Abstract
Purpose: The aim of this study was to compare the performance of a microwave ablation (MWA) apparatus in preclinical and clinical settings.
Materials and method: The same commercial 2.45 GHz MWA apparatus was used throughout this study. In total 108 ablations at powers ranging from 20 to 130 W and lasting from 3 to 30 min were obtained on ex vivo bovine liver; 28 ablations at 60 W, 80 W and 100 W lasting 5 and 10 min were then obtained in an in vivo swine model. Finally, 32 hepatocellular carcinomas (HCCs) and 19 liver metastases in 46 patients were treated percutaneously by administering 60 W for either 5 or 10 min. The treatment outcome was characterised in terms of maximum longitudinal and transversal axis of the induced ablation zone.
Results: Ex vivo ablation volumes increased linearly with deposited energy (r2 = 0.97), with higher sphericity obtained at lower power for longer ablation times. Larger ablations were obtained on liver metastases compared to HCCs treated with 60 W for 10 min (p < 0.003), as ablation diameters were 4.1 ± 0.6 cm for metastases and 3.7 ± 0.3 cm for HCC, with an average sphericity index of 0.70 ± 0.04. The results on the in vivo swine model at 60 W were substantially smaller than the ex vivo and clinical results (either populations). No statistically significant difference was observed between ex vivo results at 60 W and HCC results (p > 0.08).
Conclusions: For the selected MW ablation device, ex vivo data on bovine liver was more predictive of the actual clinical performance on liver malignancies than an in vivo porcine model. Equivalent MW treatments yielded a significantly different response for HCC and metastases at higher deposited energy, suggesting that outcomes are not only device-specific but must also be characterised on a tissue-by-tissue basis.
Introduction
Percutaneous thermal tumour ablation is now being commonly used for the treatment of focal tumours in the liver, lung, kidney, and bone [Citation1–5]. Much of the initial literature, early basic research, and long-term clinical studies have been performed with radiofrequency (RF) energy-based systems [Citation1]. While RF ablation remains the most ubiquitous platform available in clinical use, it suffers from several significant limitations: relatively long treatment times, difficulties in creating uniform zones of tissue heating, and biophysical tissue interactions that ultimately limit RF heating (including tissue vaporisation and perfusion-mediated cooling) [Citation1].
Microwave (MW) thermal ablation platforms have been developed and investigated as alternatives to traditional RF-based systems [Citation6,Citation7]. Potential advantages of MW-based ablation include the ability to achieve higher temperatures (>100 °C) and larger ablation zones in shorter times, with less susceptibility to blood flow-induced heat sink effects [Citation7–9] and to impedance-driven performance variability compared to RF ablation. Recently, several commercial microwave ablation (MWA) platforms have become available [Citation10] with initial clinical studies having been published about their safety and efficacy [Citation6,Citation11–14]. Yet, these reports do not provide a comprehensive a priori algorithm for the determination of the ablation parameters given tumour features. Indeed, it must be acknowledged that physicians are typically compelled to base their choice of energy parameters for most types of ablation devices upon the results produced by a given platform in ex vivo tissues, such as bovine liver. Nevertheless, the accuracy of the relationship between ex vivo and clinical ablation results has been challenged [Citation15]. Indeed, many differences are known between the two scenarios, such as the presence of blood perfusion and different physical features of the tumour with respect to healthy tissue [Citation16].
Despite the fact that several MW platforms are commercially available and being used clinically, many of these have only undergone limited characterisation with ex vivo and in vivo animal testing. Here we perform a systematic characterisation of a commercial MW ablation apparatus, comparing ex vivo, in vivo, and clinical performance in two sets of patients, those with ablation-eligible primary hepatocellular carcinoma (HCC group) in cirrhotic livers, and those with liver metastases (MET group) in order to more precisely determine differences in ablation outcome among relevant tissues.
Materials and methods
Experimental overview
All studies were performed using a 2.45 GHz MW ablation platform (HS AMICA, HS Hospital Service, Rome, Italy), with maximum generator output of 140 W and a cooled mini-choked 14 gauge antenna [Citation17,Citation18]. Partial support of the study was provided by the ablation equipment manufacturer. Three investigators (C.A., S.C. and N.T.) are employees of HS Hospital Service. However, members of the study at the external participating institutions had complete and independent control of all portions of the study including data gathering, analysis, and manuscript preparation. For all animal studies, institutional Animal Care and Use Committee approval was obtained prior to any studies. Similarly, for all clinical studies, Institutional Review Board approval was obtained. The study was performed in three phases, which are outlined below ().
Phase I: characterisation in ex vivo bovine liver
Systematic characterisation of tissue ablation over a range of MW power and ablation time combinations was performed in ex vivo bovine liver, the most common tissue in which ablation laboratory trials are performed and reported [Citation10]. This consisted of a grid of six time points (3, 5, 10, 15, 20, and 30 min) and six power settings (20, 40, 60, 80, 100 and 130 W), providing the most used parameter combinations in the clinical practice. A total of 108 ablations were performed with three repetitions per power/time group, each on a piece of ex vivo bovine liver measuring at least 5 × 10 × 10 cm3. Baseline liver temperature was 17.4 ± 1.2 °C (minimum 11 °C, maximum 21 °C). The MW antenna was placed into the liver away from vessels and for at least 2 cm into the tissue.
Outcome variables included ablation zone length (i.e. the ablation long axis, along the MW probe shaft trajectory), diameter (i.e. the ablation short axis, perpendicularly to the MW probe), and sphericity index (i.e. ablation short to long axes ratio), measured as described below in the Pathological and imaging evaluation section.
Phase II: characterisation in in vivo porcine liver
Based upon the results obtained in phase I, six parameter combinations were selected for in vivo porcine liver studies, namely 60 W, 80 W and 100 W ablation power for both 5 and 10 min duration (six data points, 28 ablations). Twelve Yorkshire pigs weighing 80–100 kg were used. Selected data points were randomly assigned to each animal and liver location, with a maximum of three ablations performed per animal (two in the right lobe and one in the left lobe). The animals were anaesthetised with isoflurane (2%, Baxter, Deerfield, IL). The liver was exposed via laparotomy, and ultrasound (US) was used for antenna placement to avoid puncturing large vessels, using methodology previously reported [Citation19]. Animals were sacrificed immediately after MWA completion and their liver was eventually excised for the evaluation of the ablation zones as reported in the following. Outcome variables again included ablation zone length, diameter, and sphericity index.
Phase III: clinical ablation studies in primary and secondary liver tumours
Phase III of the study was performed at the two participating hospitals under the approval of their respective Institutional Review Boards. All patients underwent US-guided percutaneous MW ablation (MWA) at one of the two tertiary referral centres (General Hospital of Busto Arsizio, or San Gerardo Hospital, Monza Brianza, Italy). All patients enrolled for the study were considered amenable to percutaneous thermal ablation based upon previously reported guidelines [Citation20,Citation21]. Specifically, all selected patients met defined inclusion criteria for tumour ablation treatment (good performance status/ECOG ≤1), and were either determined to be unresectable by consensus of a multidisciplinary team, including a hepatobiliary surgeon, or refused surgery. Only centrally located hepatic tumours, so as to be able to evaluate the entire ablation margin, and those not previously subject to prior loco-regional treatments were considered for this study. MWA procedures not performed in compliance with predefined operative standards (e.g. requiring multiple probes and/or insertions and/or energy deliveries and/or power and time settings other than those fixed for each group) automatically dropped off the study.
The study population included only central tumours treated with one probe, in a single insertion and energy delivery – i.e. without overlapping ablations – for which specific MWA settings stemming from phase I and II were applied (namely 60 W for 10 min, and 60 W for 5 min). Outcome measures included evaluating length, diameter and sphericity index of the ablation zone on imaging (contrast-enhanced CT) performed 24 h after treatment.
Clinical percutaneous ablation procedures were performed by interventional radiologists with 15 years of experience (General Hospital of Busto Arsizio: L.S., T.I.; San Gerardo Hospital: M.F.M.). Written informed consent was obtained prior to treatment. Before treatment all patients underwent a contrast-enhanced multiphasic (non-contrast, arterial, portal, and delayed phase) CT study to enable evaluation by a multidisciplinary team to determine optimal management.
MWA was performed under general anaesthesia using real-time US guidance with contrast-enhancement (SonoVue, Bracco, Milan, Italy) to improve tumour visibility and targeting, and for immediate post-treatment assessment.
All the tumours were administered 60 ± 5 W for either 5 ± 1 min or 10 ± 1 min, with the end point based upon achievement of a satisfactory hyperechogenic region encompassing the tumour and the desired ablative margin. Best effort was always made at placing the MWA applicator in the centre of the tumour, with the tip at its distal margin or no more than a few millimetres beyond. Each tumour was treated with a single uninterrupted energy delivery. Immediate post-ablation imaging with contrast-enhanced US was performed in order to assess size and adequacy of ablation. Following MWA, all patients were hospitalised for 1–2 days for observation and within 24 h they underwent multiphasic contrast-enhanced CT for the final assessment of the ablation achieved and the detection of possible complications.
Pathological and imaging evaluation
For ex vivo experiments the specimens were sectioned along the antenna insertion track immediately at the conclusion of the power delivery in order to expose the plane including the ablation maximum longitudinal and transversal dimensions to visual inspection. Ablation length and diameter were determined at gross pathological examination according to standard criteria for the coagulation zone [Citation5], using digital calipers. The accuracy of this method, determined as the discrepancy between measurements by three independent observers (S.N.G., S.C., and C.A.), was 1 mm.
For in vivo animal experiments the specimens were harvested and sectioned parallel to the antenna, and the length and diameter of each ablation were measured with the consensus of two observers (N.T. and S.N.G.). Antenna orientation was documented prior to procedure, thus taking into account axis orientation during gross analysis. Additionally, multiple sections were taken to ensure optimal measurement.
For clinical cases, length (along the MW antenna) and diameter (perpendicular to the antenna) of the ablation zone were independently measured retrospectively in blind fashion based upon reconstruction of the needle path on the 24-h post-treatment CT scans in the portal phase. All measurements were performed by individuals blinded to the treatment group (M.A., S.N.G.). The volume (V) of the ablation zones was estimated as that of an ellipsoid of revolution according to the formula V = L·D2·π/6, where D and L are the ablation zone diameter (short axis) and length (long axis).
Statistical analysis
The dimensions for each experimental population were characterised from its average, standard deviation, and coefficient of variation. Using multivariate ANOVA, the diameter, length, volume and sphericity of each ablation were compared among groups. Multivariate ANOVA was used in analysing the effects of the applied power and the treatment duration of the ablation. Groups were compared and significance established using a two-tailed heteroscedastic t-test (p < 0.05). Linear best fits were obtained through the least squares method and the correlation between data sets was calculated through the Pearson product-moment correlation coefficient.
Results
Phase I: characterisation in ex vivo bovine liver
Ablation zone parameters (length, diameter, and sphericity index) for different MW ablation settings (power 20–130 W, time 3–30 min) are provided in . For a set ablation time, increasing power increased both ablation zone length and diameter (p < 0.05, all comparisons). Similarly, for a set MW power, increasing time resulted in a larger ablation length and diameter (p < 0.05, all comparisons). Of the ablation length observed at 30 min, 80% was already achieved after 15 min of treatment at almost all power settings (20–100 W), and after 10 min for the highest power setting (130W, ).
Table 1. Microwave ablation zone sizes in ex vivo bovine liver for different combinations of treatment power and duration.
Similarly sized ablation zones were achieved at varying power and time settings (for example, ablation zone length was 5.1–5.4 cm for 100 W × 3 min, 80 W × 5 min, 60 W × 10 min, and 40 W × 15 min). However, lower powers with longer ablation times generally resulted in a greater sphericity index compared to the highest power for shortest time. For example, ablation at 40 W for 30 min resulted in a sphericity index of 0.9 compared to 0.6 at 130 W for 3 min for similarly sized ablation zones (6.1 ± 0.4 cm × 5.2 ± 0.1 cm at 40 W compared to 5.6 ± 0.2 cm × 3.6 ± 0.3 cm at 130 W).
The ablation volume increased linearly (r2 = 0.97) with the energy deposited in the tissues, with a coefficient of approximately 1.3 cm3/kJ (). The increase with deposited energy of the two linear ablation dimensions was fitted with a cubic root (average r2 = 0.98 ± 2). Hence, for doubling either the ablation length or diameter the deposited energy must be increased by approximately eight times.
Figure 2. Ex vivo ablation diameter (upper graph) and sphericity (lower graph) for different power settings and ablation times. The lines in Figure 2a represent the best cubic root fit of the data. The lines in Figure 2b are guides for the eye.
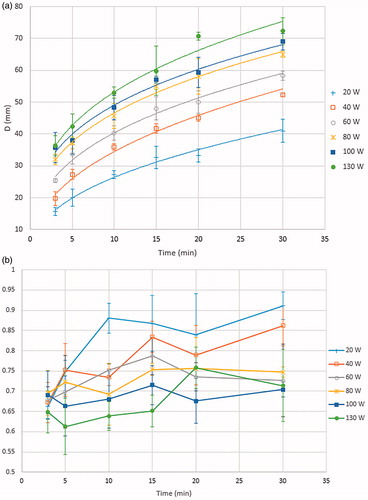
Figure 3. Ex vivo ablation volume as a function of the deposited energy. The black line is the best linear fit.
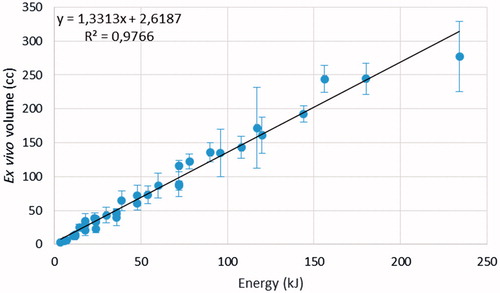
Phase II: characterisation in in vivo porcine liver
Increasing the ablation power and/or time resulted in a significant (p < 0.05) increase of the ablation volume () for all comparisons, excepting that of between 80 W and 100 W at 5 min (p = 0.25), a finding attributed to the large uncertainty affecting the volume determination at 100 W at 5 min. The average ablation length in the porcine model was 101% ± 13% (p = 0.85) of the ex vivo ablation length, while the average diameter was 87 ± 11% (p = 0.03) of its ex vivo counterpart. Consequently, the average ablation volume and sphericity in the porcine model respectively reduced to 78% and 86% compared to ex vivo. Similarly to the ex vivo findings, the in vivo ablation volume also showed an increase with deposited energy, though in a non-linear fashion (r2 = 0.74).
Table 2. Microwave ablation zone sizes for different treatment power and time settings in in vivo porcine liver.
Phase III: clinical ablation studies in primary and secondary liver tumours
From May 2010 to July 2011 a total of 46 patients were enrolled (29 men, 17 women, age 70 ± 10 years, min 43 years, max 84 years) with 51 liver tumours (size 0.8–4.2 cm, 2.3 ± 0.8 cm maximum diameter). The HCC group was composed of a total of 30 patients with 32 tumours of size 2.4 ± 0.7 cm. The MET group was composed of a total of 16 patients with 19 tumours of size 2.2 ± 0.7 cm (p = 0.2), of which 13 were from colorectal carcinoma and one each from breast, thyroid, lung, pancreas and small intestine cancers).
Complete ablation was obtained on 48/51 tumours (94.1%). The overall average ablation length and diameter obtained in the group treated with 60 W for 5 min were, respectively, 48 ± 7 mm and 33 ± 5 mm for HCC and 55 ± 17 mm and 40 ± 9 mm for metastases (). For treatments performed at 60 W for 10 min the ablation length and diameter respectively increased to 52 ± 6 mm and 37 ± 3 mm for HCC and to 65 ± 9 mm and 41 ± 6 mm for metastases. For both HCC and metastasis ablation, increasing the ablation time from 5 min to 10 min did not achieve statistically significant increase of either ablation length or diameter (p > 0.05). Whereas there was no significant difference between the outcomes on HCC and MET groups for 5-min ablations, the two tumour types showed a significant difference in outcome for 10-min ablations for both diameter and length (p < 0.02 and p < 0.0003 respectively). Additionally, on average the ablation short axis exceeded the tumour size by 1.1 ± 0.9 cm in the HCC group and by 1.9 ± 0.7 cm in the MET group (p < 0.002). Finally, no statistical difference was found on the sphericity among all the investigated groups, with an overall average sphericity index of S = 0.7 ± 0.1 (p > 0.05 for all comparisons). The overall average coefficient of variation (CV), defined as the ratio between the standard deviation and the mean, was 0.12 for HCC and 0.20 for MET.
Table 3. Average microwave ablation zone sizes in patients with HCC or liver metastases treated at 60 W for either 5 or 10 min.
Clinical findings are in agreement with the corresponding ex vivo results within 8% for HCC and 20% for MET (), with the difference being significant only for MET treated for 10 min. By contrast, the in vivo porcine results systematically underestimated the clinical results, the difference being significant for the majority of comparisons.
Table 4. Percentage variations of the ablation volume V, sphericity S, diameter D and length L of the three in vivo (IN) experiments compared to ex vivo (EX) results. Each percentage variation was calculated as 100×(IN-EX)/EX. Below each percentage variation the corresponding p-value is reported to assess the significance of the variation.
Discussion
MW-based thermal ablation systems are being developed to address limitations in existing ablation systems, including difficulties of RF in ablating larger tumours, the need for longer ablation times, and the need to use multiple ablation probes. Yet limited data exist regarding characterisation of tissue energy interactions, particularly for different types of tissue.
Ex vivo results
Our results confirm that in ex vivo tissues, where there is no blood perfusion, the ablation volume (V) depends most on the overall deposited energy (Ed) and not on the individual power and time values. This implies that it is necessary to deposit in the tissue a given amount of energy to achieve thermal coagulation of a given tissue volume. The amount of energy required per unit coagulation volume (ɛ) might vary according to the properties of the tissue (e.g. specific heat, electrical conductivity and water content), of the energy source (e.g. operating frequency, delivery algorithm) and of the applicator (e.g. the MW radiation pattern). In this study, the value ɛ = 0.77 kJ/cm3 was found. Since the ablation volume is proportional to the product of the ablation length × the square ablation diameter, both must be proportional to the cubic root of Ed, as indeed was verified through best fitting.
The energy deposited in the tissues causes a temperature increase around the antenna according to the spatial distribution of the emitted MW field [Citation18]: this is usually referred to as active heating (i.e. directly due to the energy source in use) [Citation22]. The active heating induces a temperature increase proportional to Ed. The fact that V was also found to be proportional to Ed suggests that all other heating phenomena contributing to the ablation process (e.g. passive heating) are either negligible or directly proportional to active heating itself.
Unlike V, the ablation sphericity is actually affected by the individual power and time settings used to deposit a certain energy. Faster (i.e. high power) depositions consistently result in less spherical ablations. On the other side, however, slower (i.e. low power) depositions are more likely affected by blood perfusion and heat sinks in in vivo applications [Citation23]. Delivering MW energy in vivo at intermediate power and time settings (60 W for 5 or 10 min) may therefore supply the best trade-off between ablation volume and sphericity.
In vivo porcine liver
The ablation zones observed in porcine liver were significantly (p < 0.05) smaller and less spherical than those observed in almost every other set-up, with isolated exceptions possibly caused by experimental errors.
The reduced ablation volume is likely attributable to the appreciably higher perfusion in the animal model compared to hypovascular hepatic tumours. The tissue variability could also induce an increased tissue contraction due to the positive correlation between the latter and the tissue water content [Citation24–25]. Moreover, it has been previously demonstrated in ex vivo bovine liver [Citation26] that MW ablation sphericity is altered when the thickness of the specimen is close to the ablation diameter obtained in unrestricted tissue for a given power/time combination, due to the heavily modified thermal and electromagnetic boundary conditions. Indeed, the limited porcine liver lobe thickness may well account for the smaller ablation sphericity observed in our animal model.
Clinical cases
On average, similar tumour sizes treated with the same 60 W/10 min protocol produced overall larger ablation as well as a larger ablative margin for the MET group (1.0 cm) than for the HCC group (0.6 cm), complying with the most commonly accepted guidelines. This is particularly innovative for the treatment of liver metastases, where RFA has proved to be less effective than for HCC on a size per size basis [Citation27]. Clearly, more study defining the appropriateness of the traditional 3-cm diameter cut-off used for RF ablation [Citation28] is warranted.
The larger ablated volume obtained for metastases compared to HCCs could potentially be ascribed to the reduced water content of HCCs and the surrounding cirrhotic liver, causing a lower absorption of the emitted MW field by target tissues, and therefore a less effective active heating. The better sphericity of the HCC ablation could instead be due to the capsulation of HCC tumours that is not present in metastases [Citation29,Citation30].
Clinical cases’ outcomes also compared favourably with ex vivo data, as significant differences (p < 0.05) were limited to the MET group treated with 60 W for 10 min, where an increased ablation length and a consequently reduced sphericity were found. Since none of the other comparisons yielded significant variations, it might be concluded that, at least limited to the explored power/time settings, the ex vivo model is fairly predictive of clinical outcomes, and certainly more accurate than the ablation of in vivo porcine liver. This is different from the traditional ablation paradigm based on initial experience with RF ablation, where clinical ablation zones are smaller than those found during the preliminary characterisation in ex vivo and in vivo models [Citation31].
The coefficient of variation of clinical cases was far larger than what was found in ex vivo experiments at the same power and time (CV =0.06). This difference attests an increased outcome uncertainty in clinical cases, particularly for metastases. Besides the intrinsic variability of the tissues physical properties (e.g. permittivity, water content and thermal conductivity), possible sources of ablation performance variability might include the proximity of the target tumours to the liver capsule or to large blood vessels, the clinical history of the patient or the presence of co-morbidities. Regardless, the large ablation volumes achieved in clinical treatments combined with their variability suggest that great care needs to be taken with early clinical applications to ensure patient safety. Indeed, complications and difficulty controlling ablation size have been reported with MWA systems in early applications [Citation32,Citation33].
The lack of statistically significant differences between tumours treated at 60 W for 5 and 10 min might be the result of the fact that blood perfusion dissipates an increasing amount of heat as the ablation zone surface expands over time. However, the average ablation lengths and diameters are in some case clinically relevant and the lack of statistically significant differences could be due just to the high coefficients of variation. A larger study over a better stratified population could improve the understanding of this phenomenon.
General remarks
Whereas for RFA in vivo porcine characterisation proved to be a useful ‘worst case scenario’ to predict clinical performances, the same seems no longer valid for MWA. Such difference may be due to the larger ablation extension and the larger amount of vaporisation induced by MWA with respect to RFA. In vivo porcine MWA characterisation was indeed found to be so far off as to question its utility for newer MWA devices characterisation. Ex vivo coagulation data appear to be way more representative of the actual MWA outcome in the treatment of HCCs than the animal model. This could be caused by the predominance in MWA of active heating. Active heating is barely affected by blood perfusion, which is the main source of differences between ex vivo and clinical outcomes of RFA [Citation34,Citation35]. Complete device characterisation in a three-tiered manner is currently recommended for assessing all new devices and ablation platforms, and holds substantial promise for more rapid regulatory approval and robust clinical use. These data underscore the fact that general principles (such as the one where ablation volumes tend to be smaller with ex vivo studies) are not universally applicable. In this respect it is particularly relevant that the complete characterisation of the ablation device on the in vivo animal model was found to have little practical benefits in terms of predicting the device clinical performance. If confirmed, this could impact on the characterisation protocols required for regulatory purposes.
Limitations
The results presented here are device-specific, and there is no long-term follow-up of clinical cases. Further investigations should attempt at refining the characterisation focus for this MWA platform by providing more precise target selection criteria and accordingly larger patient and tumour numbers for sustaining increased data stratification. Additionally a larger range of power/time parameters needs to be studied in clinics, as the in vivo data suggest that power has a greater influence on ultimate ablation outcome compared to time. Finally, as our study has shown tissue-specific differences under some conditions, further study in multiple organs and tumour types is clearly warranted.
Conclusion
The investigated MWA system was capable of generating large, consistent and reproducible ablation zones, whose volume scaled linearly with deposited energy in ex vivo bovine liver. Lower power and longer treatment times resulted in more spherical ablations. Ex vivo ablations demonstrated better and reasonably accurate correspondence with their clinical counterparts compared to the in vivo animal model, a finding which should be taken into due account when providing guidelines as to the use of new ablation platforms based on preclinical data. Finally, HCC and hepatic metastases show a different response to equivalent high energy ablation treatments. Accordingly, further investigation is warranted in order to provide optimised ablation protocols specific for each target tissue and pathology.
Acknowledgements
L.S. and T.I. are currently affiliated to the Department of Radiology, Humanitas University and Research Hospital, Rozzano, Milan, Italy, and M.F.M. is currently affiliated to the Radiology Department, Interventional Ultrasound, Casa di Cura Igea, Milan, Italy.
Disclosure statement
C.A., S.C. and N.T. are employees of H.S. Hospital Service SpA, the company manufacturing the MWA apparatus used in the study. L.S. is a consultant of H.S. Hospital Service SpA, S.N.G. is a consultant of Angiodynamics and Cosman Medical Inc. for unrelated development of IReversible Electroporation (IRE) and RF devices respectively. All other authors have no known conflicts to report. The authors alone are responsible for the content and writing of the paper.
References
- Ahmed M, Brace CL, Lee FTJ, Goldberg SN. Principles of and advances in percutaneous ablation. Radiology 2011;258:351–69.
- Gillams A, Goldberg SN, Ahmed M, Bale R, Breen D, Callstrom M, et al. Thermal ablation of colorectal liver metastases: a position paper by an international panel of ablation experts, the Interventional Oncology Sans Frontières meeting 2013. Eur Radiol 2012;25:3438–54.
- Seror O. Ablative therapies: advantages and disadvantages of radiofrequency, cryotherapy, microwave and electroporation methods, or how to choose the right method for an individual patient? Diagn Interv Imaging 2015;96:617–24.
- Wells SA, Hinshaw JL, Lubner MG, Ziemlewicz TJ, Brace CL, Lee FTJ. Liver ablation: best practice. Radiol Clin North Am 2015;53:933–71.
- Ahmed M. Image-guided tumor ablation: standardization of terminology and reporting criteria – a 10-year update. Radiology 2014;273:241–60.
- Liu Y, Li S, Wan X, Li Y, Li B, Zhang Y, et al. Efficacy and safety of thermal ablation in patients with liver metastases. Eur J Gastroenetrol Hepatol 2013;25:442–6.
- Lubner MG, Brace CL, Hinshaw JL Lee FTJ. Microwave tumor ablation: mechanism of action, clinical results and devices. J Vasc Interv Radiol 2010;21:S192–S203.
- Andreano A, Brace CL. A comparison of direct heating during radiofrequency and microwave ablation in ex vivo liver. Cardiovasc Intervent Radiol 2013;36:505–11.
- Di Vece F, Tombesi P, Ermilli F, Maraldi C, Sartori S. Coagulation areas produced by cool-tip radiofrequency ablation and microwave ablation using a device to decrease back-heating effects: a prospective pilot study. Cardiovasc Intervent Radiol 2014;37:723–9.
- Hoffmann R, Rempp H, Erhard L, Blumenstock G, Pereira PL, Claussen CD, Clasen S. Comparison of four microwave ablation devices: an experimental study in ex vivo bovine liver. Radiology 2013;268:89–97.
- Livraghi T, Meloni F, Solbiati L, Zanus G. Complications of microwave ablation for liver tumors: results of a multicenter study. Cardiovasc Intervent Radiol 2012;35:868–74.
- Poggi G, Montagna B, Di Cesare P, Riva G, Bernardo G, Mazzucco M, Riccardi A. Microwave ablation of hepatocellular carcinoma using a new percutaneous device: preliminary results. Anticancer Res 2013;33:1221–7.
- Horn JC, Patel RS, Kim E, Nowakowsky FS, Lookstein RA, Fischman AM. Percutaneous microwave ablation of renal tumors using a gas-cooled 2.4-GHz probe: technique and initial results. J Vasc Interv Radiol 2014;25:448–53.
- Ziemlewicz TJ, Hinshaw JL, Lubner MG, Brace CL, Alexander ML, Agarwal P, Lee FTJ. Percutaneous microwave ablation of hepatocellular carcinoma with a gas-cooled system: initial clinical results with 107 tumors. J Vasc Intervent Radiol 2015;26:62–8.
- Winokur RS, Du JY, Pua BB, Talenfeld AD, Sista AK, Schiffman MA, et al. Characterization of in vivo ablation zones following percutaneous microwave ablation of the liver with two commercially available devices: are manufacturer published reference values useful? J Vasc Interv Radiol 2014;25:1939–46.
- Rossmann C, Haemmerich D. Review of temperature dependence of thermal properties, dielectric properties, and perfusion of biological tissues at hyperthermic and ablation temperatures. Crit Rev Biomed Eng 2014;42:467–92.
- Longo I, Biffi Gentili G, Cerretelli M, Tosoratti N. A coaxial antenna with miniaturized choke for minimally invasive interstitial heating. IEEE Trans Biomed Eng 2003;50:82–8.
- Cavagnaro M, Amabile C, Bernardi P, Pisa S, Tosoratti N. A minimally invasive antenna for microwave ablation therapies: design, performances and experimental assessment. IEEE Trans Biomed Eng 2011;58:949–59.
- Hines-Peralta A, Pirani N, Clegg P, Cronin N, Ryan T, Liu Z, Goldberg S. Microwave ablation: results with a 2.45-GHz applicator in ex vivo bovine and in vivo porcine liver. Radiology 2006;239:94–102.
- Mazzaferro V, Lencioni R, Majno P. Early hepatocellular carcinoma on the procrustean bed of ablation, resection, and transplantation. Semin Liver Dis 2014;34:415–26.
- Lee H, Heo J, Cho Y, Yun S, Kim H, Lee W. Hepatectomy vs radiofrequency ablation for colorectal liver metastasis: a propensity score analysis. World J Gastroenterol 2015;21:3300–7.
- Schramm W, Yang D, Haemmerich D. Contribution of direct heating, thermal conduction and perfusion during radiofrequency and microwave ablation. Conf Proc IEEE Eng Med Biol Soc 2006;1:5013–16.
- Ringe K, Lutat C, Rieder C, Schenk A, Wacker F, Raatschen H. Experimental evaluation of the heat sink effect in hepatic microwave ablation. PLoS ONE 2015;10: e0134301.
- Liu D, Brace CL. CT imaging during microwave ablation: analysis of spatial and temporal tissue contraction. Med Phys 2014;41:113303.
- Sommer CM, Sommer SA, Mokry T, Gockner T, Gnutzmann D, Belleman N, et al. Quantification of tissue shrinkage and dehydration caused by microwave ablation: experimental study in kidneys for the estimation of effective coagulation volume. J Vasc Interv Radiol 2013;24:1241–8.
- Cavagnaro M, Amabile C, Cassarino S, Tosoratti N, Pinto R, Lopresto V. Influence of the target tissue size on the shape of ex vivo microwave ablation zones. Int J Hyperthermia 2015;31:48–57.
- Solbiati L, Livraghi T, Goldberg S, Ierace T, Meloni M, Dellanoce M, et al. Percutaneous radio-frequency ablation of hepatic metastases from colorectal cancer: long-term results in 117 patients. Radiology 2001;221:159–66.
- Livraghi T. Single HCC smaller than 2 cm: surgery or ablation: interventional oncologist’s perspective. J Hepatobiliary Pancreat Sci 2010;17:425–9.
- Liu Z, Ahmed M, Weinstein Y, Yi M, Mahajan R, Goldberg S. Characterization of the RF ablation-induced 'oven effect': the importance of background tissue thermal conductivity on tissue heating. Int J Hyperthermia 2006;22:327–42.
- Rossi S, Gallati M, Rosa L, Marini A, Viera F, Maestri M, Dionigi P. Effect of hyperbarism on radiofrequency ablation outcome. Am J Roentgenol 2007;189:876–82.
- Cha J, Choi D, Lee M, Rhim H, Kim Y, Lim H, et al. Radiofrequency ablation zones in ex vivo bovine and in vivo porcine livers: comparison of the use of internally cooled electrodes and internally cooled wet electrodes. Cardiovasc Intervent Radiol 2009;32:1235–40.
- Mitsuzaki K, Yamashita Y, Nishiharu T, Sumi S, Matsukawa T, Takahashi M, et al. CT appearance of hepatic tumors after microwave coagulation therapy. Am J Roentgenol 1998;171:1397–403.
- Hompes R, Fieuws S, Aerts R, Thijs M, Penninckx F, Topal B. Results of single-probe microwave ablation of metastatic liver cancer. Eur J Surg Oncol 2010;36:725–30.
- Goldberg SN, Hahn PF, Tanabe KK, Mueller PR, Schima W, Athanasoulis CA. Percutaneous radiofrequency tissue ablation: does perfusion-mediated tissue cooling limit coagulation necrosis? J Vasc Interv Radiol 1998;9:101–11.
- Ahmed M, Liu Z, Humphries S, Goldberg SN. Computer modeling of the combined effects of perfusion, electrical conductivity, and thermal conductivity on tissue heating patterns in radiofrequency tumor ablation. Int J Hyperthermia 2008;24:577–88.