K. Tumor regression by inductive hyperthermia combined with hepatic embolization using dextran magnetite-incorporated microspheres in rats. Int J Oncol 2000; 16: 1153–1158
- Jones SK, Winter JG, Gray BN. Treatment of experimental rabbit liver tumours by selectively targeted hyperthermia. Int J Hyperthermia 2002; 18: 117–128
- Granov AM, Tiutin LA, Tarazov PG, Granov DA. [Modern technologies of diagnosis and combined surgical treatment in liver tumors.]. Vestn Ross Akad Med Nauk 2003; 00: 51–54
- Jordan A, Wust P, Fahling H, John W, Hinz A, Felix R. Inductive heating of ferrimagnetic particles and magnetic fluids: Physical evaluation of their potential for hyperthermia. Int J Hyperthermia 1993; 9: 51–68
- Jordan A, Scholz R, Maier-Hauff K, van Landeghem FK, Waldoefner N, Teichgraeber U, Pinkernelle J, Bruhn H, Neumann F, Thiesen B, et al. The effect of thermotherapy using magnetic nanoparticles on rat malignant glioma. J Neurooncol 2006; 78: 7–14
- Johannsen M, Thiesen B, Jordan A, Taymoorian K, Gneveckow U, Waldofner N, Scholz R, Koch M, Lein M, Jung K, et al. Magnetic fluid hyperthermia (MFH) reduces prostate cancer growth in the orthotopic Dunning R3327 rat model. Prostate 2005; 64: 283–292
- Johannsen M, Thiesen B, Gneveckow U, Taymoorian K, Waldöfner N, Scholz R, Deger S, Jung K, Loening S, Jordan A. Thermotherapy using magnetic nanoparticles combined with external radiation in an orthotopic Dunning rat model of prostate cancer. Prostate 2005, in press
- Gneveckow U, Jordan A, Scholz R, Bruess V, Waldoefner N, Ricke J, Feussner A, Hildebrandt B, Rau B, Wust P. Description and characterization of the novel hyperthermia- and thermoablation-system MFH 300F for clinical magnetic fluid hyperthermia. Med Phys 2004; 31: 1444–1451
- Sreenivasa G, Gellermann J, Rau B, Nadobny J, Schlag P, Deuflhard P, Felix R, Wust P. Clinical use of the hyperthermia treatment planning system hyperplan to predict effectiveness and toxicity. Int J Radiation Oncol Biol Phys 2003; 55: 407–419
- Sapareto SA, Dewey WC. Thermal dose determination in cancer therapy. Int J Radiation Oncol Biol Phys 1984; 10: 787–800
- Maier-Hauff K, Rothe R, Scholz R, Gneveckow U, Wust P, Thiesen B, Feussner A, von Deimling A, Waldoefner N, Felix R, et al. Intracranial thermotherapy using magnetic nanoparticles combined with external beam radiotherapy: Results of a feasibility study on patients with glioblastoma multiforme. J Neurooncol 2007; 81: 53–60
- Wust P, Gneveckow U, Johannsen M, Bohmer D, Henkel T, Kahmann F, Sehouli J, Felix R, Ricke J, Jordan A. Magnetic nanoparticles for interstitial thermotherapy–feasibility, tolerance and achieved temperatures. Int J Hyperthermia 2006; 22: 673–685
- Johannsen M, Gneveckow U, Eckelt L, Feussner A, Waldofner N, Scholz R, Deger S, Wust P, Loening SA, Jordan A. Clinical hyperthermia of prostate cancer using magnetic nanoparticles: Presentation of a new interstitial technique. Int J Hyperthermia 2005; 21: 637–647
- Johannsen M, Gneveckow U, Thiesen B, Taymoorian K, Cho CH, Waldofner N, Scholz R, Jordan A, Loening SA, Wust P. Thermotherapy of prostate cancer using magnetic nanoparticles: Feasibility, imaging, and three-dimensional temperature distribution. Eur Urol 2007; 52: 1653–1662
- Johannsen M, Gneveckow U, Taymoorian K, Thiesen B, Waldofner N, Scholz R, Jung K, Jordan A, Wust P, Loening SA. Morbidity and quality of life during thermotherapy using magnetic nanoparticles in locally recurrent prostate cancer: Results of a prospective phase I trial. Int J Hyperthermia 2007; 23: 315–323
- Anscher MS, Samulski TV, Dodge R, Prosnitz LR, Dewhirst MW. Combined external beam irradiation and external regional hyperthermia for locally advanced adenocarcinoma of the prostate. Int J Radiat Oncol Biol Phys 1997; 37: 1059–1065
- Van Vulpen M, De Leeuw AA, Raaymakers BW, Van Moorselaar RJ, Hofman P, Lagendijk JJ, Battermann JJ. Radiotherapy and hyperthermia in the treatment of patients with locally advanced prostate cancer: Preliminary results. BJU Int 2004; 93: 36–41
- Hurwitz MD, Kaplan ID, Hansen JL, Prokopios-Davos S, Topulos GP, Wishnow K, Manola J, Bornstein BA, Hynynen K. Association of rectal toxicity with thermal dose parameters in treatment of locally advanced prostate cancer with radiation and hyperthermia. Int J Radiat Oncol Biol Phys 2002; 53: 913–918
- Hartman KB, Wilson L J, Rosenblum MG. Detecting and treating cancer with nanotechnology. Mol Diagn Ther 2008; 12: 1–14