Abstract
Purpose
Hyperthermia demonstrated clinical efficacy in multimodal cancer treatment. Multipotent mesenchymal stromal cells (MSCs) as part of the tumor-supporting stroma modulate tumor response and tissue regeneration after hyperthermia. We aimed to investigate the effects of hyperthermia on the survival, stem cell characteristics and heat shock expression of human MSCs.
Materials and methods
Human MSCs and normal human dermal fibroblasts (NHDFs) were exposed to temperatures between 37 °C and 44 °C for 60 min, and hyperthermic sensitivity was examined by clonogenicity, proliferation and viability assays. The influence of 42 °C hyperthermia on the MSCs’ adhesion potential, migratory capacity, surface marker expression and multi-lineage differentiation capability was investigated. Cell cycle distribution, apoptosis and senescence after 42 °C hyperthermia were determined by flow cytometry and β-galactosidase staining. Heat shock protein expression was determined by Western Blots.
Results
MSCs exhibited decreased clonogenic survival after 40 °C and 42 °C hyperthermia compared to NHDFs, while proliferative activity and viability were comparable after hyperthermia up to 44 °C. MSC adhesion was reduced after 42 °C hyperthermia, while the characteristic surface marker expression and the migratory ability remained unaffected in 42 °C hyperthermia-exposed MSCs. 42 °C hyperthermia diminished the adipogenic differential potential of all tested MSC samples. A pronounced G2/M arrest was found after 42 °C hyperthermia and was associated with increased apoptosis and senescence levels in MSCs. MSCs exhibited slightly lower heat shock protein levels compared to NHDFs.
Conclusion
Human MSCs exhibit a thermosensitive phenotype which reduced the multipotent cells’ regenerative abilities, resulting in impaired tissue regeneration after hyperthermia treatment or thermal injuries. On the other hand, tumor-associated MSCs may be efficiently targeted by hyperthermia treatment.
Introduction
Hyperthermia treatment has been successfully introduced into the treatment algorithms for several malignancies such as recurrent breast cancer and soft-tissue sarcoma, and is commonly combined with radiotherapy or chemotherapy [Citation1–5]. Besides its direct cytotoxic effects, hyperthermia acts as a sensitizer for radiotherapy and chemotherapy and demonstrated supraadditive effects in vitro and in vivo. Hyperthermia can lead to increased tumor perfusion and thereby higher tissue oxygen levels [Citation6–8], resulting in improved chemotherapeutic drug delivery and enhanced radiation damage due to the oxygen enhancement effect [Citation9]. Furthermore, hyperthermia may exert its anti-tumor effects via immunomodulation including induction of major histocompatibility complex I (MHCI) molecules, release of immunogenic heat shock proteins (HSPs) and improved penetration of immune cells due to increased vascular permeability [Citation10].
Multipotent mesenchymal stromal cells (MSCs) were first described by Friedenstein and colleagues in bone marrow samples, but have since been isolated from many other tissues such as adipose tissue, thymus, placenta and umbilical cord [Citation11–16]. Hyperthermia-exposed MSCs have shown to suppress ovarian and breast cancer growth in vitro [Citation17,Citation18]. On the other hand, tumor-associated MSCs were observed to protect ovarian cancer from hyperthermia via the CXCL12/CXCR4-axis [Citation19]. Furthermore, heat-exposed MSCs exhibited reduced inhibitory effects on lymphocytes compared to untreated MSCs [Citation20]. Besides the stem cells’ potential role in tumor protection after hyperthermia, MSCs have shown to play a role in the regeneration of hyperthermia-treated normal tissues. This may be crucial as combination therapies consisting of re-irradiation and hyperthermia for recurrent breast cancer have demonstrated increased levels of higher-grade acute toxicities such as dermatitis and ulceration in up to a third of patients [Citation21]. Due to the MSCs’ tropism toward injured tissue, endogenous MSCs have the potential to attenuate skin toxicity caused by irradiation and hyperthermia [Citation22,Citation23]. However, the influence of hyperthermia treatment on MSCs is almost completely unknown.
In this study, we thoroughly examined the effects of hyperthermia exposure on the survival, stem cell characteristics and functional abilities on human multipotent MSCs in comparison with normal human dermal fibroblasts (NHDFs). In addition, underlying mechanisms for the different thermal response between MSCs and NHDFs were investigated.
Materials and methods
Cells and cell culture
MSCs were harvested from bone marrow of three healthy volunteers as previously described [Citation24]. MSCs were grown in StemMACS™ MSC Expansion Media (Miltenyi Biotec, Bergisch-Gladbach, Germany). NHDFs were purchased from the ATCC and cultured in Dulbecco’s Modified Eagle Medium (Biochrom, Berlin, Germany) supplemented with 10% fetal bovine serum. Written consent was obtained prior to isolation, and this investigation was approved by the Heidelberg University Ethics Committee (#S-384/2004).
Hyperthermia
Hyperthermia treatment was performed in a temperature circulator-coupled water bath. Multiwell plates and culture flasks were placed in the water bath for 70 min. This exposure time was chosen to guarantee hyperthermia treatment for 60 min with the required temperature, as the average temperature inside the wells and culture flasks needed 10 min to reach its target value. Temperature inside the wells was controlled using a digital thermometer (Testo 735-1, Testo GmbH & Co., Lanzkirch, Germany).
Clonogenic survival, proliferation and viability assays
In order to investigate the reproductive ability of MSCs and NHDFs after hyperthermia treatment, clonogenic survival assays were performed: Cells were plated in 6-well plates and allowed to attach 24 h prior to hyperthermia exposure with various temperatures ranging between 37 °C and 44 °C with an exposure time of 60 min. At 9 days after treatment, colonies were stained with crystal violet/methanol solution, and colonies consisting of 50 or more cells were counted. Cellular survival fraction was calculated according to the following formula: (#colonies/#plated cells)treated/(#colonies/#plated cells)untreated. Three biological triplicates were conducted for all clonogenic survival assays.
To assess proliferation, cells were plated in 6-well plates 24 h before hyperthermia exposure and afterwards proliferated for 96 h. Cells were harvested and stained with 0.4% trypan blue solution, before viable cells were counted using a Neubauer chamber. Numbers of viable cells were normalized to untreated control samples. Proliferation assays were carried out with three biological replicates containing three technical replicates each.
Cellular viability at 96 h after hyperthermia was assessed by resazurin assays. At 24 h before heating, 2 × 103 cells/well were plated in 96-well plates and treated with temperatures ranging between 37 °C and 44 °C for 60 min. At 96 h after hyperthermia exposure, 20 µL 0.3 mg/mL resazurin (Sigma, Steinheim, Germany) was added to each well containing 100 µL cell culture medium and incubated for 4 h prior to colorimetric analyses by measuring light absorbance at 570 nm and 600 nm with a VersaMax microplate reader (Molecular Devices, LLC, Sunnyvale, CA, USA). Background absorbance at 600 nm was subtracted from absorbance at 570 nm.
Cell adhesion measurements
For cellular adhesion measurements, MSCs and NHDFs were seeded in T25 flasks at 24 h before hyperthermia treatment. Hyperthermia treatment with 37 °C and 42 °C lasted 60 min. Immediately after heating, cells were dissociated using Trypsin-EDTA (0.5%), and 100 cells/well were seeded in 96-well plates. Number of attached cells was determined at different time points after hyperthermia. For cell counting, medium with non-attached cells was removed and attached cells were stained with 1 µg/mL DAPI. Fluorescence images were obtained using an Operetta CLS High-Content Analysis System (PerkinElmer, Waltham, MA, USA), and the number of attached cells was assessed automatically with the CLS Harmony® high-content imaging and analysis software (PerkinElmer).
Cell migration analyses
Migratory ability of human MSCs and NHDFs was analyzed by time-lapse microscopy. Cells were plated in T25 flasks 24 h before heating and exposed to the target temperature of 42 °C for 60 min. Immediately after hyperthermia treatment, 4 × 103cells/well were plated in 24-well plates. Long-term live cell imaging was performed using an Incucyte S3 (Essen BioScience, Ann Arbor, MI, USA) fitted with an incubator box at 37 °C and 5% CO2. ImageJ software (National Institutes of Health, Bethesda, MD, USA) was used for manual single-cell tracking, and tracking was performed for at least 30 cells/well from three randomly chosen fields-of-view for 24 h.
Surface marker expression analyses
MSCs were plated in T75 flasks and allowed to attach for 24 h prior to hyperthermia treatment with 42 °C for 60 min. At 96 h after hyperthermia, surface marker expression was measured on a FACSVerse™ flow cytometer (BD Biosciences, San Jose, CA, USA). Surface marker staining were executed with a MSC phenotyping kit (Miltenyi Biotec) according to the manufacturer’s instructions, and 10,000 events were recorded for each treatment condition. For each measurement, unstained controls and isotype controls were used. Data analysis was performed with FlowJo™ v10.6.1 software (FlowJo LLC, Ashland, OR, USA).
Cell morphology analyses
MSCs and NHDFs were plated in 6-well plates 24 h prior to hyperthermia treatment with 42 °C for 60 min. At 96 h after hyperthermia treatment, cells were fixed using 3% paraformaldehyde (PFA)/phosphate-buffered saline (PBS). Representative phase contrast images were taken with an Olympus IX51 inverted microscope (Olympus, Center Valley, PA, USA) using a 10× objective.
MSC differentiation capability
In order to assess the multi-lineage differentiation capability of MSCs after hyperthermia treatment, 3 × 104 cells were plated in 24-well plates and exposed to 37 °C, 40 °C or 42 °C for 60 min. 24 h later, culturing medium was replaced with differentiation media, and cells were allowed to differentiate for 14–21 days. Adipogenic differentiation was induced using StemMACS™ AdipoDiff Media (Miltenyi Biotec), and differentiated cells were incubated in 1 µM/mL BODIPY® 493/503 solution for 20 min prior to nuclei staining with 1 µg/mL DAPI/PBS. For osteogenic differentiation, StemMACS™ OsteoDiff Media (Miltenyi Biotec) was used, and hydroxyapatite levels as a specific parameter for osteogenic differentiation were quantified with OsteoImageTM Staining Reagent (Lonza, Basel, Switzerland) as described in the manufacturer’s instructions.
For chondrogenic differentiation, MSC spheroids were created by seeding 1 × 105 MSCs/well in a 96-well plate with a U-bottom shape (Greiner, Frickenhausen, Germany). 24 h afterwards, spheroids were exposed to 37 °C, 40 °C or 42 °C, and culturing medium was replaced with StemMACSTM ChondroDiff Media (Miltenyi Biotech). At 21 days after hyperthermia therapy, spheroids were fixed with 4% PFA/PBS solution and frozen at −20 °C prior to sectioning on a cryomicrotome. For aggrecan staining, sections were incubated with 0.3% Triton X-100, 1% bovine serum albumin (BSA) and 10% donkey serum in PBS followed by incubation with the primary antibody against human aggrecan (1:10, R&D Systems, Minneapolis, MN, USA) and an Alexa488-coupled secondary donkey-anti-goat antibody (1:200, Abcam, Cambridge, UK).
Fluorescence staining were visualized on a Keyence BioRevo9000 microscope (Keyence, Neu-Isenburg, Germany), and staining intensities were quantified using ImageJ software.
Cell cycle and apoptosis measurements
Both MSCs and NHDFs were plated in T25 flasks 24 h before hyperthermia treatment and were exposed to 37 °C and 42 °C for 60 min. At 24, 48 and 96 h after treatment, cells were harvested, fixed in 3% PFA/PBS solution and permeabilized in ice-cold 70% ethanol. After several washing steps with 0.5% BSA/PBS, samples were incubated with an Alexa647-coupled antibody against activated caspase-3 (1:20, BD Pharmingen, Heidelberg, Germany) for 1 h at room temperature. After centrifugation with 200 g for 5 min, specimens were incubated in 1 μg/mL DAPI/PBS solution. Measurements were performed on a BD FACSVerse™ flow cytometer, and 10,000 events were counted for each specimen. Cell cycle and apoptosis analyses were carried out using FlowJo™ v10.6.1 software (FlowJo LLC).
Senescence analyses
2 × 103 cells/well were plated on glass cover slips in 24-well plates at 24 h prior to heating. Adherent cells were exposed to 37 °C and 42 °C for 60 min. At 48 and 96 h after treatment, specimens were fixed and stained using the Senescence β-Galactosidase Staining Kit (Cell Signaling Technology, Leiden, Netherlands) according to the manufacturer’s instructions. Nuclei were counterstained with 1 µg/mL DAPI, and images were taken on a Keyence BioRevo9000 microscope. The percentage of β-galactosidase-positive cells was assessed using ImageJ software.
Western blots
5 × 105 MSCs or NHDFs were seeded in T75 flasks 24 h prior to 42 °C hyperthermia treatment for 60 min. 24 h after hyperthermia, cells were harvested and incubated in RIPA buffer for 20 min on ice. Protein samples were run on 4–15% precast polyacrylamide gels (Bio-Rad, Hercules, CA, USA) and transferred to polyvinylidenedifluoride membranes (Millipore, Darmstadt, Germany). After blotting, membranes were incubated with a mouse antibody against HSP27 (1:1000, Cell Signaling Technology), a rabbit antibody against HSP60 (1:1000, Cell Signaling Technology), a mouse antibody against heat shock protein HSP70 (1:1000, LifeSpan Biosciences, Seattle, WA, USA), a rat antibody against heat shock transcription factor HSF1 (1:1000, Cell Signaling Technology) and a rabbit antibody against β-actin (1:1000; Cell Signaling Technology). Membranes were then incubated with HRP (horseradish peroxidase)-conjugated secondary antibodies (1:5000, Jackson ImmunoResearch, West Grove, PA, USA). Blots were visualized on X-ray films with a Luminol-based enhanced chemiluminescence HRP substrate (Thermo Scientific™ SuperSignal™ West Dura Chemiluminescent Substrate).
Results
MSCs lose their clonogenic ability but remain viable after hyperthermia treatment
Hyperthermia sensitivity of human MSCs and NHDFs was determined by clonogenic survival, proliferation and viability assays. Both the treatment doses and the exposure time were chosen in order to mimic clinical hyperthermia therapy in cancer treatment [Citation25].
Clonogenic survival of MSCs was significantly reduced compared to NHDFs after treatment with 40 °C and 42 °C (40 °C: p < 0.05 for MSC1, p < 0.001 for MSC2 and MSC3; 42 °C: p < 0.001 for MSC1, MSC2 and MSC3) (). Hyperthermia with 42 °C resulted in a clonogenic survival rate of 83.1% in NHDF cells, while the stem cells’ clonogenic survival ranged between 10.1% (MSC3) and 24.9% (MSC2). However, colony forming was almost completely abolished after 44 °C hyperthermia for both MSCs and NHDFs. In terms of proliferation and metabolic viability after hyperthermia, MSCs and NHDF cells showed comparable results. Heat exposure with 40 °C resulted in non-significantly reduced proliferation levels of MSC1 (65.4%) compared to NHDFs (77.1%) (p = 0.39), while MSC2 and MSC3 showed comparable proliferation levels (MSC2: 70.9%, MSC3: 76.5%) to NHDFs (). Metabolic viability of MSCs and NHDFs was investigated 96 h after hyperthermia treatment using resazurin assays. Temperatures up to 42 °C did not result in reduced viability of both MSCs and NHDFs, suggesting that hyperthermia rather reduces the cells’ ability to undergo multiple cell divisions than impairing mitochondrial respiration (). All tested MSC samples exhibited significantly lower viability levels than NHDFs after hyperthermia with 40 °C (p < 0.001), and MSC1 and MSC3 cells were found to have reduced metabolic viability levels in comparison with NHDFs after exposure to 42 °C (p < 0.01 for MSC1, p < 0.001 for MSC3).
Figure 1. MSCs lose their proliferative ability but remain viable after hyperthermia. (A) Clonogenic survival assays for human MSCs and NHDFs. (B) Proliferation at 96 h after hyperthermia therapy of MSCs and NHDFs. (C) Resazurin assays at 96 h after hyperthermia. *p < 0.05, ***p < 0.001. Data are presented as mean ± standard deviation.
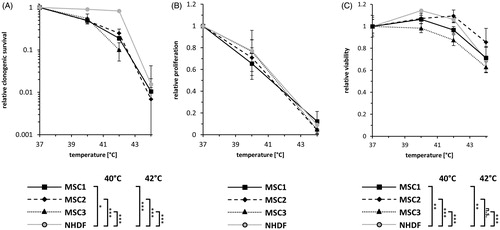
Hyperthermia results in delayed cellular adhesion but not in impaired velocity of MSCs
The ability to adhere to plastic surfaces belongs to the defining stem cell characteristics of MSCs [Citation26]. While MSC1 cells did not show impaired attachment after hyperthermia, both MSC2 and MSC3 were observed to exhibit lower attachment rates at 30 min after exposure to 42 °C compared to untreated controls (p < 0.05 after 0.5 h for MSC3, p < 0.01 after 0.5 h for MSC2) (). At later time points, hyperthermia-exposed MSC2 and MSC3 cells were still found to have reduced levels of attached cells (p < 0.05 after 4 h for MSC2, p < 0.01 after 4 h for MSC3). In contrast to MSCs, hyperthermia-treated NHDFs did not demonstrate reduced levels of cellular attachment and exhibited even more attached cells at 4 h after 42 °C (p < 0.01).
Figure 2. MSCs show delayed cellular adhesion but unaltered velocity after hyperthermia. (A) Relative adhesion rate of MSCs and NHDFs after exposure to 37 °C or 42 °C for 60 min. (B) Average cellular motility of hyperthermia-exposed MSCs and NHDFs determined by time-lapse microscopy. Date are shown as mean ± standard deviation. *p < 0.05, **p < 0.01, ***p < 0.001.
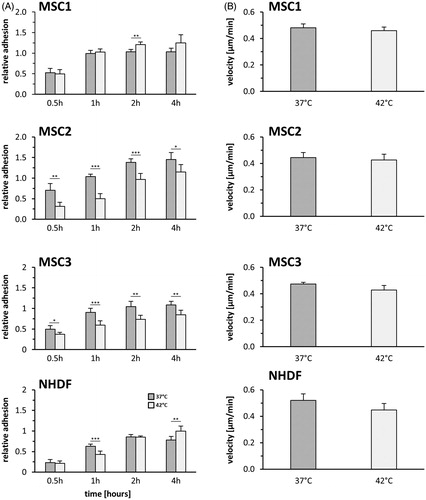
Single-cell tracking using time-lapse microscopy was performed to determine cellular velocity after hyperthermia. Average cellular velocity of individual untreated MSC specimens was comparable and ranged between 0.44 µm/min for MSC2 and 0.48 µm/min for MSC1 (). The average velocity of MSC1, MSC3 and NHDFs was found unaltered after exposure to 42 °C (p = 0.41 for MSC1, p = 0.60 for MSC3, p = 0.21 for NHDF). Only MSC3 cells showed a non-significant trend toward reduced cellular movement (p = 0.10).
Hyperthermia does not abrogate the characteristic surface marker expression of MSCs
MSCs exhibit a characteristic surface marker pattern consisting of positive and negative markers [Citation26]. The expression of defining surface proteins was investigated using flow cytometry (). Untreated MSCs exhibited strong expression of CD73, CD90 and CD105 and were shown to lack expression of the hematopoietic markers CD14, CD20, CD34 and CD45. The surface marker expression was observed unaltered after hyperthermia treatment with up to 42 °C in MSCs. Negative markers were found to remain unexpressed in all tested MSC specimens at 96 h after hyperthermia.
Figure 3. MSCs largely retain their characteristic surface marker pattern but show morphological alterations after hyperthermia. (A) Flow cytometry analyses of positive (CD73, CD90 and CD105) and negative (CD14, CD20, CD34 and CD45) MSC surface markers at 96 h after hyperthermia. (B) Representative phase contrast images showing cellular morphology of MSCs and NHDFs at 96 h after hyperthermia with 42 °C. 10× objective, scale bar 100 µm.
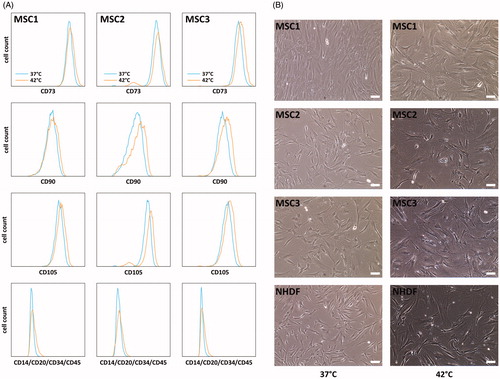
Morphology of hyperthermia-treated MSCs and NHDFs was observed altered at 96 h after exposure to 42 °C. Both MSCs and NHDFs showed enlarged cell bodies as well as increased numbers of vacuoles and membrane blebbing, demonstrating morphological signs of both senescence and apoptosis initiation ().
Hyperthermia reduces the adipogenic differentiation potential of MSCs
The ability to differentiate into adipocytes, osteocytes and chondrocytes is a characteristic stem cell feature of MSCs, and the differentiation of hyperthermia-treated MSCs was quantified using immunocytochemical staining [Citation26]. Hyperthermia with both 40 °C and 42 °C for 60 min was found to reduce adipogenic differentiation in all tested MSC preparations (p < 0.01 for 40 °C, p < 0.05 for 42 °C) (). In contrast, exposure to 42 °C led to increased osteogenic differentiation in MSC1 and MSC3 (p < 0.05) (). MSC2 cells showed increased osteogenic differentiation potential after 40 °C (p < 0.05) but not after 42 °C (p = 0.24). Chondrogenic differentiation potential of MSCs was largely unaffected after heat application, and only MSC3 cells exhibited decreased aggrecan levels as markers for chondrogenic differentiation both after 40 °C and 42 °C (p < 0.001 for 40 °C, p < 0.05 for 42 °C) ().
Figure 4. Hyperthermia reduces adipogenic differentiation but enhances osteogenic differentiation potential of MSCs. (A) Adipogenic differentiation after exposure to 37 °C, 40 °C and 42 °C quantified by BODIPY® 493/503 staining. 2× objective, scale bar 1000 µm. (B) OsteoImageTM staining for assessment of hydroxyapatite amount as marker for osteogenic differentiation after hyperthermia. 10× objective, scale bar 200 µm. (C) Chondrogenic differentiation after hyperthermia determined by aggrecan staining. 10× objective, scale bar 200 µm. *p < 0.05, **p < 0.01, ***p < 0.001.
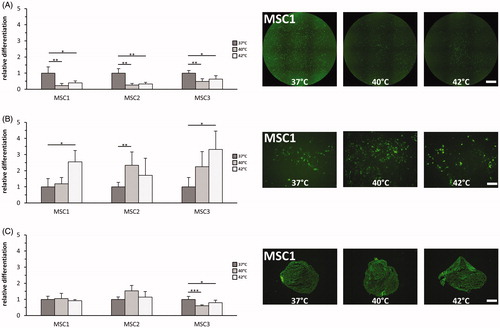
Hyperthermia results in a prolonged G2/M-phase accumulation
Cell cycle distribution after heat application with 37 °C and 42 °C was measured by flow cytometry over a time course of 96 h (). A 42 °C exposure resulted in a pronounced accumulation in the G2/M-phase at 24 h after hyperthermia in all tested MSC samples (p < 0.001), but not in NHDFs (p = 0.34). The G2/M-phase arrest remained constant at 48 and 96 h after treatment with hyperthermia and involved between 19.7% (MSC2) and 32.6% (MSC3) of cells (p < 0.001 for MSC1 and MSC3, p < 0.01 for MSC2 after 96 h). Additionally, the number of S-phase cells increased after heat exposure in all MSC preparations, beginning at 24 h after heat application and suggesting an additional hyperthermia-induced S-phase arrest (p < 0.01 for MSC1 and MSC2 after 96 h, p < 0.001 for MSC3 after 96 h).
Hyperthermia induces apoptosis in MSCs
As cleaved caspase-3 has a central role both in the extrinsic and intrinsic apoptosis pathway, percentage of cleaved caspase-3-positive cells was assessed by flow cytometry for quantification of cellular apoptosis as described previously [Citation27]. Hyperthermia resulted in considerably increased apoptosis rates for MSCs and NHDFs (): At 24 h after hyperthermia, both MSC1 and MSC3 exhibited elevated numbers of caspase-3-positive cells, with up to 10% apoptotic cells (p < 0.05 for MSC1, p < 0.01 for MSC3), while MSC2 showed a non-significant trend toward increased apoptosis (p = 0.06). At later time points, hyperthermia with 42 °C led to substantially further increases of caspase-3-positive cells with up to 49.3% apoptotic cells in MSC3 (p < 0.01). Similarly, MSC1 and MSC2 cells exhibited significantly increased apoptosis levels at 96 h after treatment with 27.4% (MSC1) and 7.5% (MSC2) apoptotic cells (p < 0.001 for MSC1, p < 0.05 for MSC2). The percentage of caspase-3-positive NHDFs was found to be comparable to untreated controls at 24 h after hyperthermia, while it increased at later time points with 36.1% apoptotic cells at 96 h after treatment (p < 0.05).
Figure 6. Hyperthermia leads to both increased apoptosis and senescence levels in MSCs. (A) Percentage of apoptotic MSCs and NHDFs determined by levels of activated caspase-3 at 24, 48 and 96 h after hyperthermia with up to 42 °C. (B) Percentage of senescent cells assessed by β-galactosidase staining at 48 and 96 h after hyperthermia. Representative images showing β-galactosidase staining of hyperthermia-treated MSCs and NHDFs. 10× objective, scale bar 200 µm. (C) Western Blot analyses of HSF1, HSP70, HSP60 and HSP27 at 24 h after hyperthermia treatment in MSCs and NHDFs. β-actin was used as loading control. *p < 0.05, **p < 0.01, ***p < 0.001.
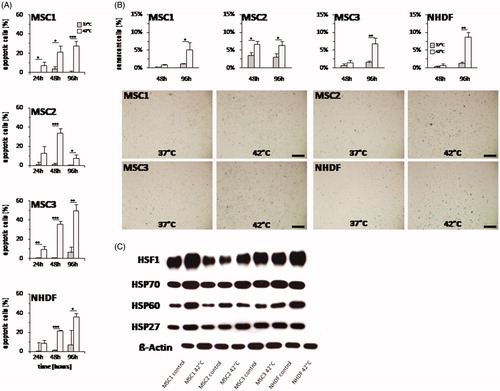
Heat application results in cellular senescence induction
Hyperthermia-induced senescence was assessed by β-galactosidase staining in MSCs and NHDFs. Compared to the percentage of apoptotic cells, senescence levels remained relatively low with a maximum of 10% senescent cells after exposure to 42 °C. At 48 h after hyperthermia, senescence levels were found to be slightly elevated only in MSC2 with 6.6% β-galactosidase-positive cells (p < 0.05) (). All tested MSC specimens showed increased numbers of β-galactosidase-positive cells at 96 h after heat application (p < 0.05 for MSC1 and MSC2, p < 0.01 for MSC3). NHDF fibroblasts showed a similar response with no increased senescence induction after 48 h, but after 96 h (p < 0.01).
MSCs express slightly lower levels of HSPs than NHDFs
Expression of the heat shock proteins HSP27, HSP60, HSP70 and the heat shock transcription factor HSF1 have been linked to the ability of cells to deal with thermal stress and were therefore investigated in our study [Citation28,Citation29]. NHDFs exhibited slightly higher expression levels of several heat shock proteins compared to MSCs (, Supplementary Figure S1). The differences in the expression levels were most prominent between MSC2 and NHDF cells. Especially for HSP60, hyperthermia treatment with 42 °C led to increased protein levels in comparison with untreated controls. MSC1 cells were found to exhibit the highest heat shock protein levels among the different MSC samples. Although MSCs had slightly lower heat shock protein levels than NHDFs, there was still a strong expression of these proteins compared to β-actin levels.
Discussion
Tumor-infiltrating MSCs are known to enhance the metastasis potential of cancer cells and to negatively influence the prognosis of cancer patients for various malignancies [Citation30–33]. On the other hand, MSCs may play a critical role in the regeneration of cancer treatment-related toxicities [Citation34,Citation35]. Hyperthermia in combination with chemo- or radiotherapy is an established treatment modality for several cancer entities and is able to target the tumor microenvironment and cancer stem cells [Citation1,Citation3,Citation25,Citation36]. However, the effects of therapeutic hyperthermia on the survival, stem cell characteristics and regenerative abilities of human primary MSCs are poorly understood.
In this study, we demonstrated that human primary MSCs exhibit a thermosensitive phenotype, as underlined by reduced clonogenic survival rates, impaired adipogenic differentiation and strongly increased apoptosis levels. Reduced proliferation and viability levels have been associated with decreased regenerative potential of MSCs in a wound healing model suggesting that our results indicate an impaired regenerative capacity of heat-exposed MSCs [Citation37].
Interestingly, cellular velocity of MSCs was found unaltered after therapeutic hyperthermia which is in contrast to osteosarcoma and pancreatic cancer cell lines exhibiting reduced motility after hyperthermia [Citation38,Citation39]. However, sub-lethal hyperthermia significantly enhanced cell motility of breast cancer cells [Citation40]. Although average motility of MSCs was unaffected, directed migration toward injured normal tissues may be hampered. The SDF-1/CXCR4-axis is known to be crucial for the migration of MSCs toward both injured lesions and tumors, and SDF-1 was found to contribute to the thermoprotective ability of MSCs for ovarian cancer [Citation19,Citation41].
Hyperthermia resulted in a long-lasting G2/M-phase accumulation of MSCs which was more pronounced in MSCs than in NHDFs. Similarly, ionizing radiation, ultraviolet radiation and various chemotherapeutic compounds have been demonstrated to cause a G2/M-phase block in MSCs [Citation42–45]. Hyperthermia rather activates the ATR/Chk1- than the ATM/Chk2-pathway, leading to a G2/M-phase arrest of heat-exposed cells [Citation46]. Furthermore, hyperthermia-induced damage to the DNA replication machinery may arrest cells in the S-phase, potentially explaining the increased percentage of S-phase cells after heat exposure of MSCs [Citation47]. The hyperthermia-induced G2/M-phase arrest may contribute to a radiosensitization of MSCs, as cells are more radiosensitive in the G2- and especially in the M-phase of the cell cycle [Citation48]. Indeed, work from our group revealed a potent radiosensitization of MSCs after pretreatment with 40 °C (data not shown). MSCs are known to evade apoptosis after chemo- or radiotherapy due to efficient DNA repair and high expression of anti-apoptotic proteins [Citation49–52]. In contrast, hyperthermic treatment had pronounced pro-apoptotic effects on MSCs leading to almost 50% caspase-3-positive cells after exposure to 42 °C. Previous studies have reported varying apoptosis rates after hyperthermia depending on the cell and tissue type [Citation53]. Hyperthermia has been reported to cause apoptosis induction both via the intrinsic and via the extrinsic pathway, while heat stress-related production of reactive oxygen species further modulates apoptosis induction [Citation54]. Consistent with our results, whole body hyperthermia was shown to result in increased apoptosis rates in bone marrow samples associated with marrow hypocellularity, while other normal tissues such as heart, lung, kidney or liver did not exhibit elevated apoptosis levels after whole body hyperthermia [Citation55].
The increased levels of β-galactosidase-positive MSCs as a surrogate for increased senescence after heat application were consistent with the altered morphology of heat-exposed MSCs showing also signs of premature senescence such as enlargement and flattening. However, senescence levels after thermal exposure remained relatively low in our study with senescence values not exceeding 10%. In contrast to our results, other groups have reported higher levels of premature senescence following hyperthermia [Citation56]. However, different hyperthermia treatment protocols with different temperatures and shorter heat exposure times ranging between 10 and 30 min were used which could explain the different results [Citation56].
The observed increases of osteogenic differentiation in hyperthermia-treated MSCs are in line with previous reports which revealed enhanced osteogenic differentiation ability after periodic hyperthermia with 41 °C [Citation57]. A recent study could show that hyperthermia-induced upregulation of HSP70 contributes to increased osteogenic differentiation of MSCs, as functional knock-down of HSP70 abolished the increased osteogenic differentiation capacity of hyperthermia-treated MSCs [Citation58]. However, the exact mechanisms for an increased osteogenic differentiation potential due to hyperthermia-related upregulation of HSP70 are not well understood. HSP70-induced activation of the ERK pathway was reported to result in increased expression of genes involved in osteogenesis such as Runx2 and osterix [Citation59]. Other reports have demonstrated increased chondrogenic differentiation after periodic hyperthermia which was not observed in our analyses with a single hyperthermia treatment [Citation60]. Our hyperthermia protocol was chosen to mimic clinical protocols in which radiotherapy and water-filtered infra-red-A hyperthermia were combined, especially for the treatment of recurrent locoregional recurrent breast cancer [Citation4,Citation25,Citation61].
As increased expression of heat shock proteins has been linked to cellular thermoresistance, we investigated the expression of several heat shock proteins and the heat shock transcription factor HSF1 before and after hyperthermia. In our analysis, MSCs exhibited slightly lower basal levels of HSP27, HSP60 and HSP70 compared to NHDFs which could partly explain the observed thermosensitive phenotype of MSCs, as these proteins play a major role in the heat shock response pathway. Especially MSC2 cells were found to have clearly reduced levels of HSF1 and HSP60, whereby MSC1 had similar levels compared with NHDFs. Heat shock proteins exhibit anti-apoptotic abilities by blocking both the intrinsic and extrinsic apoptotic pathways, and increased levels of HSF1 have been shown to inhibit autophagy induction after hyperthermia [Citation62,Citation63]. We observed only slightly increased levels of heat shock proteins at 24 h after hyperthermia. This marginal increase could be related to the short time interval, as in other studies, protein expression of HSP27, HSP70 and HSP90 reached its maximum in MSCs at 48 h after hyperthermia [Citation64,Citation65]. Although the levels of heat shock proteins were higher for NHDFs than for MSCs, it should be noticed that the expression of these proteins were markedly higher than the β-actin protein levels for all tested samples, which is consistent with previous reports showing a high expression of heat shock proteins in MSCs [Citation50].
Hyperthermia therapy has been investigated for many malignancies including glioblastoma, head-and-neck cancers, breast cancer or prostate carcinoma [Citation25,Citation66–68]. For all these tumors, tumor-infiltrating MSCs may promote tumor growth and worsen survival of patients [Citation30–33]. Based on the cell survival assays in our study, therapeutic hyperthermia with up to 44 °C for 1 h could efficiently reduce tumor-associated MSCs and thereby improve the anti-tumor effect of hyperthermia. Additionally, as tumor-associated MSCs have been known to support an immunosuppressive microenvironment inside tumors, the immunogenic effects of hyperthermia such as increased MHCI expression and release of immunogenic HSPs may reduce the MSC-mediated immunosuppression [Citation10,Citation69].
On the other hand, our results may also have clinical relevance regarding MSC-based treatments for burn injuries, as allogeneic MSCs for severe non-healing burn wounds have resulted in promising results both in animal studies and in case reports involving human patients [Citation70,Citation71]. In vivo studies could demonstrate that intravenously administrated MSCs migrated into burn wounds and supported the regeneration by decreasing the amount of infiltrating inflammatory cells and inflammatory cytokines and by improving neovascularization through increased vascular endothelial growth factor levels [Citation71]. Interestingly, a clinical report also provided evidence that MSCs reduced burn wound infection due to the anti-infective properties of MSCs [Citation70]. Our results suggest that for treatment of burn injuries, treatments using non-exposed allogeneic MSCs may be superior to endogenously mobilized heat-exposed MSCs.
In summary, we could demonstrate that human MSCs are relatively sensitive to hyperthermia shown by strongly reduced clonogenicity and increased apoptosis rates after therapeutic hyperthermia. Lower protein expression of several heat shock proteins compared to NHDFs may at least in part explain the observed thermosensitivity of MSCs. Our results should be taken into consideration when planning studies regarding MSC-based treatments for hyperthermia-related normal tissue injuries such as skin ulceration after burn injuries. On the other side, tumor-infiltrating and supporting MSCs may be efficiently eradicated by hyperthermia as part of multimodal cancer treatment.
Supplemental Figure 1
Download TIFF Image (235.2 KB)Acknowledgements
The authors thank Elke Firat, Simone Gaedicke, Elena Guffart and Christine Aldrian for helping with flow cytometry, Western Blots and hyperthermia treatment.
Disclosure statement
No potential conflict of interest was reported by the author(s).
Additional information
Funding
References
- Perez CA, Pajak T, Emami B, et al. Randomized phase III study comparing irradiation and hyperthermia with irradiation alone in superficial measurable tumors. Final report by the Radiation Therapy Oncology Group. Am J Clin Oncol. 1991;14(2):133–141.
- Vernon CC, Hand JW, Field SB, et al. Radiotherapy with or without hyperthermia in the treatment of superficial localized breast cancer: results from five randomized controlled trials. International Collaborative Hyperthermia Group. Int J Radiat Oncol Biol Phys. 1996;35(4):731–744.
- Issels RD, Lindner LH, Verweij J, et al. Neo-adjuvant chemotherapy alone or with regional hyperthermia for localised high-risk soft-tissue sarcoma: a randomised phase 3 multicentre study. Lancet Oncol. 2010;11(6):561–570.
- Datta NR, Puric E, Klingbiel D, et al. Hyperthermia and radiation therapy in locoregional recurrent breast cancers: a systematic review and meta-analysis. Int J Radiat Oncol Biol Phys. 2016;94(5):1073–1087.
- Notter M, Thomsen AR, Nitsche M, et al. Combined wIRA-hyperthermia and hypofractionated re-irradiation in the treatment of locally recurrent breast cancer: evaluation of therapeutic outcome based on a novel size classification. Cancers. 2020;12(3):606.
- Bicher HI, Hetzel FW, Sandhu TS, et al. Effects of hyperthermia on normal and tumor microenvironment. Radiology. 1980;137(2):523–530.
- Vaupel P, Müller-Klieser W, Otte J, et al. Impact of various thermal doses on the oxygenation and blood flow in malignant tumors upon localized hyperthermia. In: Lübbers DW, Acker H, Leniger-Follert E, et al., editors. Oxygen transport to tissue-V. Boston, MA: Springer US; 1984. p. 621–629.
- Song CW, Shakil A, Osborn JL, et al. Tumour oxygenation is increased by hyperthermia at mild temperatures. Int J Hyperthermia. 1996;12(3):367–373.
- Gray LH, Conger AD, Ebert M, et al. The concentration of oxygen dissolved in tissues at the time of irradiation as a factor in radiotherapy. Br J Radiol. 1953;26(312):638–648.
- Toraya-Brown S, Fiering S. Local tumour hyperthermia as immunotherapy for metastatic cancer. Int J Hyperthermia. 2014;30(8):531–539.
- Zuk PA, Zhu M, Ashjian P, et al. Human adipose tissue is a source of multipotent stem cells. Mol Biol Cell. 2002;13(12):4279–4295.
- Rzhaninova AA, Gornostaeva SN, Goldshtein DV. Isolation and phenotypical characterization of mesenchymal stem cells from human fetal thymus. Bull Exp Biol Med. 2005;139(1):134–140.
- In 't Anker PS, Scherjon SA, Kleijburg-van der Keur C, et al. Isolation of mesenchymal stem cells of fetal or maternal origin from human placenta. Stem Cells. 2004;22(7):1338–1345.
- Bieback K, Kern S, Kluter H, et al. Critical parameters for the isolation of mesenchymal stem cells from umbilical cord blood. Stem Cells. 2004;22(4):625–634.
- Friedenstein A, Chailakhjan R, Lalykina K. The development of fibroblast colonies in monolayer cultures of guinea‐pig bone marrow and spleen cells. Cell Tissue Kinet. 1970;3(4):393–403.
- Friedenstein AJ, Petrakova KV, Kurolesova AI, et al. Heterotopic of bone marrow. Analysis of precursor cells for osteogenic and hematopoietic tissues. Transplantation. 1968;6(2):230–247.
- Park H, Cho JA, Kim SK, et al. Hyperthermia on mesenchymal stem cells (MSCs) can sensitize tumor cells to undergo cell death. Int J Hyperthermia. 2008;24(8):638–648.
- Cho JA, Park H, Kim HK, et al. Hyperthermia-treated mesenchymal stem cells exert antitumor effects on human carcinoma cell line. Cancer. 2009;115(2):311–323.
- Lis R, Touboul C, Mirshahi P, et al. Tumor associated mesenchymal stem cells protects ovarian cancer cells from hyperthermia through CXCL12. Int J Cancer. 2011;128(3):715–725.
- Hesami S, Mohammadi M, Rezaee MA, et al. The effects of hyperthermia on the immunomodulatory properties of human umbilical cord vein mesenchymal stem cells (MSCs). Int J Hyperthermia. 2017;33(7):1–712.
- Oldenborg S, Rasch CRN, van Os R, et al. Reirradiation + hyperthermia for recurrent breast cancer en cuirasse [Rebestrahlung + Hyperthermie bei Brustkrebs in Form von Cancer en cuirasse]. Strahlenther Onkol. 2018;194(3):206–214.
- Sasaki M, Abe R, Fujita Y, et al. Mesenchymal stem cells are recruited into wounded skin and contribute to wound repair by transdifferentiation into multiple skin cell type. J Immunol. 2008;180(4):2581–2587.
- Landry Y, Le O, Mace KA, et al. Secretion of SDF-1alpha by bone marrow-derived stromal cells enhances skin wound healing of C57BL/6 mice exposed to ionizing radiation. J Cell Mol Med. 2010;14(6b):1594–1604.
- Rühle A, Perez RL, Glowa C, et al. Cisplatin radiosensitizes radioresistant human mesenchymal stem cells. Oncotarget. 2017;8(50):87809–87820.
- Notter M, Piazena H, Vaupel P. Hypofractionated re-irradiation of large-sized recurrent breast cancer with thermography-controlled, contact-free water-filtered infra-red-A hyperthermia: a retrospective study of 73 patients. Int J Hyperthermia. 2017;33(2):227–236.
- Dominici M, Le Blanc K, Mueller I, et al. Minimal criteria for defining multipotent mesenchymal stromal cells. The International Society for Cellular Therapy position statement. Cytotherapy. 2006;8(4):315–317.
- Lopez Perez R, Munz F, Kroschke J, et al. Cell cycle-specific measurement of γH2AX and apoptosis after genotoxic stress by flow cytometry. J Vis Exp. 2019;(151). DOI:10.3791/59968.
- Trautinger F, Kokesch C, Herbacek I, et al. Overexpression of the small heat shock protein, hsp27, confers resistance to hyperthermia, but not to oxidative stress and UV-induced cell death, in a stably transfected squamous cell carcinoma cell line. J Photochem Photobiol B. 1997;39(1):90–95.
- Xu M, Wright WD, Higashikubo R, et al. Intracellular distribution of hsp70 during long duration moderate hyperthermia. Int J Hyperthermia. 1998;14(2):211–225.
- Shahar T, Rozovski U, Hess KR, et al. Percentage of mesenchymal stem cells in high-grade glioma tumor samples correlates with patient survival. Neuro Oncol. 2017;19(5):660–668.
- Krueger TE, Thorek DLJ, Meeker AK, et al. Tumor-infiltrating mesenchymal stem cells: drivers of the immunosuppressive tumor microenvironment in prostate cancer? Prostate. 2019;79(3):320–330.
- Karnoub AE, Dash AB, Vo AP, et al. Mesenchymal stem cells within tumour stroma promote breast cancer metastasis. Nature. 2007;449(7162):557–563.
- Liotta F, Querci V, Mannelli G, et al. Mesenchymal stem cells are enriched in head neck squamous cell carcinoma, correlates with tumour size and inhibit T-cell proliferation. Br J Cancer. 2015;112(4):745–754.
- Rühle A, Huber PE, Saffrich R, et al. The current understanding of mesenchymal stem cells as potential attenuators of chemotherapy‐induced toxicity. Int J Cancer. 2018;143(11):2628–2639.
- Rühle A, Perez RL, Zou B, et al. The therapeutic potential of mesenchymal stromal cells in the treatment of chemotherapy-induced tissue damage. Stem Cell Rev and Rep. 2019;15(3):356–373.
- Oei A, Vriend L, Krawczyk P, et al. Targeting therapy-resistant cancer stem cells by hyperthermia. Int J Hyperthermia. 2017;33(4):419–427.
- Deskins DL, Bastakoty D, Saraswati S, et al. Human mesenchymal stromal cells: identifying assays to predict potency for therapeutic selection. Stem Cells Transl Med. 2013;2(2):151–158.
- Nakajima K, Yanagawa T, Watanabe H, et al. Hyperthermia reduces migration of osteosarcoma by suppression of autocrine motility factor. Oncol Rep. 2012;28(6):1953–1958.
- Jin H, Zhao Y, Zhang S, et al. Hyperthermia inhibits the motility of gemcitabine-resistant pancreatic cancer PANC-1 cells through the inhibition of epithelial-mesenchymal transition. Mol Med Rep. 2018;17(5):7274–7280.
- Lee TH, Bu J, Kim BH, et al. Sub-lethal hyperthermia promotes epithelial-to-mesenchymal-like transition of breast cancer cells: implication of the synergy between hyperthermia and chemotherapy. RSC Adv. 2019;9(1):52–57.
- Li L, Jiang J. Regulatory factors of mesenchymal stem cell migration into injured tissues and their signal transduction mechanisms. Front Med. 2011;5(1):33–39.
- Munz F, Lopez Perez R, Trinh T, et al. Human mesenchymal stem cells lose their functional properties after paclitaxel treatment. Sci Rep. 2018;8(1):312.
- Nicolay NH, Liang Y, Lopez Perez R, et al. Mesenchymal stem cells are resistant to carbon ion radiotherapy. Oncotarget. 2015;6(4):2076–2087.
- Rühle A, Xia O, Perez RL, et al. The radiation resistance of human multipotent mesenchymal stromal cells is independent of their tissue of origin. Int J Radiat Oncol Biol Phys. 2018;100(5):1259–1269.
- Lopez Perez R, Brauer J, Rühle A, et al. Human mesenchymal stem cells are resistant to UV-B irradiation. Sci Rep. 2019;9(1):20000.
- Furusawa Y, Iizumi T, Fujiwara Y, et al. Inhibition of checkpoint kinase 1 abrogates G2/M checkpoint activation and promotes apoptosis under heat stress. Apoptosis. 2012;17(1):102–112.
- Roti Roti JL. Cellular responses to hyperthermia (40–46 °C): cell killing and molecular events. Int J Hyperthermia. 2008;24(1):3–15.
- Sinclair W, Morton RA. Variations in X-ray response during the division cycle of partially synchronized Chinese hamster cells in culture. Nature. 1963;199(4899):1158–1160.
- Lopez Perez R, Münz F, Vidoni D, et al. Mesenchymal stem cells preserve their stem cell traits after exposure to antimetabolite chemotherapy. Stem Cell Res. 2019;40:101536.
- Nicolay NH, Lopez Perez R, Rühle A, et al. Mesenchymal stem cells maintain their defining stem cell characteristics after treatment with cisplatin. Sci Rep. 2016;6(1):2003.
- Nicolay NH, Rühle A, Perez RL, et al. Mesenchymal stem cells exhibit resistance to topoisomerase inhibition. Cancer Lett. 2016;374(1):75–84.
- Nicolay NH, Lopez Perez R, Saffrich R, et al. Radio-resistant mesenchymal stem cells: mechanisms of resistance and potential implications for the clinic. Oncotarget. 2015;6(23):19366–19380.
- Harmon BV, Takano YS, Winterford CM, et al. The role of apoptosis in the response of cells and tumours to mild hyperthermia. Int J Radiat Biol. 1991;59(2):489–501.
- Ahmed K, Tabuchi Y, Kondo T. Hyperthermia: an effective strategy to induce apoptosis in cancer cells. Apoptosis. 2015;20(11):1411–1419.
- Sakaguchi Y, Stephens LC, Makino M, et al. Apoptosis in tumors and normal tissues induced by whole body hyperthermia in rats. Cancer Res. 1995;55(22):5459–5464.
- Alekseenko LL, Zemelko VI, Domnina AP, et al. Sublethal heat shock induces premature senescence rather than apoptosis in human mesenchymal stem cells. Cell Stress Chaperones. 2014;19(3):355–366.
- Chen J, Shi ZD, Ji X, et al. Enhanced osteogenesis of human mesenchymal stem cells by periodic heat shock in self-assembling peptide hydrogel. Tissue Eng Part A. 2013;19(5–6):716–728.
- Li C, Sunderic K, Nicoll SB, et al. Downregulation of heat shock protein 70 impairs osteogenic and chondrogenic differentiation in human mesenchymal stem cells. Sci Rep. 2018;8(1):553–553.
- Chen E, Xue D, Zhang W, et al. Extracellular heat shock protein 70 promotes osteogenesis of human mesenchymal stem cells through activation of the ERK signaling pathway. FEBS Lett. 2015;589(24PartB):4088–4096.
- Chen J, Li C, Wang S. Periodic heat shock accelerated the chondrogenic differentiation of human mesenchymal stem cells in pellet culture. PLoS One. 2014;9(3):e91561.
- Seegenschmiedt MH, Klautke G, Walther E, et al. Water-filtered infrared-A-hyperthermia combined with radiotherapy in advanced and recurrent tumors. Initial results of a multicenter phase I-II study. Strahlenther Onkol. 1996;172(9):475–484.
- Dokladny K, Myers OB, Moseley PL. Heat shock response and autophagy—cooperation and control. Autophagy. 2015;11(2):200–213.
- Ravagnan L, Gurbuxani S, Susin SA, et al. Heat-shock protein 70 antagonizes apoptosis-inducing factor. Nat Cell Biol. 2001;3(9):839–843.
- Moloney TC, Hoban DB, Barry FP, et al. Kinetics of thermally induced heat shock protein 27 and 70 expression by bone marrow-derived mesenchymal stem cells. Protein Sci. 2012;21(6):904–909.
- Wang Q, Li X, Wang Q, et al. Heat shock pretreatment improves mesenchymal stem cell viability by heat shock proteins and autophagy to prevent cisplatin-induced granulosa cell apoptosis. Stem Cell Res Ther. 2019;10(1):348.
- Mahmoudi K, Bouras A, Bozec D, et al. Magnetic hyperthermia therapy for the treatment of glioblastoma: a review of the therapy’s history, efficacy and application in humans. Int J Hyperthermia. 2018;34(8):1316–1328.
- Verduijn GM, de Wee EM, Rijnen Z, et al. Deep hyperthermia with the HYPERcollar system combined with irradiation for advanced head and neck carcinoma – a feasibility study. Int J Hyperthermia. 2018;34(7):994–1001.
- Muller AC, Zips D, Heinrich V, et al. Regional hyperthermia and moderately dose-escalated salvage radiotherapy for recurrent prostate cancer. Protocol of a phase II trial. Radiat Oncol. 2015;10(1):138.
- Schuler PJ, Westerkamp AM, Kansy BA, et al. Adenosine metabolism of human mesenchymal stromal cells isolated from patients with head and neck squamous cell carcinoma. Immunobiology. 2017;222(1):66–74.
- Jeschke MG, Rehou S, McCann MR, et al. Allogeneic mesenchymal stem cells for treatment of severe burn injury. Stem Cell Res Ther. 2019;10(1):337.
- Liu L, Yu Y, Hou Y, et al. Human umbilical cord mesenchymal stem cells transplantation promotes cutaneous wound healing of severe burned rats. PLoS One. 2014;9(2):e88348.