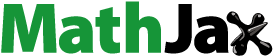
Abstract
Background: The use of magnetic resonance imaging-guided high-intensity focused ultrasound (MR-HIFU) to deliver mild hyperthermia requires stable temperature mapping for long durations. This study evaluates the effects of respiratory motion on MR thermometry precision in pediatric subjects and determines the in vivo feasibility of circumventing breathing-related motion artifacts by delivering MR thermometry-controlled HIFU mild hyperthermia during repeated forced breath holds.
Materials and methods: Clinical and preclinical studies were conducted. Clinical studies were conducted without breath-holds. In phantoms, breathing motion was simulated by moving an aluminum block towards the phantom along a sinusoidal trajectory using an MR-compatible motion platform. In vivo experiments were performed in ventilated pigs. MR thermometry accuracy and stability were evaluated.
Results: Clinical data confirmed acceptable MR thermometry accuracy (0.12–0.44 °C) in extremity tumors, but not in the tumors in the chest/spine and pelvis. In phantom studies, MR thermometry accuracy and stability improved to 0.37 ± 0.08 and 0.55 ± 0.18 °C during simulated breath-holds. In vivo MR thermometry accuracy and stability in porcine back muscle improved to 0.64 ± 0.22 and 0.71 ± 0.25 °C during breath-holds. MR-HIFU hyperthermia delivered during intermittent forced breath holds over 10 min duration heated an 18-mm diameter target region above 41 °C for 10.0 ± 1.0 min, without significant overheating. For a 10-min mild hyperthermia treatment, an optimal treatment effect (TIR > 9 min) could be achieved when combining 36–60 s periods of forced apnea with 60–155.5 s free-breathing.
Conclusion: MR-HIFU delivery during forced breath holds enables stable control of mild hyperthermia in targets adjacent to moving anatomical structures.
Introduction
Mild hyperthermia (localized tissue heating in the range of 40–45 °C for durations of 10–90 min) has improved outcomes in multiple clinical trials when combined with radiation and/or chemotherapy for the treatment of cancer [Citation1–5]. Hyperthermia sensitizes tumor cells to radiotherapy by inhibiting DNA damage repair, and reduces the radio-resistance of hypoxic cells by increasing tumor perfusion and oxygenation [Citation6]. Combining mild hyperthermia with certain chemotherapy agents increases their cytotoxic effect [Citation7,Citation8], and localized heating can be used for physical targeting of drug delivery [Citation9]. Hyperthermia is also a potent stimulator of both the innate and adaptive immune systems [Citation10–13], which may increase the likelihood of response to immune checkpoint inhibitors, or trigger an abscopal effect. Thus, mild hyperthermia continues to be an important therapy modality for enhancing the effects of other cancer therapies.
The clinical utilization of hyperthermia has been limited by technical challenges in delivering and monitoring heat deposition in large targets deep within the body [Citation14–16]. Since hyperthermia is an adjuvant to a patient’s primary treatment, typically delivered repeatedly within 1–2 h of each fraction or dose of the primary treatment, it is desirable to have noninvasive hyperthermia delivery and monitoring. Electromagnetic (EM) and ultrasound (US) hyperthermia are both capable of noninvasive energy delivery into the body; US is capable of focusing energy into millimeter to centimeter-sized regions deep beneath the skin. Earlier generations of hyperthermia devices relied upon arrays of invasive thermocouple or optical temperature sensors inserted percutaneously into the tumor and surrounding tissue in order to monitor and control therapy delivery [Citation15]. Recently, magnetic resonance imaging-based thermometry (MRT) has been combined with EM [Citation17,Citation18] and high intensity focused ultrasound (HIFU) [Citation19–21] energy deposition for noninvasive hyperthermia monitoring. Due to the combination of precisely controlled extracorporeal energy deposition and real-time noninvasive three-dimensional temperature monitoring, MR-guided HIFU (MR-HIFU) is an especially promising method for delivery of mild hyperthermia. A commercial MR-HIFU hyperthermia system [Citation22,Citation23] is being investigated in Phase I trials of hyperthermia combined with radiotherapy for recurrent rectal cancer (NCT02528175).
A major technical challenge for MR-HIFU and MR-guided thermal therapy in general is the motion sensitivity of proton resonance frequency shift – based MR thermometry. The technique uses a subtraction of phase images acquired before and during therapy to detect minute magnetic field variations, which correspond linearly to temperature change at a rate of 10 parts per billion per degree Celsius [Citation24,Citation25]. However, small amounts of tissue motion anywhere within the MRI bore disrupt the background magnetic field due to variations in magnetic susceptibility, which can create time-varying magnetic field variations many times larger than those induced by temperature. This effect is a major contributor to the limited applicability of MR-HIFU in the treatment of abdominal targets [Citation26–28].
In the ablation of liver, pancreas, and kidney tumors, HIFU has been used with breath holds, heating one focal spot over less than 30 s during each breath hold [Citation29–32]. In hyperthermia, effective adjuvant therapy requires tens of minutes of thermal exposure at precisely maintained temperatures in the range of 40–45 °C, far too long for an individual breath hold. However, in some clinical situations, notably pediatric diagnostic MR imaging, in which young patients are routinely anesthetized for imaging, repeated forced breath holds of 1–2 min duration are routinely performed over total durations of up to an hour or more [Citation33].
In our study, we first conducted a clinical trial to evaluate the accuracy and precision of MR thermometry in pediatric patients with solid tumors, without applying breath-holds. We found poor temporal stability in tumors of the chest/spine, while temperature stability was adequate in extremity tumors. Based on these observations, we hypothesized that breath-hold hyperthermia, consisting of repeated cycles of ventilation followed by breath holds (forced apnea) during which heating is applied could improve thermometry quality and expand the scope of hyperthermia target ability to tumors of the chest and abdomen. Thus, we investigated the use of repeated forced breath holds in phantom and in vivo models as a means of eliminating the effects of motion on MR-HIFU hyperthermia treatment delivery. This technique could provide a simple and robust means of delivering MR-HIFU hyperthermia to selected abdominal and chest wall targets, greatly expanding the eligible patient population.
Materials and methods
Clinical trial
In order to evaluate the effect of anatomic location on the quality of MR thermometry in patients with cancer, children (n = 7) with a known or suspected diagnosis of solid malignancy who were scheduled to undergo a routine clinical MRI, with or without sedation, were enrolled. All patients and/or the legal guardians signed written informed consent and this study was approved by the institutional review board (IRB) at UT Southwestern Medical Center (IRB Number: STU 052014-023). At the completion of the patient’s clinical MRI scan (Achieva 1.5 T, Philips Healthcare, Best, The Netherlands), MR thermography of their tumor was acquired for 2–6 min using a segmented fast field echo echo-planar imaging (FFE-EPI) sequence with water-selective excitation (TE = 16 ms, TR = 38 ms, resolution = 160 × 160, voxel size = 2.5 × 2.5 × 7 mm, time per dynamic = 3.6 s, number of slices = 6) [Citation34]. Temperature maps were calculated offline by the manufacturer of the MR-HIFU system used in the subsequent laboratory experiments. No heating was applied during the imaging, so any changes in measured temperature were assumed to be artifact. Within a 10 mm region of interest centered in the tumor, MR thermometry accuracy (mean temperature change from baseline), uniformity (spatial standard deviation of temperature within a region of interest centered in the tumor), and stability (temporal standard deviation of the mean temperature within this region of interest across each dynamic image) were evaluated.
Pre-clinical study design
We evaluated the accuracy and stability of MR thermometry and investigated the effect of repeated motion initiation and cessation on MR thermometry quality and heating performance in two laboratory studies. The first laboratory study used a tissue-mimicking phantom instrumented with fiber-optic temperature sensors, where MR thermometry artifacts were introduced by computer-controlled motion of a metal object several centimeters away from the targeted region. The second validated this approach in an in vivo pig model, heating the longissimus dorsi muscles adjacent to moving organs of the abdomen.
MR-HIFU hyperthermia system
All laboratory experiments were performed on a 3 T MRI (Ingenia, Philips Healthcare, Best, The Netherlands) equipped with a clinical MR-HIFU system (Sonalleve V2, Profound Medical, Mississauga, Canada) utilizing research software designed for large-volume, long-duration mild hyperthermia under MR temperature control [Citation23]. After acquiring a set of T1-weighted planning images (T1-FFE, TE = 2 ms, TR = 4 ms, flip angle = 8°), an 18-mm diameter treatment cell was planned in the center of the 17 cm diameter phantom (). Temperature maps were acquired for 10–20 min using the same sequence as in the clinical imaging study.
Phantom study
A study was conducted with a cylindrical phantom (MR-HIFU QA Phantom, Philips Healthcare) and a programable MR-compatible motion platform capable of linear translation [Citation35]. An aluminum disk attached to the end of a plastic rod was mounted on the motion platform, in order to create a localized static magnetic field distortion (). Magnetic field changes associated with respiratory motion were simulated by moving the aluminum disk along a sinusoidal one-dimensional trajectory. The aluminum disk was set up near the same height as the target region to introduce large motion artifacts during MR thermometry acquisition. Breath holds were simulated by halting the motion at the smallest distance away from the phantom.
Figure 2. Experimental setup for phantom motion study. An MRI-compatible motion platform was used to create one-dimensional motion to simulate breathing artifacts during hyperthermia treatment. An aluminium disk was taped at the end of a plastic rod to introduce temperature artifacts (A). Reference images (1) for temperature mapping were acquired prior to the treatment with motion being held. HIFU started during first breath hold (2). Artifacts on temperature map were observed (3) when sinusoidal motion was introduced; HIFU was disabled during this period. Treatment resumed when motion was paused with HIFU enabled (4).

Prior to hyperthermia treatment, the stability of MR thermometry during motion with repeated breath holds was evaluated by collecting temperature maps for 10 min with HIFU disabled (n = 3). Subsequently, HIFU hyperthermia (1.2 MHz, 60 W, n = 3) using customized treatment control software [Citation23] was performed, with energy delivery enabled only during periods of simulated breath holds. Repeated breath holds were simulated by pausing motion for 60 s at the smallest distance to the phantom, and then resuming motion for 60 s. During the first breath hold, reference images were acquired for ∼20–25 s prior to regular temperature mapping with HIFU enabled. After the first breath hold, sinusoidal motion was introduced for 60 s to simulate free breathing, during which HIFU was disabled. The motion was then paused again for 60 s while HIFU was enabled during the simulated breath hold. This periodic gating was continued for 20 min, with 10 min of hyperthermia treatment followed by 10 min of post-treatment temperature mapping to observe the phantom cooling. The experimental work flow is described in .
Animal model
Animal experiments were approved by the Institutional Animal Care and Use Committee (IACUC) at the University of Texas Southwestern Medical Center. Two female Yorkshire pigs weighing 40–45 kg (Change of Pace, Aubrey, TX) were used in this study. The animals were intubated for general anesthesia with 2.0–3.5% isoflurane delivered by mechanical ventilation (10–15 mL/kg, 15–25 breaths per minute). A 22 G angiocatheter was placed in the marginal ear vein for the delivery of intravenous contrast agents. In one of the two pigs, vecuronium bromide (0.1 mg/kg) was injected every 20–30 min during the experiment as a neuromuscular blocking agent to eliminate any muscle contraction during forced breath holds. The back of each animal was carefully shaved, chemically depilated and cleaned to avoid air or skin defects which could interfere with ultrasound propagation. Heart rate and oxygen saturation were monitored with a MR-compatible physiological monitor (Nonin 8600 V with 10 m fiber-optic cable, Plymouth, MN) to ensure appropriate depth of anesthesia. A secondary MR-compatible physiological monitor (Philips Invivo Expressions, Orlando, FL) was used to measure respiration rate and end-tidal CO2 concentration. A temperature-controlled circulating water pump (Gaymar TP-500, Orchard Park, NY) was connected to a water blanket to maintain the core body temperature of the animal during the scan.
In-vivo experiments were performed on the same 3 T MRI and clinical MR-HIFU system as the phantom experiments described above. A 4-cm thick ultrasound gel pad (Aquasonic, Parker Laboratories, Fairfield, NJ) was placed on the acoustic window to allow ultrasound penetration and elevate the animal to an optimal height for targeting. Air bubbles between the skin and gel pad were removed carefully to avoid skin burns. Animals were placed supine on the MR-HIFU tabletop, providing an acoustic path from the ultrasound transducer into the thoracic longissimus dorsi back muscle. MR images were acquired using the five-element coil array of the MR-HIFU system. T1-weighted gradient echo images (T1-FFE, TE = 2 ms, TR = 4 ms, flip angle = 8°) were obtained first for treatment planning. An 18-mm treatment cell was placed in the back muscle. Temperature maps were acquired for 10–20 min with the same thermal sequence used in the phantom study. Temperature mapping acquisitions with HIFU disabled (n = 7) were performed to evaluate the stability and precision of MR thermometry.
Gated hyperthermia sonications under MR temperature control (1.2 MHz, 60 or 80 W, n = 5) were performed to evaluate the feasibility of breath-hold MR-HIFU hyperthermia treatment. During imaging, end expiration breath holds of 40–60 s were implemented by pausing the ventilator, during which the HIFU beam was enabled for hyperthermia treatment. A recovery respiration period of 40–60 s with HIFU disabled was performed after each forced breath hold. This process of gated hyperthermia continued for 10 min, after which another 10 min of imaging was acquired to monitor tissue cooling.
MR thermometry validation
MR thermometry was validated by fiber-optic temperature measurements in the phantom and in vivo. In the phantom study, same images were acquired for treatment planning (). Two fiber-optic temperature sensors (T1, Neoptix, Quebec, Canada) were inserted near the treatment cell to measure temperature change at the periphery of the heated region without being affected by the ultrasound beam (). Two additional fiber-optic sensors were inserted well away from the treatment cell to measure background temperature (). In the animal study, one fiber-optic sensor was inserted into the animal’s back muscle through a 19 G catheter at locations 2.0–2.5 cm away from the treatment cell to assess the accuracy and stability of MR thermometry. Another fiber-optic sensor was inserted into the animal’s rectum to record core body temperature.
After each sonication, MR thermometry accuracy was validated by calculating the absolute error between measurements made by fiber-optic temperature sensors and MR thermometry measurements from 10-mm diameter ROIs selected around the sensors (defined as the temporal mean value of Tmean – Tprobe). The averaged absolute error for each breath-hold session during the 10 min hyperthermia was calculated and plotted over time for all experiments to test the feasibility of a prolonged treatment using this proposed strategy. MR thermometry stability was evaluated as the temporal standard deviation of the spatial mean within the heated region during hyperthermia treatment.
Hyperthermia treatment evaluation
Breath-hold MR-HIFU hyperthermia treatment was performed for 10 min in the phantom and pig models. For analysis, an 18-mm diameter ROI was selected around the target region on the temperature maps to quantify the temperature change during the treatment. The target temperature within this region was selected to be 42.5 °C. A successful hyperthermia treatment was defined based on two criteria. First, the spatially-averaged temperature in the target ROI was maintained in the relevant hyperthermia range of 41–45 °C for the intended treatment duration, i.e., a time in range (TIR) of 10 min. Additionally, a uniform temperature distribution was achieved, i.e., median, 10th and 90th percentile of the temperature measurements within the target region were maintained at the target temperature. The TIR, then, is the sum of the time in which the ROI temperature was maintained at 41–45 °C. To obtain an estimate of the TIR across an entire hyperthermia treatment including a series of breath-holds and free-breathing periods, a temporally averaged temperature map calculated across the dynamics of each breath hold period was used to replace the motion-corrupted temperature maps of the following free breathing period. This sample-and-hold method has the advantage of using only the reliable measurements made during heating to give an estimate of TIR at all times, but may be biased towards higher TIR measurements when heating occurs faster than cooling. Hyperthermia temperature control was evaluated based on the temporal mean and standard deviation of the temperature in the target ROI.
Effect of breath-hold and free-breathing durations
An important parameter in the use of repeated forced breath holds in MR-HIFU hyperthermia treatment delivery is the duration of each breath-hold and free-breathing period. For optimal therapeutic effect, free-breathing periods should be short enough that target region temperature remains within the mild hyperthermia range (41–45 °C). Likewise, breath-hold periods should be long enough to re-heat the target region to the desired temperature using a safe HIFU power level. These durations are practically constrained in that each breath-hold period should start by collecting at least one set of motion-free temperature maps before applying power, as an uncorrupted input to the temperature control algorithm. Durations must also be clinically acceptable in terms of maintaining the anesthetized patient at safe blood oxygen saturation and end-tidal CO2 levels, and reducing overall treatment time.
To identify appropriate breath-hold and free-breathing durations, heating and cooling rates were estimated from phantom and in vivo MR-HIFU hyperthermia experiments, and used to model the overall treatment duration and heating quality for various breath-hold timing protocols. These parameters were modeled by making coarse approximations to the Pennes bioheat transfer equation, assuming equal density and specific heat
of tissue and blood (EquationEquation 1
(1)
(1) ).
(1)
(1)
is the temperature rise above the arterial temperature,
is time,
is thermal diffusivity (mm2/s),
is the blood perfusion rate (1/s), and
is the ultrasound-induced heating rate (°C/s).
During cooling () from an initial focal point temperature elevation
the temperature distribution perpendicular to the focus can be approximated analytically [Citation36–38] by assuming that the shape of HIFU-induced heating is Gaussian, with nominal lateral radius
of 9 mm, and negligible heat loss along the elongated longitudinal direction.
(2)
(2)
Using EquationEquation 2(2)
(2) , thermal diffusivity was estimated by calculating the Gaussian variance of the temperature distribution across the heated region at each MR thermometry time step during cooling, then calculating
from the slope of the Gaussian variance over time [Citation38]. For in vivo datasets, perfusion rate
was subsequently estimated using EquationEquation 2
(2)
(2) by fitting the temperature at the ultrasound focus (
) during cooling.
During heating, was estimated based on the initial temperature increase at the ultrasound focus in the first breath-hold period, using the coarse approximation that the effects of thermal diffusion and perfusion were small during the first 10–20 dynamics of heating (EquationEquation 3
(3)
(3) ) [Citation39].
(3)
(3)
Fitted heating and cooling rates in both phantom and in-vivo data were used to model temperature rise and fall at the focus, with the goal of identifying the shortest possible breath-hold durations and longest possible free-breathing durations that would still achieve effective MR-HIFU hyperthermia therapy. During the initial heating period, the minimum breath-hold period was estimated using EquationEquation 3(3)
(3) as the time required to heat the treated area from body temperature (assumed to be 37 °C) to the maximum allowed temperature during treatment (defined as 45 °C) as well as to the ideal treatment temperature (42 °C). After the temperature reaches 42 °C, minimum breath hold period was estimated as the time required to heat from the low end of treatment temperature (41 °C) to the high end of treatment temperature (44 °C). The maximum free-breathing period was estimated using EquationEquation 2
(2)
(2) as the time to cool the treated area from the maximum allowed temperature (45 °C) to the minimum acceptable temperature during treatment (defined as 41 °C). Different combinations of breath-hold and free-breathing periods were compared by their effective heating durations (TIR between 41 °C and 45 °C).
Statistical analysis
Results were presented as the mean and standard deviation unless otherwise specified. The differences in the absolute error and stability of MR thermometry during motion and no motion period were evaluated using Student t-test with Holm-sidak correction. Additional t-test was performed to evaluate the effect of heating on the absolute error and stability. To evaluate the trend of absolute error over time, one-way ANOVA with Tukey adjustment for multiple comparison was performed. p values <.05 were considered as statistically significant.
Results
MR thermometry validation in children with cancer
MR thermometry accuracy, uniformity, and stability in pediatric solid tumors are shown in and . No breath-hold was applied in this study. Children with extremity tumors (limbs) demonstrated acceptable thermometry accuracy, uniformity, and stability without sedation. Those parameters were worse in patients with tumors in the chest and spine despite sedation, consistent with their location near moving organs. Tumors in the pelvis were variable with one tumor in a sedated patient demonstrating good thermometry while the other, in an unsedated patient, demonstrated poor thermometry quality.
Figure 3. Evaluation of MR thermometry quality in children with tumours in different locations (with/without sedation). Cluster of points represent the mean temperature error (compared with baseline at body temperature) within a target ROI at different time points during the 5 minutes of imaging. The accuracy of MR thermometry was estimated by calculating the mean value of each cluster. The distribution of the points in each cluster indicated the temporal stability of MR thermometry.

Table 1. Evaluation of MR thermometry quality in patients with solid tumors in various locations.
MR thermometry validation in phantom and pigs
In order to model the thermometry variation observed in patients’ tumors near moving organs, temperature measurements were acquired in phantoms subjected to simulated motion artifacts with fiber-optic sensors and MR thermometry as shown in for experiments with HIFU enabled () and HIFU disabled (). Different colors were assigned to periods with (red) and without (blue) simulated motion artifacts. With HIFU enabled, the maximum error decreased from 2.1 ± 1.0 °C during motion periods to 0.9 ± 0.1 °C in no-motion periods. With HIFU disabled, the maximum error also decreased, from 2.9 ± 0.5 °C to 2.1 ± 0.3 °C in no-motion periods. The accuracy and stability of MR Thermometry improved significantly during no-motion periods compared to motion periods. During periods with no motion, absolute error and stability did not change significantly with or without enabling HIFU (p = .6828, .6067). The detailed results are listed in .
Figure 4. Temperature measured with MR thermometry and optical temperature probes in the phantom motion study, with HIFU enabled (A) and disabled (B). In both cases, the absolute error was significantly reduced when there was no motion present. The insert shows the probe location and the analysed ROI in MR thermometry.

Table 2. Absolute error and stability of MR thermometry measured with and without motion in phantoms and in in vivo pig back muscle.
During in vivo experiments, the fiber-optic sensor was placed in unheated tissue away from the target region to avoid artifacts arising from interaction of the ultrasound beam and the sensor (, insert). During HIFU enabled sonications (), the maximum error was 4.0 ± 1.6 °C during free breathing and was reduced to 3.1 ± 1.6 °C during breath hold periods. Similarly, in experiments with HIFU disabled () the maximum error was 3.5 ± 1.0 °C during free breathing, and was reduced to 2.0 ± 0.6 °C during forced breath holds. In both cases, the accuracy and stability of MR thermometry improved during breath-hold periods compared to free-breathing periods. Moreover, during motion-hold periods, absolute error and stability did not change significantly with or without enabling HIFU (p = .6828, .0560). The detailed results are listed in .
Figure 5. Temperature measured with MR thermometry and an optical temperature probe in the pig study when HIFU is enabled (A) and disabled (B). In both cases, the absolute error was significantly reduced when there was no motion present. The insert shows the probe location and the analysed ROI in MR thermometry.

The absolute error during each breath-hold session increased over time with a small but significant amount (e.g., from 0.5 ± 0.4 °C at the beginning to 1.3 ± 1.0 °C at the end of 10-min hyperthermia treatment) during in vivo experiments (, red). No significant trend was observed in phantom experiments (, blue).
Figure 6. The breath-hold sessions during in vivo 10 minutes hyperthermia treatment (A, black arrows) and the MR thermometry absolute error for each session (B). The error changed as a function of time with bars represent mean ± SEM across all experiments (n = 6 for phantom, n = 12 for in vivo). The graph suggests the absolute error tends to increase over time and the change was more pronounced in the pig study than that in phantom.

Feasibility of breath-hold hyperthermia treatment
MR-HIFU hyperthermia delivered during intermittent forced breath holds over 10-min durations with a target temperature of 42.5 °C were completed successfully in all 8 treatments (n = 3 with phantom, n = 5 in vivo). The 18-mm diameter target region () was heated above 41 °C for 9.6 ± 1.0 min in pigs, and 9.1 ± 0.4 min in the phantom, demonstrating the feasibility of breath-hold MR-HIFU hyperthermia (). Negligible overheating was introduced during the treatment (time for temperature higher than 44 °C = 0.01 ± 0.02 min). Median (10th, 90th percentile) temperatures during breath holds were 41.1 ± 1.6 °C (40.3 ± 1.4 to 41.9 ± 1.8 °C) in phantoms, and 41.1 ± 1.4 °C (40.1 ± 1.4 to 42.1 ± 1.5 °C) in vivo. The mean ± standard deviation diameter and length of the 10 min 41 °C isocontour was 22.3 ± 0.5 mm (diameter), 50.5 ± 3.9 mm (length) in phantoms, and 28.7 ± 4.7 mm (diameter), 51.5 ± 6.0 mm (length) in vivo.
Figure 7. In vivo mild hyperthermia treatment in a pig. Treatment planning image (A) and heating pattern (B) indicate effective hyperthermia treatment within target region (black contour shows 42 °C isotherm). Bar = 5 cm. Temperature curve (C) indicates that mild heating (41–45 °C) within target region was achieved for 10.0 minutes, confirming the feasibility of breath-hold mild hyperthermia treatment. Gray bars indicate the power level during the treatment, and green curve indicates a stable body temperature measured using invasive probe.

Effect of breath-hold and free-breathing durations
Prior to the estimation of different combination of breath-hold and free-breathing durations, the following parameters for heating rate as well as thermal diffusivity were obtained from the phantom and in-vivo experiments: The initial heating rate estimated during the first breath hold of each hyperthermia experiment was 0.23 °C/s in phantoms and 0.13 °C/s in vivo (). During cooling, the thermal diffusivity estimated based on the expanding Gaussian temperature distribution was 0.16 mm2/s in phantoms and 0.17 mm2/s in vivo (). The perfusion rate was estimated to be 0.00104 g/cm3/s across 5 in-vivo datasets ().
Figure 8. The fitting curve for heating and cooling period in phantom and pig experiment. The black thick line is the overall fitting curve across all datasets, whereas the thin colored curves are raw temperature measurements from MR thermometry. A, B and C, D are for heating and cooling period respectively. The fitting coefficients were acquired based on the accurate temperature measurements during breath hold.

The minimum in-vivo forced breath-hold period was estimated to be 61 s for an initial temperature rise from body temperature (37 °C) to a maximum allowable temperature (45 °C), and 38 s to reach to the ideal treatment temperature (42 °C). In practice, at least two extra imaging dynamics (∼7 s) should be added to the breath-hold period to allow stabilization of temperature maps after motion. During steady-state heating, the minimum breath-hold period to heat from a low of 41 °C to a high of 44 °C was 30 s plus 2 extra dynamics (7 s) for temperature stabilization. The maximum free-breathing period was 164 s to cool from the maximum temperature of 45 to 41 °C, and 88 s to cool from the practice case (43 °C) to 41 °C to maintain the hyperthermia effect.
Effective heating duration was evaluated using the periods of time when the target temperature is in the range of 41–45 °C (TIR). TIR using various combinations of breath-hold and free-breathing periods for 10-min hyperthermia treatment are shown in . For 20 s breath-hold and 60 s free-breathing periods, TIR for a 10-min treatment was estimated to be 7.8 min (10th/90th percentile for temperature distribution: 42.4/44.7 °C). Increasing the breath-hold period to 60 s (at a fixed free-breathing period) improved the heating by increasing the TIR to 9.5 min (10th/90th percentile for temperature distribution: 42.3/44.6 °C). On the other hand, increasing the free-breathing period to 120 s (for fixed breath-hold of 20 s) reduced TIR to 3.1 min (10th/90th percentile for temperature distribution: 40.3/42.4 °C). depicts this interrelationship between free-breathing and breath-hold durations to aid in selection of appropriate combinations for a 10-min hyperthermia treatment. Using this model, an optimal treatment effect (TIR > 9 min) could be achieved when combining 36–60 s breath-hold period with 60–155.5 s free-breathing.
Figure 9. Shows the time in range (TIR) estimates based on various combinations of breath-hold and free breathing periods. Panel A shows examples of the heating profile in time for different combinations. At a fixed free-breathing period, shorter breath-hold period generated less heating effect and shorter TIR (first and second row). On the other hand, with a fixed breath-hold period, longer free-breathing period resulted in a shorter TIR (first and third row). Panel B depicts a contour map of TIR for various combinations of breath hold and free breathing duration for a 10-minutes hyperthermia treatment. Contour lines indicate the suggested combination to achieve a TIR of 7, 8, and 9 minutes, respectively.

Discussion
In this study, we demonstrated the feasibility of using repeated breath holds to mitigate the effects of motion artifacts on MRI-controlled HIFU mild hyperthermia. Experiments performed with a moving metal object near a stationary phantom, and in pig back muscle, reproduced the situation that occurs when delivering hyperthermia to stationary targets near moving organs with a large susceptibility difference. In patients, tumors in the abdomen, chest, or proximal arms near the lungs are susceptible to these effects [Citation26]. In pigs, temperature maps calculated from images acquired during forced breath holds at the same respiratory phase as the initial reference images demonstrated stable temperature measurements with a temporal standard deviation of 0.7 ± 0.2 °C, compared to 1.3 ± 0.5 °C for images acquired during free breathing. This finding confirmed the benefits of applying breath holds in mild hyperthermia treatment to achieve an adequate accuracy and stability of MR thermometry. Based on measured heating and cooling rates, practical ranges of breath-hold and free-breathing durations were identified, subject to constraints of collecting at least two artifact-free sets of temperature images before applying HIFU in each breath-hold period and maintaining the minimum temperature (41 °C) after each free-breathing period. Our estimates suggest a minimum forced breath-hold period of ∼40 s during the initial heating to reach the ideal treatment temperature of 42 °C (∼1 min to reach the maximum temperature of 45 °C), and 30 s thereafter to maintain stable temperatures between 41 to 44 °C with free-breathing periods up to 2–3 min in this model. These precise parameters will vary in different tissues due to differing perfusion rates as well as with the HIFU power applied and goal treatment volume. However, breath hold durations of this magnitude are easily clinically achievable and are shorter than the breath-hold durations commonly used in pediatric diagnostic imaging suggesting this is a clinically tractable approach.
Our validated measurements of MR temperature mapping stability during breath holds compare favorably with previous measurements of MR thermometry during MR-HIFU hyperthermia made by Tillander et al. [Citation23], Chu et al. [Citation22], and Zhang et al. [Citation36] in pigs and Chu et al. [Citation22] in volunteers with rectal cancer, Zhang [Citation40] in patients with symptomatic uterine fibroids (). On the other hand, the values of diffusivity found in this study compare reasonably well with those identified previously by Dragonu [Citation37], Dillon [Citation41], and Zhang [Citation36,Citation40] (). By applying the diffusivity estimated from the previous studies (mean diffusivity across 7 studies in = 0.15 ± 0.01 mm2/s) and the absorption coefficient estimated from this system in this model, the suggested combination of breath-hold and free-breathing periods for an optimal treatment effect (TIR > 9 min) is 36–60 s (forced breath-hold) and 60–171 s (free-breathing). However, it is also possible to achieve the target temperature over a longer period of time with shorter breath-hold duration. This strategy could be beneficial if extending the technique to awake patients who are voluntarily holding their breath as opposed to anesthetized patients under forced breath-hold. These results further confirmed the feasibility and effectiveness of our proposed breath-hold MR-HIFU hyperthermia treatment.
Table 3. Comparison of the accuracy and stability of MR thermometry evaluated in this study with other references (A) and the estimated diffusivity generated with our model compared with other studies (B).
Clinically, MR thermometry precision and accuracy are stable in the extremities, but highly variable in targets affected by respiratory motion [Citation28,Citation42]. In a retrospective review of 121 pediatric patients with sarcoma and 61 patients with neuroblastoma, it was identified that respiratory motion compensation such as breath holds would increase the number of targetable primary sarcoma and neuroblastoma lesions from 67 to 77, and from 0 to 15, respectively, by enabling treatment of more abdominal, pelvic, chest, and proximal arm lesions [Citation26]. Our preliminary study on 7 pediatric patients with tumors confirmed the necessity of extra attention when using MR thermometry for temperature measurement during hyperthermia as this technique requires precise temperature control to avoid unintended tissue damage from overheating and lack of efficacy from underheating. The overall quality of MR thermometry was much worse when applied in the tumors grown in the chest, spine or pelvis, compared to the tumors in limbs. Particularly, when performing ventilated breath on the patient with pelvis tumor, the quality of MR thermometry improved significantly, indicating a possible solution to this problem. Previous studies developed methods for performing MR-HIFU in abdominal organs under free breathing, which requires motion-corrected MR thermometry [Citation43–45], multi-baseline MR thermometry [Citation46–49], and target tracking [Citation46,Citation50–52]. These techniques have shown great promise for short ablative sonications and have provided an alternative modality for those patients who are not suitable for general anesthesia or breath hold. However, these methods are usually not commercially available, requiring for additional setup time and dedicated imaging coils, and also involving computationally intensive real-time image processing. Most importantly, these techniques are still sensitive to susceptibility changes during therapy, especially for longer duration mild hyperthermia. The advantage of the breath-hold approach is that it can be used with standard MR thermometry acquisition and processing. Our validation of thermometry stability and hyperthermia feasibility demonstrate the potential for this technique to be applied in clinical investigations of MR-HIFU hyperthermia.
This study has several important limitations. Our approach relies on periodic respiratory motion with breath holds always conducted at the same phase of the respiratory cycle. This may be clinically impractical in adult hyperthermia treatments, which are commonly delivered under conscious sedation to incorporate pain as an important part of treatment feedback. However, in young children, general anesthesia is acceptable in diagnostic imaging and likely necessary for all MR-HIFU hyperthermia treatments as patients are unable to lay still for the required duration on the MR table. We did not evaluate the effect of using end-inspiration versus end-expiration breath holds. In pediatric MR imaging, end-expiration breath holds are more reproducible [Citation33,Citation53,Citation54]; similar conclusions arise from the adult abdominal imaging and respiratory-gated radiotherapy [Citation55,Citation56]. Another limitation of our study is that heating experiments were limited to 10 min, which allowed for repeated measurements and assessment of variability, whereas longer durations may be desirable for applications like drug release from temperature-sensitive liposomes [Citation57]. Further, the ventilator and patient monitoring system were not configured to electronically trigger breath holds or record relevant vital signs. Future development of breath hold MR HIFU hyperthermia should aim to record these values for further refinement of the safety and efficiency of the technique. Lastly, the MR thermometry absolute error increased over time with a small amount in vivo but not with phantom experiments. This may suggest that further refinements to this technique are necessary to enable long duration breath-hold MR-HIFU hyperthermia treatment in the clinical practice.
Conclusion
In conclusion, MRI controlled high intensity focused ultrasound mild hyperthermia in targets where MR thermometry is affected by periodic motion of nearby anatomical structures is feasible when heating is delivered during forced breath holds. These results can be used to provide guidance on the potential clinical applications.
Acknowledgement
This study was funded by the Cancer Prevention and Research Institute of Texas (CPRIT, #R1308), the National Institutes of Health (NIH, #R01CA199937), and the Hyundai Hope on Wheels Foundation. The authors would like to thank Mr. Cecil Futch for the assistance he provided during the in vivo experiments.
Disclosure statement
Robert Staruch was a paid employee of Philips Research, and is now a paid employee of Profound Medical. Rajiv Chopra has financial interests in Profound Medical. No other authors report any conflicts of interest.
Additional information
Funding
References
- Datta NR, Ordóñez SG, Gaipl US, et al. Local hyperthermia combined with radiotherapy and-/or chemotherapy: recent advances and promises for the future. Cancer Treat Rev. 2015;41(9):742–753.
- Hurwitz M, Stauffer P. Hyperthermia, radiation and chemotherapy: the role of heat in multidisciplinary cancer care. Semin Oncol. 2014;41(6):714–729.
- Wust P, Hildebrandt B, Sreenivasa G, et al. Hyperthermia in combined treatment of cancer. Lancet Oncol. 2002;3(8):487–497.
- van der Zee J, González D, van Rhoon GC, et al. Comparison of radiotherapy alone with radiotherapy plus hyperthermia in locally advanced pelvic tumours: a prospective, randomised, multicentre trial. Lancet. 2000;355(9210):1119–1125.
- Issels RD, Lindner LH, Verweij J, et al. Neo-adjuvant chemotherapy alone or with regional hyperthermia for localised high-risk soft-tissue sarcoma: a randomised phase 3 multicentre study. Lancet Oncol. 2010;11(6):561–570.
- Song CW. Effect of local hyperthermia on blood flow and microenvironment: a review. Cancer Res. 1984;44:(10 Suppl):4721s–4730s.
- Urano M, Kuroda M, Nishimura Y. For the clinical application of thermochemotherapy given at mild temperatures. Int J Hyperthermia. 1999;15:79–107.
- Issels RD. Hyperthermia adds to chemotherapy. Eur J Cancer. 2008;44(17):2546–2554.
- Staruch RM, Hynynen K, Chopra R. Hyperthermia-mediated doxorubicin release from thermosensitive liposomes using MR-HIFU: therapeutic effect in rabbit Vx2 tumours. Int J Hyperth. 2015;31(2):118–133.
- Calderwood SK, Theriault JR, Gong J. How is the immune response affected by hyperthermia and heat shock proteins? Int J Hyperth. 2005;21(8):713–716.
- Skitzki JJ, Repasky EA, Evans SS. Hyperthermia as an immunotherapy strategy for cancer. Curr Opin Investig Drugs. 2009;10:550–558.
- Toraya-Brown S, Fiering S. Local tumour hyperthermia as immunotherapy for metastatic cancer. Int J Hyperthermia. 2014;30(8):531–539.
- Frey B, Weiss E-M, Rubner Y, et al. Old and new facts about hyperthermia-induced modulations of the immune system. Int J Hyperthermia. 2012;28(6):528–542.
- Perez CA, Gillespie B, Pajak T, et al. Quality assurance problems in clinical hyperthermia and their impact on therapeutic outcome: a Report by the Radiation Therapy Oncology Group. Int J Radiat Oncol Biol Phys. 1989;16(3):551–558.
- Trefná HD, Crezee H, Schmidt M, et al. Quality assurance guidelines for superficial hyperthermia clinical trials: I. Clinical requirements. Int J Hyperth. 2017;33(4):471–482.
- Emami B, Scott C, Perez CA, et al. Phase III study of interstitial thermoradiotherapy compared with interstitial radiotherapy alone in the treatment of recurrent or persistent human tumors. A prospectively controlled randomized study by the Radiation Therapy Group. Int J Radiat Oncol Biol Phys. 1996;34(5):1097–1104.
- Gellermann J, Wlodarczyk W, Feussner A, et al. Methods and potentials of magnetic resonance imaging for monitoring radiofrequency hyperthermia in a hybrid system. Int J Hyperth. 2005;21:497–513.
- Numan WCM, Hofstetter LW, Kotek G, et al. Exploration of MR-guided head and neck hyperthermia by phantom testing of a modified prototype applicator for use with proton resonance frequency shift thermometry. Int J Hyperth. 2014;30(3):184–191.
- Mougenot C, Tillander M, Koskela J, et al. High intensity focused ultrasound with large aperture transducers: A MRI based focal point correction for tissue heterogeneity. Med Phys. 2012;39(4):1936–1945.
- Partanen A, Tillander M, Yarmolenko PS, et al. Reduction of peak acoustic pressure and shaping of heated region by use of multifoci sonications in MR-guided high-intensity focused ultrasound mediated mild hyperthermia. Med Phys. 2012;40(1):013301.
- Mougenot C, Quesson B, de Senneville BD, et al. Three-dimensional spatial and temporal temperature control with MR thermometry-guided focused ultrasound (MRgHIFU). Magn Reson Med. 2009;61(3):603–614.
- Chu W, Staruch RM, Pichardo S, et al. Magnetic Resonance–Guided High-Intensity focused ultrasound hyperthermia for recurrent rectal cancer: MR thermometry evaluation and preclinical validation. Int J Radiat Oncol. 2016;95(4):1259–1267.
- Tillander M, Hokland S, Koskela J, et al. High intensity focused ultrasound induced in vivo large volume hyperthermia under 3D MRI temperature control. Med Phys. 2016;43(3):1539–1549.
- Rieke V, Butts Pauly K. MR thermometry. J Magn Reson Imaging. 2008;27:376–390.
- Ishihara Y, Calderon A, Watanabe H, et al. A precise and fast temperature mapping using water proton chemical shift. Magn Reson Med. 1995;34:814–823.
- Shim J, Staruch RM, Koral K, et al. Pediatric sarcomas are targetable by MR-Guided High Intensity Focused Ultrasound (MR-HIFU): anatomical distribution and radiological characteristics. Pediatr Blood Cancer. 2016;63(10):1753–1760.
- Aubry J-F, Pauly KB, Moonen C, et al. The road to clinical use of high-intensity focused ultrasound for liver cancer: technical and clinical consensus. J Ther Ultrasound. 2013;1(1):13.
- Kothapalli A, Khattak MA. Safety and efficacy of anti-PD-1 therapy for metastatic melanoma and non-small-cell lung cancer in patients with viral hepatitis. Melanoma Res. 2018;28:1.
- Okada A, Murakami T, Mikami K, et al. A case of hepatocellular carcinoma treated by MR-guided focused ultrasound ablation with respiratory gating. MRMS. 2006;5(3):167–171.
- Häcker A, Peters K, Knoll T, et al. Ablation of clinically relevant kidney tissue volumes by High-Intensity focused ultrasound: preliminary results of standardized Ex-Vivo investigations. J Endourol. 2006;20(11):930–938.
- Anzidei M, Napoli A, Sandolo F, et al. Magnetic Resonance-Guided focused ultrasound ablation in abdominal moving organs: a feasibility study in selected cases of pancreatic and liver cancer. Cardiovasc Inter Rad. 2014;37(6):1611–1617.
- van Breugel JMM, Wijlemans JW, Vaessen HHB, et al. Procedural sedation and analgesia for respiratory-gated MR-HIFU in the liver: a feasibility study. J Ther Ultrasound. 2016;4:19.
- Jaimes C, Gee MS. Strategies to minimize sedation in pediatric body magnetic resonance imaging. Pediatr Radiol. 2016;46:916–927.
- Köhler MO, Mougenot C, Quesson B, et al. Volumetric HIFU ablation under 3D guidance of rapid MRI thermometry. Med Phys. 2009;36(8):3521–3535.
- Nofiele J, Yuan Q, Kazem M, et al. An MRI-compatible platform for one-dimensional motion management studies in MRI. Magn Reson Med. 2016;76(2):702–712.
- Zhang J, Mougenot C, Partanen A, et al. Volumetric MRI-guided high-intensity focused ultrasound for noninvasive, in vivo determination of tissue thermal conductivity: Initial experience in a pig model. J Magn Reson Imaging. 2013;37(4):950–957.
- Dragonu I, de Oliveira PL, Laurent C, et al. Non-invasive determination of tissue thermal parameters from high intensity focused ultrasound treatment monitored by volumetric MRI thermometry. NMR Biomed. 2009;22(8):843–851.
- Cheng H-L, Plewes DB. Tissue thermal conductivity by magnetic resonance thermometry and focused ultrasound heating. J Magn Reson Imaging. 2002;16(5):598–609.
- Dillon CR, Vyas U, Payne A, et al. An analytical solution for improved HIFU SAR estimation. Phys Med Biol. 2012;57(14):4527–4544.
- Zhang J, Fischer J, Warner L, et al. Noninvasive, in vivo determination of uterine fibroid thermal conductivity in MRI-guided high intensity focused ultrasound therapy. J Magn Reson Imaging. 2015;41(6):1654–1661.
- Dillon CR, Payne A, Christensen DA, et al. The accuracy and precision of two non-invasive, magnetic resonance-guided focused ultrasound-based thermal diffusivity estimation methods. Int J Hyperth. 2014;30(6):362–371.
- Laetsch TW, Staruch RM, Koral K, et al. Prospective imaging study of magnetic resonance thermometry quality in pediatric solid tumors. Int Congr Hyperthermic Oncol. New Orleans. 2016.
- Wyatt CR, Soher BJ, MacFall JR. Correction of breathing-induced errors in magnetic resonance thermometry of hyperthermia using multiecho field fitting techniques. Med Phys. 2010;37(12):6300–6309.
- Köhler MO, Denis de Senneville B, Quesson B, et al. Spectrally selective pencil-beam navigator for motion compensation of MR-guided high-intensity focused ultrasound therapy of abdominal organs. Magn Reson Med. 2011;66(1):102–111.
- Schmitt A, Mougenot C, Chopra R. Spatiotemporal filtering of MR-temperature artifacts arising from bowel motion during transurethral MR-HIFU. Med Phys. 2014;41(11):113302.
- Vigen KK, Daniel BL, Pauly JM, et al. Triggered, navigated, multi-baseline method for proton resonance frequency temperature mapping with respiratory motion. Magn Reson Med. 2003;50:1003–1010.
- de Senneville BD, Mougenot C, Moonen C. Real-time adaptive methods for treatment of mobile organs by MRI-controlled high-intensity focused ultrasound. Magn Reson Med. 2007;57(2):319–330.
- Pichardo S, Köhler M, Lee J, et al. In vivo optimisation study for multi-baseline MR-based thermometry in the context of hyperthermia using MR-guided high intensity focused ultrasound for head and neck applications. Int J Hyperth. 2014;30:579–592.
- Grissom WA, Rieke V, Holbrook AB, et al. Hybrid referenceless and multibaseline subtraction MR thermometry for monitoring thermal therapies in moving organs. Med Phys. 2010;37:5014–5026.
- Ries M, de Senneville BD, Roujol S, et al. Real-time 3D target tracking in MRI guided focused ultrasound ablations in moving tissues. Magn Reson Med. 2010;64(6):1704–1712.
- Holbrook AB, Ghanouni P, Santos JM, et al. Respiration based steering for high intensity focused ultrasound liver ablation. Magn Reson Med. 2014;71(2):797–806.
- Holbrook AB, Santos JM, Kaye E, et al. Real-time MR thermometry for monitoring HIFU ablations of the liver. Magn Reson Med. 2010;63(2):365–373.
- Mevada ST, Al-Mahruqi N, El-Beshlawi I, et al. Single Breath-Hold physiotherapy technique: effective tool for T2* magnetic resonance imaging in young patients with thalassaemia major. SQUMJ. 2016;16(1):e78–81.
- Serai SD, Dillman JR, Trout AT. Spin-echo Echo-planar Imaging MR Elastography versus Gradient-echo MR elastography for assessment of liver stiffness in children and young adults suspected of having liver disease. Radiology. 2017;282:761–770.
- Kim T, Kim S, Park Y-K, et al. Motion management within two respiratory-gating windows: feasibility study of dual quasi-breath-hold technique in gated medical procedures. Phys Med Biol. 2014;59(21):6583–6594.
- Keall PJ, Chang M, Benedict S, et al. Investigating the temporal effects of Respiratory-Gated and Intensity-Modulated radiotherapy treatment delivery on in vitro survival: an experimental and theoretical study. Int J Radiat Oncol. 2008;71(5):1547–1552.
- Bing C, Patel P, Staruch RM, et al. Longer heating duration increases localized doxorubicin deposition and therapeutic index in Vx2 tumors using MR-HIFU mild hyperthermia and thermosensitive liposomal doxorubicin. Int J Hyperther. 2019;36:196–203.