Abstract
Purpose
To investigate the mechanism through which hyperthermia promotes exosome secretion and drug sensitivity in adriamycin-resistant breast cancer.
Materials and Methods
We first evaluated the effect of hyperthermia on adriamycin-resistant breast cancer viability and used transmission electron microscopy, nanoparticle tracking analysis, and a bicinchoninic acid kit to validate the effect of hyperthermia on exosome secretion. The effective targeting molecules and pathways changed by hyperthermia were explored by RNA microarray and verified in vitro. The adriamycin-resistant MCF-7/ADR cells co-incubated with the exosomes produced by MCF-7/ADR cells after hyperthermia were assessed. The uptake of exosomes by MCF-7/ADR cells after hyperthermia treatment was evaluated by confocal microscopy. Finally, the mechanism through which hyperthermia promotes exosome secretion by hyperthermia was determined.
Results
Hyperthermia significantly suppressed the growth of adriamycin-resistant breast cancer cells and increased drug sensitivity by upregulating FOS and CREB5, genes related to longer overall survival in breast cancer patients. Moreover, hyperthermia promoted exosome secretion through Rab7b, a small GTPase that controls endosome transport. The upregulated FOS and CREB5 antioncogenes can be transferred to MCF-7/ADR cells by hyperthermia-treated MCF-7/ADR cell-secreted exosomes.
Conclusions
Our results demonstrated a novel function of hyperthermia in promoting exosome secretion in adriamycin-resistant breast cancer cells and revealed the effects of hyperthermia on tumor cell biology. These hyperthermia-triggered exosomes can carry antitumor genes to the residual tumor and tumor microenvironment, which may be more beneficial to the effects of hyperthermia. These results represent an exploration of the relationship between therapeutic strategies and exosome biology.
1. Introduction
Breast cancer is the most common cancer in women. It accounts for 30% of all new cancer cases and is the second leading cause of cancer-related deaths in women worldwide [Citation1]. Traditional treatments for breast cancer, such as surgery, chemotherapy, radiation therapy, and hormone therapy, have made great progress depending on cancer subtypes and tumor stage [Citation2]. Adriamycin, a representative anthracycline, is the first-line chemotherapeutic drug for breast cancer. Although adriamycin is highly effective and broad-spectrum, treatment failure caused by adriamycin resistance remains one of the most challenging problems [Citation3]. Therefore, exploring pivotal resistance-driving genes and their underlying mechanisms may help improve the prognosis of patients with adriamycin-resistant breast cancer.
Recently, more efforts have focused on hyperthermia, a nontraditional treatment. Clinical trials have shown that hyperthermia combined with radiotherapy or chemotherapy can be used to treat many types of cancer, especially recurrent breast cancer, and improve the therapeutic effect of conventional therapy without increasing toxicity [Citation4–7]. Depending on the temperature range, hyperthermia can be divided into sub high-temperature hyperthermia (39.5 °C–41.5 °C), conventional hyperthermia (41.5 °C–45 °C), and high-temperature hyperthermia (>65 °C) [Citation8]. Due to the irregular structure, twisted blood vessels, high blood flow resistance, and lack of elastic basement membrane in tumor tissues, they are more sensitive to hyperthermia than normal tissues [Citation9,Citation10]. Hyperthermia treatment for 30 min to an hour can irreversibly damage tumor cells [Citation11]. This occurs through several reported mechanisms, such as inducing cell cycle arrest, enhancing tumor oxidative stress levels [Citation12,Citation13], changing the state of cell mitochondria [Citation14], inducing DNA damage [Citation15], and regulating the immune system [Citation16]. Hyperthermia can also cooperate with anti-PD-L1 and trigger immunotherapy by promoting CD8+ cytotoxic T lymphocytes [Citation17,Citation18]. As radio- and chemo-sensitizers, however, hyperthermia shows different dose-effect relationships and optimal scheduling, which require more detailed data [Citation19]. Moreover, the effects of hyperthermia on drug resistance in breast cancer and the exact mechanisms leading to the clinical efficacy of hyperthermia are still uncertain.
Exosomes, ranging from 50 to 150 nm, are extracellular vesicles (EVs) that serve as messengers to support tumor growth and crosstalk [Citation20]. Our previous study showed that exosomes can carry drug resistance genes as well as P-glycoprotein (P-gp) from drug-resistant to drug-sensitive breast cancer cells [Citation21]. In this study, we investigated the influence of hyperthermia on exosome secretion and its subsequent effects on cell function. A study found that exosome secretion increased in a temperature-dependent manner and was controlled by the low-density lipoprotein receptor [Citation22]. However, the potential roles of hyperthermia in regulating exosome secretion have yet to be elucidated. In this study, we investigated the potential of hyperthermia in adriamycin-resistant breast cancer and explored the mechanism through which hyperthermia promotes exosome secretion. We also investigated gene expression profiles in drug-resistant breast cancer cell lines after hyperthermia to elucidate cellular responses and identified molecular targets to control exosome secretion.
2. Materials and methods
2.1. Cell lines and culture
The drug-resistant cell line, adriamycin-resistant human breast cancer (MCF-7/ADR), was purchased from Gefan Biotechnology. Co. Ltd. (Shanghai, China). The cells were continuously exposed to 10 µM adriamycin for an additional 6 months. The resistance of MCF-7/ADR to adriamycin was verified when the cells were cultured in adriamycin-free medium for 2 weeks, with an IC50 (inhibitory concentration to produce 50% cell death) value of 46.79 µg/mL. The MCF-7/ADR cells were cultured in drug-free medium for 2 weeks before subsequent experiments to avoid the influence of the drugs. All cells were grown in RPMI-1640 medium (Key GEN Bio TECH, Jiangsu, China) with 10% fetal bovine serum (FBS) at 37 °C in a 5% CO2 humidified atmosphere.
2.2. Hyperthermia treatment
As shown in , when cells were adherent in a T-flask and reached the logarithmic growth stage, the hyperthermia group was incubated at 45 °C for 30 min, and the control group was grown at 37 °C, standard culture conditions, in a 5% CO2 incubator. Prior to hyperthermia treatment, the culture medium was replaced with a medium preheated to 45 °C, while that of the control group was replaced with a normal temperature medium. After the hyperthermia group was pretreated with heat, they were placed in a 37 °C incubator for 6 h before further examination.
Figure 1. Effect of hyperthermia on the proliferation of MCF-7/ADR breast cancer cells. (A) The protocol of hyperthermia treatment. (B) Cell proliferation after hyperthermia treatment via CCK8 assay. (C) Cell proliferation after hyperthermia treatment via Edu assay. (D) Cell cycle of MCF-7/ADR cell treated with hyperthermia by flow cytometry. (E) Cell apoptosis of MCF-7/ADR cell treated with hyperthermia. Error bars represent the mean ± SD of at least three independent experiments, *p < 0.05, **p < 0.01, ***p < 0.001.
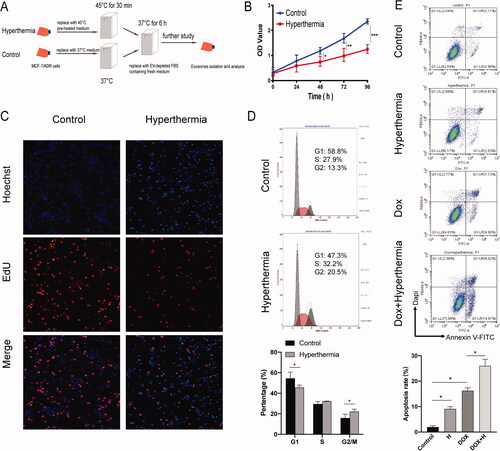
2.3. Mrna library preparation and microarray
Cells were collected 6 h after hyperthermia treatment. Total RNA was extracted using a Total RNA Kit (Tian Gen, DP419). The concentration and quality of the RNA were measured using the UV absorbance at 260 and 280 nm (260/280 nm) on a One Drop OD 1000 Spectrophotometer (One drop, Shanghai, China).
Approximately 4 µg of RNA per sample (three individuals per sample) were used to construct the complementary (cDNA) library. Following manufacturer’s recommendations, the procedures were as follows: mRNA was purified from total RNA using oligo(dT)-attached magnetic beads. Microarray libraries were generated using the NEBNext® UltraTM Directional RNA Library Prep Kit for Illumina® (NEB, Ipswich, UK). Then, libraries were sequenced on an Illumina Hiseq 4000 platform (Illumina, San Diego, CA, USA), and 150 base pairs paired-end reads were generated.
2.4. Microarray analysis
The raw data were treated using the quantile normalization method in the Linear Models for Microarray Data package using R. Functional enrichment analysis of the differentially expressed genes (DEGs) was performed. Pathway analysis to identify gene networks and biological functions was performed using the Database for Annotation, Visualization, and Integrated Discovery (DAVID; http://david.ncifcrf.gov) (version 6.7) [Citation23]. A protein-protein interaction network (PPI) was constructed, and module analysis was performed using STRING (http://string-db.org) (version 10.0) and Cytoscape [Citation24,Citation25]. The effect of the hub genes on overall survival (OS) in patients with breast cancer was analyzed using the Kaplan–Meier plotter online software (http://kmplot.com/analysis/).
2.5. Real-time quantitative PCR (RT-qPCR) for cells and exosomes
From the mRNA microarray, we chose 10 up- or down-regulated genes between the hyperthermia and control groups. We used RT-qPCR to verify the microarray analysis results. To explore the targeted genes regulated in exosomes, we verified the changes in the Rab protein family after hyperthermia, including Rab7b, Rab21, Rab27a, Rab27b, Rab30, and Rab35. The primers are described in Supplementary Table 1. Total RNA was extracted from cells and exosomes using a total RNA Kit (Tian Gen, DP419). cDNA was synthesized using HiScript II Q RT SuperMix (Vazyme, Nanjing, China). Gene expression was examined by RT-qPCR using the ChamQ SYBR qPCR Master Mix (high ROX premixed) (Vazyme, Nanjing, China) on a StepOnePlus Real-Time PCR System (Thermo Fisher Scientific, Waltham, MA, USA). The PCR conditions were as follows: 95 °C for 3 min, followed by 40 cycles at 95 °C for 10 s and 60 °C for 30 s. The melting curve stage to identify the specificity of each PCR reaction included 95 °C for 15 s, 60 °C for 60 s, and 95 °C for 15 s. GAPDH was used as an internal control to normalize mRNA. The relative expression values were calculated using the CT (2–ΔΔCt) method (Livak and Schimittgen 2001). The experiments were performed at least thrice, and the resulting data were statistically analyzed.
2.6. Western blot for cells and exosomes
Total cellular protein and isolated exosome proteins were extracted using cell lysis buffer (Solarbio, Beijing, China) containing phenylmethanesulfonyl fluoride (Beyotime, Shanghai, China) and protease inhibitor cocktail, according to the manufacturer's protocol. The protein concentration was determined using a bicinchoninic acid (BCA) protein assay kit (Beyotime, Shanghai, China), with bovine serum albumin (BSA, Beyotime, Shanghai, China) as the protein standard. All proteins were denatured at 99 °C for 10 min.
Equal amounts of protein were loaded into each lane, separated by SDS-PAGE, and then transferred onto a 0.22-μm PVDF membrane (Millipore, USA). The membrane was incubated for 1 h in a blocking solution. The membrane was then probed with primary antibodies at 4 °C overnight and with species-specific secondary antibodies at room temperature for 1 h. The primary antibodies, including FOS antibody (1:1000, Proteintech, 66590-1-Ig), NF-κB antibody (1:1000, Proteintech, 14220-1-AP), MAPK8 antibody (1:1000, Proteintech, 66210-1-Ig), CREB5 antibody (1:1000, Proteintech, 14196-1-AP), GAPDH antibody (1:5000, Proteintech, 60004-1-Ig), CD9 antibody (1:1000, Abcam, ab223052), CD63 antibody (1:1000, Abcam, ab68418), TSG101 antibody (1:1000, Abcam, ab125011), were confirmed to be suitable for immunoblotting. The secondary antibodies were horseradish peroxidase (HRP)-conjugated sheep anti-mouse IgG and HRP-conjugated donkey anti-rabbit IgG. Finally, bound proteins were detected using the BeyoECL Plus kit (Beyotime, Shanghai, China) and analyzed using the Quantity One system (Bio-Rad).
2.7. Cell viability detection
Cell viability was measured using a Cell Counting Kit-8 (CCK-8, MCE, USA) according to the manufacturer’s instructions. First, 5000 cells were seeded per well into 96-well plates for each condition. Then, the absorption value at 450 nm was determined by repeated measurement of cell viability at 0, 24, 48, and 72 h after seeding using a Multiskan FC spectrophotometer (Thermo Fisher Scientific, USA).
The EdU assay can directly measure changes in DNA to detect cell proliferation. First, 1000 cells were seeded per well into 96-well plates. After entering the logarithmic growth stage, the cells were assessed using the EdU Kit (Ribo, Suzhou, China) according to the manufacturer’s instructions. Finally, fluorescence intensity was observed using a microscope.
2.8. Cell cycle and apoptosis assays
A FACScan flow cytometer (FACSVerse, BD Biosciences, NJ, USA) was used for fluorescence-activated cell sorting (FACS) and analysis. Cell cycle assays were performed using propidium iodide staining (Vazyme, Nanjing, China) according to the manufacturer’s instructions. Apoptosis assays were performed using Annexin V-FITC and DAPI solution as described in [Citation26].
2.9. Cell transfection
MCF-7/ADR cells were transfected with predesigned small interfering RNAs (siRNAs) or a negative control (NC) using Lipofectamine RNAiMax reagent (Thermo Fisher Scientific). SiRNAs for FOS, CREB5, MAPK8, NFKB1, and Rab7b were purchased from Ribo Life Science (Suzhou, China). Also, MCF-7/ADR cells were transfected with overexpression plasmid or NC using Lipofectamine 3000 reagent (Thermo Fisher Scientific). The plasmid pcDNA3.1-Rab7b was purchased from PPL (Nanjing, China). Transfection steps were performed according to the manufacturer’s protocols.
2.10. Exosome isolation
Exosomes were extracted by sequential ultracentrifugation according to the manufacturer’s protocols. Briefly, MCF-7/ADR cells were cultured in RPMI-1640 medium containing 10% EV‑depleted FBS. The conditioned medium was collected and centrifuged at 300 × g for 10 min to remove the cells. The supernatant was then centrifuged at 2000 × g for 15 min to remove dead cells and at 12,000 × g for 30 min to remove cell debris. The resulting supernatant was filtered through a 0.22-μm filter, and the exosomes were pelleted by ultracentrifugation at 100,000 × g (Beckman Type 90 Ti) for 2 h. The exosome pellet was washed in PBS and collected by ultracentrifugation at 100,000 × g (Beckman Type 90 Ti) for 2 h. Finally, the supernatant was removed, and the exosome pellets were resuspended in 100 μl PBS.
2.11. Transmission electron microscopy (TEM)
Photomicrographs of MCF-7/ADR cells and exosomes derived from these were acquired using a Tecnai G2 Spirit Bio TWIN TEM (FEI, USA). MCF-7/ADR cells were fixed with 2.5% glutaraldehyde at 4 °C overnight. Then, all subjects were washed twice with PBS and precipitated in 1% OsO4 for 1 h at room temperature. The precipitates were dehydrated in ethyl alcohol and embedded in epoxy resin. The isolated exosomes derived from MCF-7/ADR cells were added to a carbon-coated copper network for adsorption for 120 s, washed with distilled water, and then negatively stained with uranium acetate for 120 s.
2.12. Nanoparticle tracking analysis (NTA)
To analyze the size, distribution, and concentration of exosomes, ZetaView® PMX120 Nanoparticle Tracking Analyzer was used. Exosome samples were diluted to appropriate multiples by PBS and pumped into the measurement. The camera of ZetaView recorded individual particle scattering to determine the mean velocity and diffusivity in 60-s videos. The ZetaView® software was used for instrument control and data analysis.
2.13. Exosome uptake assessment
To assess the uptake ability of exosomes by MCF-7/ADR cells after hyperthermia treatment, exosomes derived from MCF-7/ADR cells were first stained with PKH26 red fluorescent dye (Sigma-Aldrich, United Kingdom). Then, the exosomes were washed with 10 ml of PBS and pelleted by ultracentrifugation at 100,000 × g (Beckman Type 90 Ti) for 2 h. Meanwhile, the hyperthermia group was incubated at 45 °C for 30 min then placed in a 37 °C incubator for 6 h. Then, the hyperthermia and control groups were incubated with dye-stained exosomes on confocal plates for 24 h, followed by confocal microscopy to determine exosome uptake.
2.14. Statistical analysis
All experiments were repeated at least thrice independently. All measurement data are expressed as mean ± standard deviation. One-way ANOVA was used for comparison between multiple groups, and the t-test of independent samples was used to compare the two groups. Statistical significance was set at p < .05.
3. Results
3.1. Hyperthermia can suppress the proliferation and growth of MCF-7/ADR breast cancer cells
The temperature and exposure time of the hyperthermia treatment determined cell inhibition. Based on the definition of the thermal dose (D) derived from the exposure time (t) and given temperature (T), this formula, which is D = tRT with T = 43 and R = 2 for temperatures ≥43 °C, and R = 4 for temperatures <43 °C, was used to normalize the thermal dose [Citation27]. Hence, we selected 45 °C for 30 min to conduct the hyperthermia treatment to achieve a good hyperthermia effect and minimize the influence of operating time. The protocol of hyperthermia treatment is shown in .
To determine the effects of hyperthermia treatment in drug-resistant breast cancer cells, we first compared cell proliferation, cell cycle, and cell apoptosis of MCF-7/ADR cells in the hyperthermia and control groups. First, the CCK-8 assay results showed that hyperthermia can greatly inhibit the proliferation of drug-resistant breast cancer cells (), which was consistent with the results of the EdU assay (). Moreover, we focused on the arrest period of the cell cycle and the flow cytometry analysis showed that the number of G2/M phase cells in the hyperthermia group was significantly higher than that in the control group; G1 phase cells were significantly fewer than those in the control group (). The apoptosis rate of cells was also significantly higher after hyperthermia treatment using flow cytometry analysis (). Although hyperthermia could not directly promote the death of most cells, it significantly inhibited the growth of drug-resistant cells, which supports the possibility for treatment in combination with other therapies.
Furthermore, we found that hyperthermia increased drug sensitivity and reduced the IC50 of adriamycin-treated MCF-7/ADR cells (). At different doses of adriamycin, hyperthermia can promote the cytotoxic effects of adriamycin on drug-resistant cells. Also, hyperthermia can reverse drug resistance, with the IC50 values of MCF7, MCF-7/ADR, and MCF-7/ADR hyperthermia groups being 2.713 μg/mL, 46.79 μg/mL, and 6.549 μg/mL, respectively. These results suggest that hyperthermia can not only significantly control cell proliferation and apoptosis, but also promote drug sensitivity in breast cancer.
Figure 2. Hyperthermia increases drug sensitivity of MCF-7/ADR breast cancer cells. (A) Cell viability of MCF-7/ADR cell after combination of hyperthermia and adriamycin treatment. (B) Half-inhibition rate of adriamycin in MCF-7/ADR cells after hyperthermia treatment. Error bars represented the mean ± SD of at least three independent experiments, *p < 0.05, **p < 0.01, ***p < 0.001.
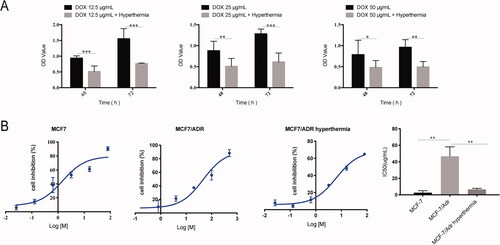
3.2. Transcriptional profiles of MCF-7/ADR cells treated with hyperthermia
To explore the molecular mechanisms underlying this thermal regulation in breast cancer, we analyzed the genome-wide gene expression profiles of hyperthermia-treated MCF-7/ADR cells. Using microarray analysis, we identified 2046 upregulated genes and 1724 downregulated genes with the following criteria: (i) p value <.05, (ii) q value <.05, and (iii) fold change >2 (). The top 20 DEGs after hyperthermia treatment in MCF7/ADR cells are listed in Supplement Tables 2 and 3. Furthermore, to explore the hub genes that play important roles in the molecular mechanisms of hyperthermia treatment, we constructed a PPI network using Cytoscape (). This PPI network included an interaction network and functional nodes which indicated the biological response of MCF7/ADR cells to hyperthermia. From the PPI network, we analyzed 300 nodes (203 upregulated genes and 97 downregulated genes) and found 673 interaction pairs. Furthermore, a total of 10 genes were identified as hub genes with ≥8 degrees, as shown in . From the 10 hub genes, we found that MAPK8, NFKB1, PLCB4, FOS, CREB5, and RAC2 were significantly related to OS. With the higher expression of MAPK8, NFKB1, FOS, CREB5, and RAC2, the prognosis of patients is better. With the lower expression of PLCB4, the prognosis of patients was better (). The upregulation and downregulation of these genes resulted from hyperthermia treatment-induced prognostic changes, which is consistent with how hyperthermia can improve the prognosis of breast cancer.
Figure 3. Analysis of microarray data reveals distinct transcriptional profiles by hyperthermia in MCF-7/ADR cells. (A) Scatterplot of differentially expressed genes and sample clustering analysis for all replicates of hyperthermia and control groups. (B) Heat map representation of the expression profiles of genes significantly changed by hyperthermia in MCF-7/ADR cells. The expression level of each transcript is represented by the color range, red indicates high and blue indicates low. Columns indicate arrays and rows indicate genes. (C) The protein-protein interaction (PPI) network of differentially expressed genes (DEGs). The red circle represents the up-regulated genes and the blue circles down-regulated genes. The area of the circle represents the degree. (D) Overall survival analyses of hub genes were performed using Kaplan–Meier analysis. Raw data was normalized within each row. p value < .05, q value < .05, and Fold change > 2.
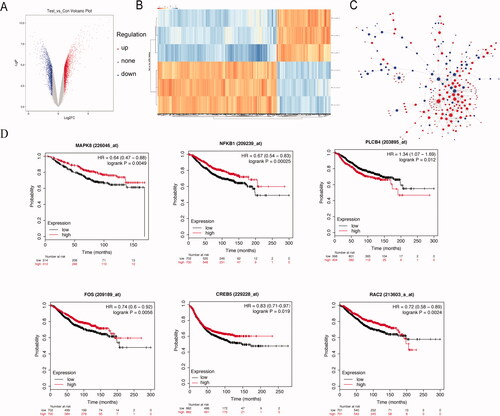
Table 1. List of the top 10 hub genes by hyperthermia treatment.
3.3. Hyperthermia upregulates anti-tumor genes FOS and CREB5 to suppress drug resistance in breast cancer
To explore the potential mechanisms of hyperthermia, the transcriptional levels of 10 selected genes were examined by RT-qPCR and compared with microarray data in independently collected samples (). The results were similar to those obtained with the microarray, confirming the accuracy of the microarray assay. Furthermore, we identified four genes with the most significant differences in expression: FOS, CREB5, MAPK8, and NFKB1. To further verify the protein levels of significantly differentially expressed genes after hyperthermia, western blot was performed (). The total FOS and CREB5 protein levels were substantially increased in the hyperthermia group, which was consistent with the RT-qPCR results. However, there was no significant difference in the protein levels of MAPK8 and NFKB1 between the control and hyperthermia groups. Therefore, we suspected that FOS and CREB5 play important roles in hyperthermia therapy.
Figure 4. The validation experiment of hub genes. (A) The differences in gene expression of 10 hub genes were validated with RT-qPCR. (B) Western blot analysis of FOS, CREB5, MAPK8 and NFKB1 protein level. (C) The efficiency of siRNA to knockdown the expression of FOS and CREB5. (D) Cell proliferation in the si-FOS and si-CREB5 group was faster compared with that in the control group, using the CCK-8 assay. (E). Half-inhibition rate of adriamycin in MCF-7/ADR cells treated with si-FOS and si-CREB5. (F) Different expression of FOS and CERB5 between invasive breast cancer and normal breast tissue by TCGA database. Error bars represented the mean ± SD of at least three independent experiments, *p < .05, **p < .01, ***p < .001.
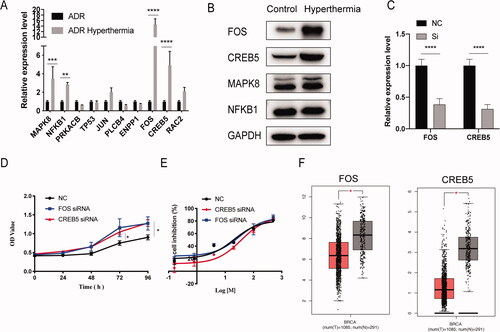
To identify the functions of FOS and CREB5 in hyperthermia MCF-7/ADR cells, we tested whether knocking down these genes can inhibit the effect of hyperthermia. The knockdown efficiency of the siRNA is shown in . From the cell proliferation assay, we found that knocking down the expression of FOS and CREB5 can reverse the inhibitory effect of hyperthermia on the proliferation of drug-resistant cells (). Also, the IC50 of NC, si-FOS, and si-CREB5 group were 20.48 μg/mL, 32.26 μg/mL, and 36.47 μg/mL, respectively (). Thus, knocking down FOS and CREB5 can reverse the effects of hyperthermia in adriamycin-resistant breast cancer. Furthermore, analysis of the TCGA database showed that the expression of FOS and CREB5 in normal breast tissues was significantly higher than that in breast cancer tissues (). Therefore, FOS and CREB5 may be regarded as biomarkers for the effects of hyperthermia and may be selected as potential targets in hyperthermia therapy for drug-resistant breast cancer.
3.4. Hyperthermia promotes exosome secretion and uptake in breast cancer
In 2014, we found that exosomes can effectively deliver drug resistance genes as well as P-gp from drug-resistant to drug-sensitive breast cancer cells [Citation21]. Recently, our group found that sEVs can mediate the transmission of heat shock protein 70 between cells and enhance the resistance of receptor cells to adriamycin, mainly by reprogramming the energy metabolism of receptor cells [Citation28]. This inspired us to study whether these upregulated antioncogenes after hyperthermia treatment can be delivered to drug-resistant cells by exosomes. We used 1640 medium containing 10% exosome-depleted FBS to culture MCF-7/ADR cells for 6 h after hyperthermia treatment. Cell numbers were counted in each group while collecting the cell culture supernatants. Exosomes were extracted by sequential ultracentrifugation and analyzed by TEM, western blot, and NTA (Supplement Figure 1). Due to the dehydration during sample preparation for the TEM, the contraction of exosomes resulted in a smaller measured particle size [Citation29,Citation30].
After hyperthermia treatment, the expression levels of FOS and CREB5 in MCF-7/ADR-secreted exosomes were detected by RT-qPCR. We found that FOS and CREB5 were upregulated in exosomes of the hyperthermia group compared to the control group (). Moreover, to study the gene transfer function of exosomes, we co-incubated MCF-7/ADR cells with the exosomes produced by MCF-7/ADR cells treated with hyperthermia (hyperthermia ADR/exo) for 24 h and then detected the sensitivity of MCF-7/ADR to adriamycin. As shown in , there was greater cytotoxicity and a lower IC50 in MCF-7/ADR cells treated with hyperthermia ADR/exo compared to untreated cells. The IC50 of hyperthermia ADR/exo-treated MCF-7/ADR to adriamycin was 16.95 μg/mL, compared to 28.58 μg/mL for untreated MCF-7/ADR. Therefore, antioncogenes upregulated by hyperthermia treatment can be delivered to drug-resistant cells by exosomes, subsequently reducing drug resistance. As shown in , the expression levels of FOS and CREB5 in MCF-7/ADR cells were upregulated when treated with hyperthermia ADR/exo ().
Figure 5. Hyperthermia promotes the secretion of exosome in MCF-7/ADR cells. (A) The expression level of FOS and CREB5 in MCF-7/ADR exosomes after hyperthermia. (B) Half-inhibition rate of adriamycin in MCF-7/ADR cells incubated with the exosomes which produced by MCF-7/ADR cells after hyperthermia. (C) The expression level of FOS and CREB5 in MCF-7/ADR after incubated with hyperthermia ADR/exo. (D) Particles concentration of exosomes derived from breast cancer cells analyzed by NTA. (E) Total protein amounts of exosomes derived from breast cancer cells analyzed by bicinchoninic acid (BCA) protein kit. (F) Transmission electron micrographs (TEM) of cells before or 6 h after hyperthermia. Intense extracellular vesicle shedding occurred 6 h after hyperthermia. Scale bar =1 μm. (G) Confocal microscope analysis of exosome uptake by MCF-7/ADR cells with or without hyperthermia treatment. Scale bar =20 μm. Error bars represent the mean ± SD of at least three independent experiments, *p < .05, **p < .01, ***p < .001.
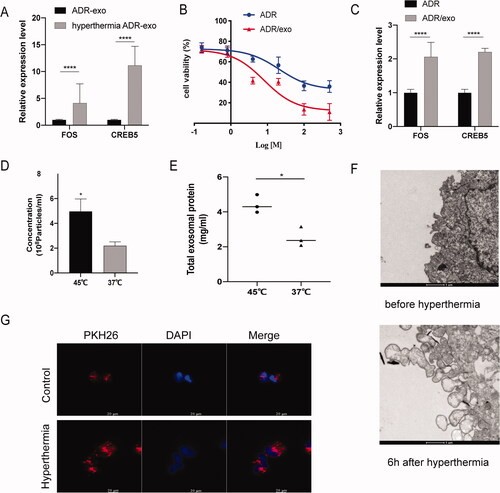
Surprisingly, we also found that hyperthermia promoted exosome release in breast cancer cells. We used NTA to compare the amount and size of exosomes and used a BCA protein kit to compare the total protein of exosomes released after hyperthermia. To normalize the cell numbers, we divided the concentration of exosomes into cell numbers to determine the exosome secretion number of each cell (particles/cell). These results showed that the number of exosomes released was significantly increased after hyperthermia treatment, while the size showed no significant difference (). Exosomes are intraluminal vesicles (ILVs) released from the fusion of multivesicular bodies (MVBs) with the plasma membrane [Citation31]. TEM images demonstrated that hyperthermia led to vesicle formation at the plasma membrane of MCF-7/ADR cells, which subsequently secreted more EVs, including exosomes and microvesicles (). To further characterize the exosome uptake of MCF-7/ADR cells affected by hyperthermia, laser scanning confocal microscopy showed that hyperthermia can promote the uptake of exosomes in MCF-7/ADR cells, which may help deliver target genes (). As shown here, after hyperthermia treatment, exosome secretion by MCF-7/ADR cells was not only abundant, but also rapid.
3.5. Hyperthermia promotes exosome secretion by regulating Rab7b in adriamycin-resistant breast cancer
Consistent with the experimental results, the KEGG pathway analysis based on the above differential genes revealed that the MAPK, PI3K-Akt, and p53 signaling pathways, as well as endocytosis, were important in regulating exosome secretion and physiological functions [Citation32,Citation33] (). The ExoBCD database identified 1696 KEGG pathways in enriched exosomal molecules, of which the MAPK signaling pathway was identified as the most affected canonical pathway [Citation34]. Thus, we found that hyperthermia can regulate exosome secretion; we also suspected that the increased exosomes can deliver more oncogenes to tumors, and cancer cells can take in more exosomes after hyperthermia treatment.
Figure 6. Effects and mechanisms of hyperthermia on EV secretion in breast cancer. (A) The kyoto encyclopedia of genes and genomes (KEGG) pathway enrichment analysis of up-regulated genes. (B) The kyoto encyclopedia of genes and genomes (KEGG) pathway enrichment analysis of down-regulated genes. (C) Effect of hyperthermia on Ras superfamily genes expression. The expression levels were confirmed by RT-qPCR and normalized to the expression of GAPDH. (D) The efficiency examination of three pre-designed siRNAs of Rab7b by RT-qPCR. (E) Particle concentration of exosomes from si-Rab7b cells cultured at 37 °C (mean ± SEM, n = 5) measured by NTA. (F) Total protein of exosomes from si-Rab7b cells cultured at 37 °C (mean ± SEM, n = 5) quantified by BCA kit. (G) Particle concentration of exosomes from si-Rab7b cells preheated at 45 °C (mean ± SEM, n = 5) measured by NTA. (H) Total protein of exosomes from si-Rab7b cells preheated at 45 °C (mean ± SEM, n = 5) quantified by BCA kit. (I) The efficiency examination of pre-designed pcDNA3.1-Rab7b by RT-qPCR. (J) Particle concentration of exosomes from pcDNA3.1-Rab7b cells cultured at 37 °C (mean ± SEM, n = 5) measured by NTA. (K) Total protein of exosomes from pcDNA3.1-Rab7b cells cultured at 37 °C (mean ± SEM, n = 5) quantified by BCA kit. Number of data points per sample was at least three. *p < .05, **p < .01, ***p < .001.
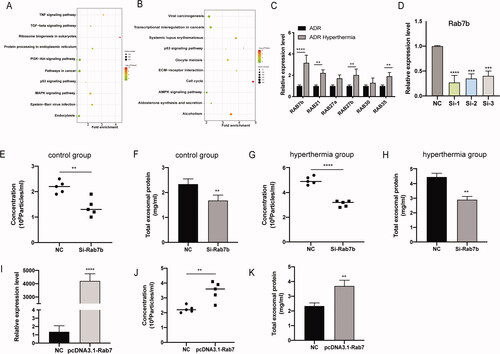
To elucidate the molecular mechanisms underlying the regulation of exosome secretion by hyperthermia, we narrowed down the list of potential genes responsible for hyperthermia response in cancer cells. The Rab protein family is the largest subfamily of the Ras superfamily, which is a small GTP binding protein that regulates the secretion, recycling, and maturation of exosomes in eukaryotes [Citation35]. We identified six Ras superfamily genes responsible for exosome secretion according to their transcriptional profile (). RT-qPCR analysis showed that the expressions of Rab7b, Rab21, Rab35, and Rab27b were significantly upregulated after hyperthermia treatment and correlated with promoting exosome secretion, with Rab7b demonstrating the most marked upregulation ().
Table 2. List of the 6 Ras superfamily genes changed in hyperthermia group.
Therefore, we knocked down Rab7b gene expression to determine its functional effects on EV secretion. MCF-7/ADR cells were transfected with si-Rab7b, including siRNA-1, −2, and −3, and the cells were cultured for another 48 h in fresh medium after transfection. No significant differences were observed in the number of cells. The efficiency examination was performed by RT-qPCR, and siRNA-1 was found to be the most effective (). Therefore, we chose siRNA-1 for further studies. After collecting the cell culture supernatants, we used NTA and BCA kit to identify the amount, size, and total protein of exosomes released by MCF-7/ADR cells. We found that the exosome concentrations were significantly decreased in Rab7b knockdown cells in both the hyperthermia and control groups, indicating that Rab7b is correlated with exosome formation and secretion (). Also, overexpression of Rab7b in MCF-7/ADR cells significantly promoted the release of exosomes (). Furthermore, our results showed that hyperthermia promoted exosome secretion by upregulating Rab7b in adriamycin-resistant breast cancer.
Discussion
Breast cancer seriously threatens women's health, and the incidence of breast cancer increases every year. Although comprehensive treatment centered on surgery has greatly improved the survival rate of patients, the recurrence, metastasis, and drug resistance of breast cancer still leads to poor therapeutic responses and high mortality in some patients [Citation36]. Adriamycin is an antitumor antibiotic that can inhibit RNA and DNA synthesis. It has the strongest inhibitory effect on RNA synthesis and has a wide antitumor spectrum. However, adriamycin resistance is challenging in clinical treatment. The mechanisms behind adriamycin resistance have been extensively studied and are mostly related to P-gp, resistance proteins, anti-apoptosis, autophagy, and other mechanisms [Citation37,Citation38]. It was found that tumor cells can gain drug resistance by increasing the threshold of apoptosis [Citation39]. To reverse adriamycin resistance in breast cancer, we developed a new adjuvant therapy to interrupt specific pathways and investigate these intrinsic molecular mechanisms.
In 1985, hyperthermia was officially certified as the fifth cancer treatment method by the Food and Drug Administration and was recognized as a green therapy. The application of tumor hyperthermia has become increasingly extensive [Citation9]. Seynhaeve found that mild hyperthermia can serve as a trigger signal for drug delivery to promote drug uptake and penetration [Citation40]. Moreover, a previous study by our research group confirmed the effect of hyperthermia in the treatment of advanced breast cancer. For patients with clinical stages III or IV who had residual breast lesions that were difficult to further reduce after comprehensive treatment, such lesions were treatable by magnetic resonance-guided radiofrequency ablation. We demonstrated that the treatment was safe and effective through follow-up [Citation41]. Here, we found that hyperthermia not only increased the apoptosis and drug sensitivity of MCF7/ADR cells to adriamycin, but also promoted exosome secretion and exosome uptake, which are significant in reversing multidrug resistance in tumor cells.
To explore the molecular mechanisms underlying this thermal regulation in breast cancer, we used microarray analysis to analyze the genome-wide gene expression profiles of hyperthermia-treated MCF-7/ADR cells. We selected 10 DEGs as hub genes and validated them by RT-qPCR and western blot. The results showed that FOS and CREB5 played the most important roles in hyperthermia treatment. FOS, as a critical regulator of the invasive phenotype, participates in cell differentiation, proliferation, and transformation. It can also form a heterodimer with Jun, whithen bind to the AP-1 site with high affinity [Citation42,Citation43]. Furthermore, Kurozumi found that FOS was expressed at lower levels in lymphovascular invasion-positive cases, which was associated with the development of metastasis in invasive breast cancer [Citation44]. CREB5, also known as CAMP response element binding protein 5, can promote the expression of IL6 and CSF3 and induce cell apoptosis via the Bad/Bax/caspase 9 signaling pathway [Citation45]. In addition, after incubation with hyperthermia ADR/exo, the drug resistance of MCF-7/ADR was lower than that of MCF-7/ADR in the absence of ADR/exo, which indicated the delivery function of exosomes between cells. Thus, we hypothesized that hyperthermia can reverse the drug resistance of adriamycin-resistant breast cancer cells by overexpression of FOS and CREB5, which can be delivered to the residual tumor and tumor microenvironment, thereby enhancing the effect of hyperthermia.
Exosomes are double-layered vesicles that are important mediators of intracellular communication. Exosomes from different cells can not only carry specific protein molecules, but also contain key molecules to perform their functions. Thus, targeting exosome secretion has emerged as an attractive therapeutic target that can be adjusted by various conditions, such as hypoxia, photodynamic treatment, and hydrochloride hydrate GW4869 [Citation46]. Additionally, exosome release is generally associated with early apoptotic events [Citation47]. The complex processes of exosome secretion are regulated by various molecules, such as RAS-related protein (RAB) GTPases [Citation31]. Here, our results showed that Rab7b, a small GTPase that can control transport from early endosomes to late endocytic compartments—such as late endosomes and lysosomes—contributes to the promotion of exosome release by hyperthermia. With high similarity to Rab7, Rab7b controls endosome transport and promotes fusion with the plasma membrane, being localized both to the trans-Golgi network and to the late endosomes [Citation48,Citation49].
For drug-resistant cancer, exosomes can be used as carriers to transfer drug-resistant molecules between tumor cells. Cesi et al. found that EVs could transport a truncated but functional form of ALK in melanoma drug resistance and were able to activate the MAPK signaling pathway in target cells to transfer drug resistance [Citation50]. However, exosomes can also be used for passive transport of targeted tumor therapy because of their enhanced permeability and retention (EPR) effect. For example, exosomes containing IL-3 ligand or BCR-ABL functional siRNA were successfully used against imatinib resistance in chronic myeloid leukemia [Citation51]. Thus, improving the amounts of exosomes and carrying antitumor molecules are significant for targeted therapy with engineered exosomes.
In this study, our results showed that hyperthermia can not only reverse adriamycin resistance of tumors by upregulating FOS and CREB5 genes, which were related to longer OS in breast cancer patients, but also promote exosomes secretion mediated by Rab7b. These exosomes can also carry antitumor genes to the residual tumor and tumor microenvironment, which may reduce drug resistance and enhance the effect of hyperthermia. Our findings shed new light on drug resistance in the progression of breast cancer, particularly regarding EV secretion. However, more comprehensive research is needed to fully understand the mechanisms behind the generation and secretion of EVs. It is important to pay attention to the amount and content of exosomes and develop new therapies for cancer diagnosis and treatment. Our study investigated the potential mechanisms of hyperthermia by transcriptome analysis and provided a new perspective on exosome biology, which may promote the development of engineering exosome therapy in cancer.
Supplemental Material
Download PDF (173.5 KB)Disclosure statement
No potential conflict of interest was reported by the author(s).
Additional information
Funding
Reference
- Siegel RL, Miller KD, Jemal A. Cancer statistics, 2020. CA A Cancer J Clin. 2020;70(1):7–30.
- Fraguas-Sanchez AI, Martin-Sabroso C, Fernandez-Carballido A, et al. Current status of nanomedicine in the chemotherapy of breast cancer. Cancer Chemother Pharmacol. 2019;84:689–706.
- Liedtke C, Mazouni C, Hess KR, et al. Response to neoadjuvant therapy and long-term survival in patients with triple-negative breast cancer. J Clin Oncol. 2008;26(8):1275–1281.
- Bakker A, van der Zee J, van Tienhoven G, et al. Temperature and thermal dose during radiotherapy and hyperthermia for recurrent breast cancer are related to clinical outcome and thermal toxicity: a systematic review. Int J Hyperthermia. 2019;36(1):1024–1039.
- Datta NR, Puric E, Klingbiel D, et al. Hyperthermia and radiation therapy in locoregional recurrent breast cancers: a systematic review and Meta-analysis. Int J Radiat Oncol Biol Phys. 2016;94(5):1073–1087.
- Dharmaiah S, Zeng J, Rao VS, et al. Clinical and dosimetric evaluation of recurrent breast cancer patients treated with hyperthermia and radiation. Int J Hyperthermia. 2019;36(1):986–992.
- Oldenborg S, van Os R, Oei B, et al. Impact of technique and schedule of reirradiation plus hyperthermia on outcome after surgery for patients with recurrent breast cancer. Cancers. 2019;11(6):782.
- Wust P, Hildebrandt B, Sreenivasa G, et al. Hyperthermia in combined treatment of cancer. Lancet Oncol. 2002;3(8):487–497.
- van der Zee J. Heating the patient: a promising approach? Ann Oncol. 2002;13(8):1173–1184.
- Reinhold HS, Endrich B. Tumour microcirculation as a target for hyperthermia. Int J Hyperthermia. 1986;2(2):111–137.
- Habash RW, Bansal R, Krewski D, et al. Thermal therapy, part 2: hyperthermia techniques. Crit Rev Biomed Eng. 2006;34(6):491–542.
- Mundhara N, Majumder A, Panda D. Hyperthermia induced disruption of mechanical balance leads to g1 arrest and senescence in cells. Biochem J. 2021;478(1):179–196.
- Sola-Leyva A, Jabalera Y, Chico-Lozano MA, et al. Reactive oxygen species (ros) production in hepg2 cancer cell line through the application of localized alternating magnetic field. J Mater Chem B. 2020;8(34):7667–7676.
- Yuen WF, Fung KP, Lee CY, et al. Hyperthermia and tumour necrosis factor-alpha induced apoptosis via mitochondrial damage. Life Sci. 2000;67(6):725–732.
- Kampinga HH, Dynlacht JR, Dikomey E. Mechanism of radiosensitization by hyperthermia (> or = 43 degrees c) as derived from studies with DNA repair defective mutant cell lines. Int J Hyperthermia. 2004;20(2):131–139.
- Zhang HG, Mehta K, Cohen P, et al. Hyperthermia on immune regulation: a temperature’s story. Cancer Lett. 2008;271(2):191–204.
- Liu X, Zheng J, Sun W, et al. Ferrimagnetic vortex nanoring-mediated mild magnetic hyperthermia imparts potent immunological effect for treating cancer metastasis. ACS Nano. 2019;13(8):8811–8825.
- Wang R, He Z, Cai P, et al. Surface-functionalized modified copper sulfide nanoparticles enhance checkpoint blockade tumor immunotherapy by photothermal therapy and antigen capturing. ACS Appl Mater Interfaces. 2019;11(15):13964–13972.
- Oei AL, Kok HP, Oei SB, et al. Molecular and biological rationale of hyperthermia as radio- and chemosensitizer. Adv Drug Deliv Rev. 2020;163–164:84–97.
- Aigner A. Applications of rna interference: current state and prospects for sirna-based strategies in vivo. Appl Microbiol Biotechnol. 2007;76(1):9–21.
- Lv MM, Zhu XY, Chen WX, et al. Exosomes mediate drug resistance transfer in mcf-7 breast cancer cells and a probable mechanism is delivery of p-glycoprotein. Tumour Biol. 2014;35(11):10773–10779.
- Otsuka K, Yamamoto Y, Ochiya T. Uncovering temperature-dependent extracellular vesicle secretion in breast cancer. J Extracell Vesicles. 2020;10(2):e12049.
- Huang da W, Sherman BT, Lempicki RA. Systematic and integrative analysis of large gene lists using david bioinformatics resources. Nat Protoc. 2009;4(1):44–57.
- Szklarczyk D, Morris JH, Cook H, et al. The string database in 2017: quality-controlled protein-protein association networks, made broadly accessible. Nucleic Acids Res. 2017;45(D1):D362–D368.
- Shannon P, Markiel A, Ozier O, et al. Cytoscape: a software environment for integrated models of biomolecular interaction networks. Genome Res. 2003;13(11):2498–2504.
- Lee SM, Ahn RW, Chen F, et al. Biological evaluation of ph-responsive polymer-caged nanobins for breast cancer therapy. ACS Nano. 2010;4(9):4971–4978.
- Hildebrandt B, Wust P, Ahlers O, et al. The cellular and molecular basis of hyperthermia. Crit Rev Oncol Hematol. 2002;43(1):33–56.
- Hu W, Xu Z, Zhu S, et al. Small extracellular vesicle-mediated hsp70 intercellular delivery enhances breast cancer adriamycin resistance. Free Radic Biol Med. 2021;164:85–95.
- Bachurski D, Schuldner M, Nguyen PH, et al. Extracellular vesicle measurements with nanoparticle tracking analysis - an accuracy and repeatability comparison between nanosight ns300 and zetaview. J Extracell Vesicles. 2019;8(1):1596016.
- Szatanek R, Baj-Krzyworzeka M, Zimoch J, et al. The methods of choice for extracellular vesicles (evs) characterization. Int J Mol Sci. 2017;18(6):1153.
- van Niel G, D’Angelo G, Raposo G. Shedding light on the cell biology of extracellular vesicles. Nat Rev Mol Cell Biol. 2018;19(4):213–228.
- Fu C, Zhang Q, Wang A, et al. Ewi-2 controls nucleocytoplasmic shuttling of egfr signaling molecules and mirna sorting in exosomes to inhibit prostate cancer cell metastasis. Mol Oncol. 2021;15(5):1543–1565.
- Wu S, Luo M, To KKW, et al. Intercellular transfer of exosomal wild type egfr triggers osimertinib resistance in non-small cell lung cancer. Mol Cancer. 2021;20(1):17.
- Wang X, Chai Z, Pan G, et al. Exobcd: a comprehensive database for exosomal biomarker discovery in breast cancer. Brief Bioinform. 2020;22(3):bbaa088.
- Sinha D, Roy S, Saha P, et al. Trends in research on exosomes in cancer progression and anticancer therapy. Cancers. 2021;13(2):326.
- Li Q, Ma Z, Liu Y, et al. Low doses of paclitaxel enhance liver metastasis of breast cancer cells in the mouse model. Febs J. 2016;283(15):2836–2852.
- Sun WL, Lan D, Gan TQ, et al. Autophagy facilitates multidrug resistance development through inhibition of apoptosis in breast cancer cells. Neoplasma. 2015;62(2):199–208.
- Hu Y, Li C, Li H, et al. Resveratrol-mediated reversal of tumor multi-drug resistance. Curr Drug Metab. 2014;15(7):703–710.
- Nowak R, Tarasiuk J. The inhibition of apoptosis in cancer cells resistant to anticancer drugs. Postepy Biochem. 2004;50(4):330–343.
- Seynhaeve ALB, Amin M, Haemmerich D, et al. Hyperthermia and smart drug delivery systems for solid tumor therapy. Adv Drug Deliv Rev. 2020;163–164:125–144.
- Li J, Wang DD, Zhao YN, et al. Clinical assessment of magnetic resonance imaging-guided radiofrequency ablation for breast cancer. Mol Clin Oncol. 2019;11(4):411–415.
- Ozanne BW, Spence HJ, McGarry LC, et al. Transcription factors control invasion: Ap-1 the first among equals. Oncogene. 2007;26(1):1–10.
- Sundqvist A, Voytyuk O, Hamdi M, et al. Jnk-dependent cjun phosphorylation mitigates tgfbeta- and egf-induced pre-malignant breast cancer cell invasion by suppressing ap-1-mediated transcriptional responses. Cells. 2019;8(12):1481.
- Kurozumi S, Joseph C, Sonbul S, et al. A key genomic subtype associated with lymphovascular invasion in invasive breast cancer. Br J Cancer. 2019;120(12):1129–1136.
- Zhou Y, Fu X, Guan Y, et al. 1,3-dicaffeoylquinic acid targeting 14-3-3 tau suppresses human breast cancer cell proliferation and metastasis through il6/jak2/pi3k pathway. Biochem Pharmacol. 2020;172:113752.
- McAndrews KM, Kalluri R. Mechanisms associated with biogenesis of exosomes in cancer. Mol Cancer. 2019;18(1):52.
- Hugel B, Martinez MC, Kunzelmann C, et al. Membrane microparticles: two sides of the coin. Physiology. 2005; 20:22–27.
- Guerra F, Bucci C. Multiple roles of the small gtpase rab7. Cells. 2016;5(3):34.
- Progida C, Cogli L, Piro F, et al. Rab7b controls trafficking from endosomes to the tgn. J Cell Sci. 2010;123(Pt 9):1480–1491.
- Cesi G, Philippidou D, Kozar I, et al. A new alk isoform transported by extracellular vesicles confers drug resistance to melanoma cells. Mol Cancer. 2018;17(1):145.
- Bellavia D, Raimondo S, Calabrese G, et al. Interleukin 3- receptor targeted exosomes inhibit in vitro and in vivo chronic myelogenous leukemia cell growth. Theranostics. 2017;7(5):1333–1345.