Abstract
The depth dose profiles of photons mirror those of fast neutrons. However, in contrast to the high linear energy transfer (LET) characteristics of neutrons; photons exhibit low LET features. Hyperthermia (HT) inhibits the repair of radiation-induced DNA damage and is cytotoxic to the radioresistant hypoxic tumor cells. Thus, thermoradiobiologically, HT simulates high LET radiation with photons. At temperatures of 39–45 °C, the physiological vasodilation allows rapid heat dissipation from normal tissues. On the contrary, the chaotic and relatively rigid tumor vasculature results in heat retention leading to higher intratumoural temperatures. Consequently, the high LET attributes of HT with photon radiations are mostly limited to the confines of the heated tumor while the normothermic normal tissues would be irradiated with low LET photons. HT thereby augments photon therapy by conferring therapeutic advantages of high LET radiations to the tumors akin to neutrons, while the ‘heat-sink’ effect spares the normal tissues from thermal radiosensitization. Thus, photon thermoradiotherapy imparts radiobiological advantages selectively to tumors analogous to neutrons without exaggerating normal tissue morbidities. The later has been the major concern with clinical fast neutron beam therapy. Outcomes reported from several clinical trials in diverse tumor sites add testimony to the enhanced therapeutic efficacy of photon thermoradiotherapy.
Introduction
Fast neutron therapy was introduced in 1938 with the belief that its favorable radiobiological features would help overcome some of the inherent limitations of X or γ-ray photon radiotherapy (RT) [Citation1]. Compared with photons, fast neutron beams have a higher linear energy transfer (LET) (20–100 keV/μ for neutrons vs. 0.2–2 keV/μ with photons), a higher relative biological effectiveness (RBE) (2.5–3.0) and a lower oxygen enhancement ratio (OER) (1.4–1.7) [Citation2–5]. However, the physical depth dose distribution profiles of both are similar [Citation6]. The radiobiological gains with a higher LET and a higher RBE could be counterproductive with neutrons as, in contrast to protons or 12C ions, the absence of Bragg peak would lead to irradiation of the entire treatment volume with a high LET neutron beam. This could deliver unwanted higher biologically effective doses (BED) to adjacent normal tissues, resulting in higher toxicities. Thus, although a favorable tumor response has been reported for various tumor sites with neutrons, the advantage was largely offset by higher late tissue toxicities, increased risk of cancer induction and growing concerns regarding radiation protection [Citation1,Citation2,Citation5,Citation7]. These apprehensions contributed to the gradual decline in popularity of fast neutron therapy in modern RT practice.
Mild to moderate local hyperthermia (HT) is the elevation of the local tumor temperature to between 39 and 45 °C [Citation8,Citation9]. This achieves selective cytotoxic effects on the relatively radioresistant hypoxic cells, S-phase cells and reduces the repair of radiation-induced DNA damage [Citation10–12]. These features resemble those of high LET radiation [Citation13,Citation14]. HT is thus a potent radio- and chemosensitizer with immunomodulatory effects, analogous to ‘in situ tumour vaccination’ [Citation10–12, Citation15–18]. These attributes of HT have translated into positive outcomes with RT and/or chemotherapy (CT), as reported in several randomized studies and meta-analyses for different tumor sites [Citation10,Citation12,Citation19–23].
The vasculature of solid tumors is anatomically and physiologically different to normal tissue vasculature in terms of its response to higher temperatures [Citation24–26]. This may impart selective thermal sensitivity to tumors and provide a rationale for combining HT with X or γ-ray radiation based on the inherent differences in the vasculature architecture between tumors and normal tissues. The radiobiological advantages of simulated high LET radiation, achieved by coupling HT with photons, could provide a distinctive therapeutic advantage akin to neutrons. In the absence of additional normal tissue morbidity, this could result in an improved therapeutic ratio. This communication evaluates the physiological, radiobiological, physical and clinical implications of photon thermoradiotherapy (HTRT) as a surrogate for fast neutron therapy and therefore does not address other neutron sources including boron neutron capture and 252Cf brachytherapy.
Effects of hyperthermia on tumor and normal tissue vasculature
A rise in temperature following local HT profoundly augments normal tissue blood flow as a consequence of physiological vasodilation. This results in a significant increase in functional vascular volume and vascular permeability leading to efficient dissipation of heat from the normal tissues known as the ‘heat-sink’ effect [Citation24,–Citation25]. In contrast, solid tumors have a coarse, elongated, dilated, tortuous capillary network with redundant bending and are devoid of smooth muscle and innervation. As tumor vessels are unable to physiologically auto regulate, they fail to ‘wash out’ heat when exposed to raised temperatures [Citation24–27].
Mild to moderate HT initially leads to increased blood perfusion, an improved microcirculation and increased oxygen delivery in tumors. Higher temperatures could result in vascular damage resulting in greater hypoxia [Citation25,Citation27]. Over time, heat dissipates slowly from tumors compared with normal tissues resulting in a ‘heat-trap’ effect within the tumors [Citation12,Citation24,Citation25]. This has been well documented through direct tumor pO2 measurements, immunohistochemistry and imaging studies [Citation25,Citation26]. Higher tumor temperatures could thus facilitate the effects of local HT within the tumor leading to HT-induced selective radiosensitization of the tumor whilst sparing the adjacent normal tissues [Citation12,Citation24–26]. The implications of differential thermal radiosensitization in tumors and normal tissues are discussed later.
Hyperthermia with X-rays or neutrons: in vitro studies and clinical implications
In vitro studies have shown that HT alone at 42–45 °C is cytotoxic to the tumor cells with the critical temperature being 43 °C, typically depicted by the Arrhenius plot [Citation28,Citation29]. With low LET X-rays, HT has been shown to increase cell kill by achieving a reduction in the shoulder (Dq) and slope (Do) of the cell survival curves [Citation30,Citation31]. The slope of the cell survival curves with low LET radiation were reported to decrease by a factor of nearly 2 between 37 and 43 °C, while with high LET 12C ions, the slope was nearly unchanged with HT [Citation30]. Thus, HT exhibits greater thermal synergy with low LET radiation, such as photons or protons, in contrast to high LET radiation [Citation30–32]. This could be attributed to the enhanced quadratic (β) component of cell kill by inhibiting RT-induced sublethal and potentially lethal DNA damage which are more prevalent with low LET than high LET radiation [Citation22,Citation33]. The inhibition of DNA repair that results in higher cell kill by HT occurs primarily through the inhibition of homologous recombination repair pathway, although the interaction with non-homologous end joining repair continues to be debated [Citation32,Citation34].
The presence of hypoxic cells within solid tumors is a known limiting factor with low LET radiation (photons and protons). These are best treated with high LET radiation whereby the OER falls to 1.2 at around 200 keV/µm compared with 2.8–3.0 for protons or photons [Citation13,Citation27,Citation35]. However, the lack of oxygen effect with HT offers additional thermoradiobiological advantages in clinical situations with low LET radiation similar to high LET radiation [Citation29]. Thus as proposed by Robinson et al [Citation14], adding HT to low LET radiation provides potentially a viable alternative to heavy particle therapy.
Neutrons produce recoil protons, α-particles and heavier nuclear fragments and are thus high LET radiation and induce mostly irreparable lethal DNA damage. Thus adding HT to neutrons would not significantly alter the cell survival curves as demonstrated by Hahn et al. [Citation36], where the slope of the fast neutron irradiation cell survival curves remained unchanged by the addition of HT (). In contrast, low LET X-ray survival curves became steeper with loss of the ‘shoulder’ in the presence of HT, resembling high LET radiation such as neutrons. This could be attributed to the inhibition of the DNA repair kinetics with HT ().
Figure 1. Local hyperthermia (LTH) markedly influences survival of Ridgway osteogenic sarcoma cell lines. Cell survival curves following fractionated X-ray irradiation, making them steeper and reducing the shoulder. The curves almost assume the shape following fast neutron irradiation alone. The effect of LTH on cell curves with fast neutrons is minimal (Reprinted from Hahn et al. [Citation36] with permission, ©2019 Radiation Research Society).
![Figure 1. Local hyperthermia (LTH) markedly influences survival of Ridgway osteogenic sarcoma cell lines. Cell survival curves following fractionated X-ray irradiation, making them steeper and reducing the shoulder. The curves almost assume the shape following fast neutron irradiation alone. The effect of LTH on cell curves with fast neutrons is minimal (Reprinted from Hahn et al. [Citation36] with permission, ©2019 Radiation Research Society).](/cms/asset/b296c2fa-c247-4dec-8cea-6a32d5ac09e7/ihyt_a_1679895_f0001_b.jpg)
Clinical delivery of HT usually involves a gradual step-wise increase in temperature before or after RT for around 60–90 min to achieve a tumor temperature of around 41–42 °C, respecting the patient’s tolerance of heat during the entire procedure [Citation19,Citation20,Citation37–39]. In view of the differential vascular configuration between tumors and normal tissues, during the heating process tumors preferentially would be retaining heat compared to the normal structures. Thus, the inherent diverse vascular physiology would contribute towards a selective HT-induced radiosensitization in tumors alone while exempting the normothermic normal tissues. This is also reflected in the low incidence of both acute and late toxicities reported with HT and photon RT from various clinical trials [Citation19,Citation20,Citation38,Citation40,Citation41].
Dose profiles of photons and neutrons: Implications with hyperthermia
The depth dose characteristics of fast neutron beams mirror those of X- or γ radiation and are dependent primarily upon the neutron beam energy, nature of the accelerated ions, target material, its thickness and source-skin distance [Citation6]. Radiobiologically, high LET fast neutrons exhibit a significantly enhanced biological effectiveness along their path HT as compared with low LET photons.
As neutrons lack a Bragg peak, the entire irradiated volume would receive high LET irradiation. Although the higher RBE could be advantageous for tumors, it would increase the normal tissue toxicity as demonstrated in previous clinical studies [Citation1,Citation2,Citation7]. Photon beam dose profiles can be optimized to conform to target the tumor volumes. Due to the selective heat retention within the tumors, features of high LET irradiation could be imparted to the tumor whilst the normothermic organs at risk and normal structures would be irradiated by the low LET photon beam alone ().
Figure 2. Hyperthermia with photon radiotherapy selectively confers the radiobiological advantages of high LET radiation (e.g. neutrons) to tumors. Normal tissues, with a heat sink effect, would not undergo thermal radiosensitization, thus retaining low LET characteristics with photons. The physical dose profile of photons is similar to that of neutrons in the irradiated volume.
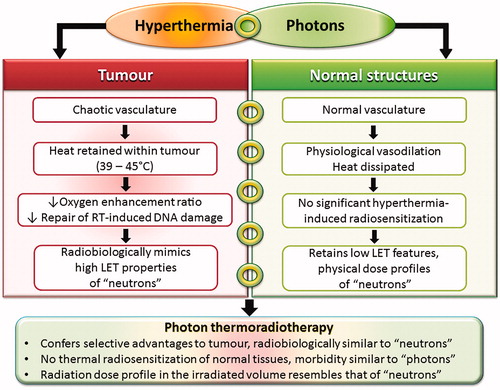
Photon therapy with HT could thus radiobiologically simulate fast neutrons in tumors (). An improvement in the therapeutic ratio would be expected with photon HTRT as this would provide a selective thermoradiobiological advantage in the tumor only without the additional normal tissue morbidity which is a major concern with neutrons. Photon HTRT could provide a cost effective alternative to fast neutron therapy with a greater therapeutic window.
An alternative approach of combining HT with protons has been shown to mimic 12C ion therapy. This has been discussed in details in our earlier publication [Citation13]. Briefly, due to their Bragg peak, both protons and 12C ion therapy, share similar physical dose distributions characterized by a low-dose entrance region, a homogeneous high-dose region at the target, followed by a steep fall-off to an almost negligible dose beyond. Radiobiologically, protons and photons are low LET radiation in contrast to the high LET characteristics of a 12C ion. Thus the thermoradiobiological advantages of HT coupled with the physical dose distribution of protons enables proton HTRT to mimic 12C ion therapy [Citation13] (). Early clinical studies have demonstrated the feasibility and effectivity of proton HTRT in radioresistant unresectable soft tissue sarcomas and large unresectable chordomas, both of which respond poorly to conventional photons or protons [Citation42,Citation43].
Figure 3. Hyperthermia with photons and protons is thermoradiobiologically analogous to high LET radiation. The upper panel illustrates the selective advantages of photon beam thermoradiotherapy in tumors, analogous to high LET neutron irradiation. Normal tissues do not show any significant thermal sensitization and are irradiated with low LET photons. A selective advantage for tumors is expected to improve the therapeutic ratio. The lower panel depicts the rationale of combining proton therapy and hyperthermia to mimic 12C ion therapy. Lower panel has been modified and reprinted from Datta et al. [Citation13] with permission, ©2019 Francis & Taylor Group.
![Figure 3. Hyperthermia with photons and protons is thermoradiobiologically analogous to high LET radiation. The upper panel illustrates the selective advantages of photon beam thermoradiotherapy in tumors, analogous to high LET neutron irradiation. Normal tissues do not show any significant thermal sensitization and are irradiated with low LET photons. A selective advantage for tumors is expected to improve the therapeutic ratio. The lower panel depicts the rationale of combining proton therapy and hyperthermia to mimic 12C ion therapy. Lower panel has been modified and reprinted from Datta et al. [Citation13] with permission, ©2019 Francis & Taylor Group.](/cms/asset/99ff7a11-c838-492f-8f11-e5cdfef69f6c/ihyt_a_1679895_f0003_c.jpg)
Clinical outcomes: Hyperthermia and photon radiotherapy
Like fast neutron beam therapy, whose efficacy has been demonstrated in various tumor sites namely cancers of the salivary glands, paranasal sinuses, head and neck as well as soft tissue sarcoma and prostate cancer; HT with photon RT is also reported to be effective in a wide spectrum of malignancies, including conventionally radioresistant melanoma [Citation7,Citation10,Citation12,Citation44]. However, the higher late toxicities reported using fast neutron beam therapy have been a matter of concern and have restricted the use of fast neutron beam therapy globally [Citation2]. This has been attributed in part to suboptimal treatment, inherent technical limitations and overlooking the higher RBE of neutrons in clinical trials [Citation2]. Nuclear interactions result in recoil protons and low energy charged nuclear fragments leading to uncertainties in the quantification of absorbed dose and the relationship with clinical responses. The RBE of neutrons shows great variability according to dose level, dose fractionation and tissue oxygenation [Citation1]. It is now accepted that the RBE of fast neutrons is 3–3.5 for most normal tissue late effects but could be higher for the central nervous system (RBE≈4–4.5) and salivary glands (RBE≈8) [Citation2]. The selective benefit of high LET tumor irradiation for 12C ions due to their Bragg peak, is lost with neutrons. Thus, tumor and normal tissues within neutron-irradiated volumes, which shares depth dose profiles similar to photons, could result in enhanced acute and late normal tissue morbidity.
With photon HTRT, both randomized and non-randomized studies have reported an improvement in therapeutic outcomes in a wide range of malignancies compared to X or γ-irradiation alone [Citation10,Citation12]. A significant odds ratio of 2.3 (95% CI 1.95–2.72, p < .001) has been reported in favor of HTRT for complete response following HTRT versus RT in different malignancies from 38 clinical trials totaling 3478 patients (HTRT: 1761; RT: 1717) [Citation10]. Furthermore, meta-analyses of HT and photon RT in locally advanced cervix cancer, locally recurrent breast cancer and locally advanced head and neck cancer have all consistently shown a significant improvement of 22–25% in complete response rate using photon HTRT compared to RT alone [Citation19–21].
In clinical studies, the addition of HT to RT is not reported to significantly increase acute or late toxicities compared with RT alone. This was evident from the meta-analysis of randomized trials between HTRT vs. RT in locally advanced cancer cervix [Citation20]. In a similar meta-analysis in superficial localized breast cancer, Vernon et al. [Citation41] reported that the addition of HT was well tolerated and did not significantly add to the clinically relevant acute or long-term toxicity over RT alone. In another meta-analysis of 779 patients of recurrent breast cancers from 16 studies who were re-irradiated with an additional mean RT dose of 36.7 Gy along with HT, none of the studies reported any significant increase in toxicities even with reirradiation and local HT [Citation19]. Similar results also emerged from the use of HT with RT in pelvic tumors (bladder, rectum and cervix) [Citation37], prostate [Citation40] and from reviews of various clinical studies in other sites [Citation10,Citation12,Citation15,Citation27,Citation38]. These indicate that photon HTRT is a safe, effective and well tolerated treatment modality in a wide range of malignancies.
The positive interaction of HT with RT and/or CT and its immunomodulatory effect creates a strong biological rationale for the clinical application of photon HTRT [Citation9,Citation16–18]. The immunomodulatory effects of HT are known to be mediated through several mechanisms – tumor cell kill, molecular changes in the tumor cell surface, heat shock proteins, exosomes and direct effects on the immune cells, namely natural killer (NK) cells, CD8 + T cells, dendritic cells (DC), macrophages and others. A detailed description of the mechanism is beyond the scope of this manuscript but has been well described in the literature [Citation10,Citation16–18]. Thus HT, as a viable and valuable addendum to the existing therapeutic modalities in cancer, has come a long way since its use in early clinical trials in the 1970s. The parallel strides in technology have further enabled HT to be delivered with certainty and ensured safer, well tolerated and an effective therapeutic modality.
The analogy of photon HTRT to neutrons is based on the presumption that intratumoural temperatures of 39–45 °C are attained during the HT sessions. However, if the desired tumor temperatures are not attained, the lack of selective thermal radiosensitization may lead to unsatisfactory outcomes [Citation44–46]. It is, therefore, imperative that effective heating with real time tumor temperature monitoring using appropriate single or multisensory temperature probes is carried out during HT sessions. Alternatively, a noninvasive volumetric assessment of temperature profiles within the tumor using proton resonance frequency shift MRI spectroscopy could be integrated as part of the HT procedure [Citation10,Citation42].
Conclusions
Thermoradiobiologically, HT imparts high LET properties to low LET proton or photon beams (). The addition of HT to photons creates a radiobiological advantage in tumors akin to fast beam neutrons while sparing the normal tissues. Such an approach has been shown to be effective in clinical trials where HT was combined with X-or γ irradiation [Citation10,Citation12,Citation19,Citation20]. As proton HTRT could mimic 12C ion therapy [Citation13], these two approaches reinforce the statement from Robinson et al. [Citation14], that HT combined with RT could be alternate to heavy particle therapy.
In view of the above physiological and thermoradiobiological implications, photon HTRT could have a wider application as X-or γ irradiation is the mainstay of RT treatment worldwide. Supplementing RT centers with superficial and deep HT facilities could improve the therapeutic outcomes and be highly cost-effective. In addition, HT is a potent chemosensitizer and has an immunomodulatory effect when combined with RT [Citation9–11,Citation16–18]. Collectively, the efficacy of HT as a multifaceted therapeutic modality justifies its integration into modern clinical oncological practice.
Acknowledgments
The authors acknowledge Dr. Susanne Rogers for reviewing the manuscript.
Disclosure statement
There are no actual or potential conflicts of interest to declare.
Additional information
Funding
References
- Goodhead DT. Neutrons are forever! Historical perspectives. Int J Radiat Biol. 2019;95:957–984.
- Laramore GE. Neutron therapy and boron neutron capture therapy In: Halperin EC, Wazer DE, Perez CA, Brady LW, editors. Perez & Brady’s principles and practice of radiation oncology. Philadelphia (PA): Wolters Kluwer Health; 2018. p. 482–491.
- Wambersie A, Hendry J, Gueulette J, et al. Radiobiological rationale and patient selection for high-LET radiation in cancer therapy. Radiother Oncol. 2004;73(2):S1–S14.
- Wambersie A, Richard F, Breteau N. Development of fast neutron therapy worldwide. Radiobiological, clinical and technical aspects. Acta Oncol. 1994;33(3):261–274.
- Denekamp J. Neutron radiobiology revisited. Acta Oncol. 1994;33(3):233–240.
- Vynckier S, Schmidt R. The physical basis for radiotherapy with neutrons. Recent Results Cancer Res. 1998;150:1–30.
- Laramore GE, Krall JM, Griffin TW, et al. Neutron versus photon irradiation for unresectable salivary gland tumors: final report of an RTOG-MRC randomized clinical trial. Radiation Therapy Oncology Group. Medical Research Council. Int J Radiat Oncol Biol Phys. 1993;27(2):235–240.
- van der Zee J, Vujaskovic Z, Kondo M, et al. The Kadota Fund International Forum 2004–clinical group consensus. Int J Hyperthermia. 2008;24(2):111–122.
- Dewhirst MW, Lee CT, Ashcraft KA. The future of biology in driving the field of hyperthermia. Int J Hyperthermia. 2016;32(1):4–13.
- Datta NR, Ordonez SG, Gaipl US, et al. Local hyperthermia combined with radiotherapy and-/or chemotherapy: recent advances and promises for the future. Cancer Treat Rev. 2015;41(9):742–753.
- Overgaard J. The heat is (still) on–the past and future of hyperthermic radiation oncology. Radiother Oncol. 2013;109(2):185–187.
- Peeken JC, Vaupel P, Combs SE. Integrating hyperthermia into modern radiation oncology: what evidence is necessary? Front Oncol. 2017;7:132.
- Datta NR, Puric E, Schneider R, et al. Could hyperthermia with proton therapy mimic carbon ion therapy? Exploring a thermo-radiobiological rationale. Int J Hyperthermia. 2014;30(7):524–530.
- Robinson JE, Wizenberg MJ, McCready WA. Combined hyperthermia and radiation suggest and alternative to heavy particle therapy for reduced oxygen enhancement ratios. Nature. 1974;251(5475):521–522.
- Horsman MR, Overgaard J. Hyperthermia: a potent enhancer of radiotherapy. Clin Oncol (R Coll Radiol). 2007;19(6):418–426.
- Repasky EA, Evans SS, Dewhirst MW. Temperature matters! And why it should matter to tumor immunologists. Cancer Immunol Res. 2013;1(4):210–216.
- Frey B, Weiss EM, Rubner Y, et al. Old and new facts about hyperthermia-induced modulations of the immune system. Int J Hyperthermia. 2012;28(6):528–542.
- Toraya-Brown S, Fiering S. Local tumour hyperthermia as immunotherapy for metastatic cancer. Int J Hyperthermia. 2014;30(8):531–539.
- Datta NR, Puric E, Klingbiel D, et al. Hyperthermia and radiation therapy in locoregional recurrent breast cancers: a systematic review and meta-analysis. Int J Radiat Oncol Biol Phys. 2016;94(5):1073–1087.
- Datta NR, Rogers S, Klingbiel D, et al. Hyperthermia and radiotherapy with or without chemotherapy in locally advanced cervical cancer: a systematic review with conventional and network meta-analyses. Int J Hyperthermia. 2016;32(7):809–821.
- Datta NR, Rogers S, Ordonez SG, et al. Hyperthermia and radiotherapy in the management of head and neck cancers: a systematic review and meta-analysis. Int J Hyperthermia. 2016;32(1):31–40.
- Datta NR, Bodis S. Hyperthermia with radiotherapy reduces tumour alpha/beta: insights from trials of thermoradiotherapy vs radiotherapy alone. Radiother Oncol. 2019;138:1–8.
- Issels RD, Lindner LH, Verweij J, et al. Effect of Neoadjuvant chemotherapy plus regional hyperthermia on long-term outcomes among patients with localized high-risk soft tissue sarcoma: the EORTC 62961-ESHO 95 randomized clinical trial. JAMA Oncol. 2018;4(4):483–492.
- Emami B, Song CW. Physiological mechanisms in hyperthermia: a review. Int J Radiat Oncol Biol Phys. 1984;10(2):289–295.
- Vaupel PW, Kelleher DK. Pathophysiological and vascular characteristics of tumours and their importance for hyperthermia: heterogeneity is the key issue. Int J Hyperthermia. 2010;26(3):211–223.
- Sun X, Xing L, Ling CC, et al. The effect of mild temperature hyperthermia on tumour hypoxia and blood perfusion: relevance for radiotherapy, vascular targeting and imaging. Int J Hyperthermia. 2010;26(3):224–231.
- Elming PB, Sorensen BS, Oei AL, Franken NAP, et al. Hyperthermia: the optimal treatment to overcome radiation resistant hypoxia. Cancers (Basel). 2019;11(1):60.
- Leith JT, Miller RC, Gerner EW, et al. Hyperthermic potentiation: biological aspects and applications to radiation therapy. Cancer. 1977;39(S2):766–779.
- Connor WG, Gerner EW, Miller RC, et al. Prospects for hyperthermia in human cancer therapy. Part II: implications of biological and physical data for applications of hyperthermia to man. Radiology. 1977;123(2):497–503.
- Gerner EW, Leith JT. Interaction of hyperthermia with radiations of different linerar energy transfer. Int J Radiat Biol Relat Stud Phys Chem Med. 1977;31(3):283–288.
- Gerner EW, Leith JT, Boone ML. Mammalian cell survival response following irradiation with 4 MeV X rays or accelerated helium ions combined with hyperthermia. Radiology. 1976;119(3):715–720.
- Maeda J, Fujii Y, Fujisawa H, et al. Hyperthermia-induced radiosensitization in CHO wild-type, NHEJ repair mutant and HR repair mutant following proton and carbon-ion exposure. Oncol Lett. 2015;10(5):2828–2834.
- Franken NA, Oei AL, Kok HP, et al. Cell survival and radiosensitisation: modulation of the linear and quadratic parameters of the LQ model (Review). Int J Oncol. 2013;42(5):1501–1515.
- Oei AL, Vriend LE, Crezee J, et al. Effects of hyperthermia on DNA repair pathways: one treatment to inhibit them all. Radiat Oncol. 2015;10(1):165.
- Furusawa Y, Fukutsu K, Aoki M, et al. Inactivation of aerobic and hypoxic cells from three different cell lines by accelerated (3)He-, (12)C- and (20)Ne-ion beams. Radiat Res. 2000;154(5):485–496.2.0.CO;2]
- Hahn EW, Canada TR, Alfieri AA, et al. The interaction of hyperthermia with fast neutrons or x rays on local tumor response. Radiat Res. 1976;68(1):39–56.
- van der Zee J, Gonzalez Gonzalez D, van Rhoon GC, et al. Comparison of radiotherapy alone with radiotherapy plus hyperthermia in locally advanced pelvic tumours: a prospective, randomised, multicentre trial. Dutch Deep Hyperthermia Group. Lancet. 2000;355(9210):1119–1125.
- Wust P, Hildebrandt B, Sreenivasa G, et al. Hyperthermia in combined treatment of cancer. Lancet Oncol. 2002;3(8):487–497.
- Datta NR, Stutz E, Puric E, et al. A pilot study of radiotherapy and local hyperthermia in elderly patients with muscle-invasive bladder cancers unfit for definitive surgery or chemoradiotherapy. Front Oncol. 2019;9:889.
- Maluta S, Dall’oglio S, Romano M, et al. Conformal radiotherapy plus local hyperthermia in patients affected by locally advanced high risk prostate cancer: preliminary results of a prospective phase II study. Int J Hyperthermia. 2007;23(5):451–456.
- Vernon CC, Hand JW, Field SB, et al. Radiotherapy with or without hyperthermia in the treatment of superficial localized breast cancer: results from five randomized controlled trials. International Collaborative Hyperthermia Group. Int J Radiat Oncol Biol Phys. 1996;35:731–744.
- Datta NR, Schneider R, Puric E, et al. Proton irradiation with hyperthermia in unresectable soft tissue sarcoma. Int J Particle Ther. 2016;3(2):327–336.
- Tran S, Puric E, Walser M, et al. Early results and volumetric analysis after spot-scanning proton therapy with concomitant hyperthermia in large inoperable sacral chordomas. Br J Radiol. 2019;92:20180883.
- Overgaard J, Gonzalez Gonzalez D, Hulshof MC, et al. Randomised trial of hyperthermia as adjuvant to radiotherapy for recurrent or metastatic malignant melanoma. European society for hyperthermic oncology. Lancet. 1995;345(8949):540–543.
- Dewhirst MW, Sim DA. The utility of thermal dose as a predictor of tumor and normal tissue responses to combined radiation and hyperthermia. Cancer Res. 1984;44(10):4772s–4780s.
- Emami B, Scott C, Perez CA, et al. Phase III study of interstitial thermoradiotherapy compared with interstitial radiotherapy alone in the treatment of recurrent or persistent human tumors. A prospectively controlled randomized study by the Radiation Therapy Group. Int J Radiat Oncol Biol Phys. 1996;34(5):1097–1104.