Abstract
We investigated the effect of particle pre-existing charges on unipolar charging. Particles carrying a defined number and polarity of pre-existing charges were used to study the unipolar charging process in a unipolar diffusion charger with positive ions. It was found that the particles initially carrying negative charges have almost the same amount of positive charges as the initially uncharged particles after passing the test charger; and the particles initially carrying more positive charges have more final charges. An analytical solution of a model for particle charge distribution of initially charged particles was provided for unipolar charging based on Fuchs' theory and the birth-and-death theory. The N ion t value used in this model was obtained by fitting the experimental data of average charge on particles for initially uncharged particles. The results from the analytical solution show very good agreements with experimental data regarding the relationship between the pre-existing charge and the final charge on particles (50–200 nm in this study). Experimental tests of the response of Nanoparticle Surface Area Monitor (NSAM) against initially charged particles demonstrated that NSAM could have a large response deviation (more than 20% in the tested charge level) depending on the particle size and the amount of pre-existing positive charges on particles. Modeling of NSAM response showed similar deviation and predicted that when pre-existing charge is high enough, the NSAM response can be as large as 5 and 9 times of the uncharged particle response for alveolar and tracheobronchial surface area concentration, respectively.
1. INTRODUCTION
Electrical particle chargers are commonly used devices in aerosol measurement technology. Recently, unipolar diffusion chargers have raised increased attention, because the electrical current resulting from the deposition of the charged particles can be tuned to be proportional to either the particle length (TSI EAD model 3070A; CitationJung and Kittelson 2005a), the total active surface area (“Fuchs surface area”) of particles (Matter engineering, LQ1-DC; CitationJung and Kittelson 2005a), or the lung deposited surface area (TSI Nanoparticle Surface Area Monitor, NSAM model 3550 and Aerotrak 9000, CitationShin et al. 2007; CitationFissan et al. 2007). Particularly the possibility of measuring particle surface area is of major interest, because several researchers proposed particle surface area to be the most health relevant measure (CitationOberdörster et al. 2000; CitationDonaldson et al. 1998).
Recent advances in the design of unipolar chargers have furthermore led to more predictable charge distributions (CitationQi et al. 2007; CitationVivas et al. 2008) that can be exploited for the determination of particle size distributions. The newly introduced Ultrafine Particle Monitor (TSI UFP Monitor, model 3031) includes a unipolar charger to provide a known particle charge distribution prior to entering a differential mobility analyzer. CitationBiskos et al. (2005) developed a Differential Mobility Spectrometer (DMS), which includes a unipolar corona charger. In both instruments, particles are detected by means of either a single (UFP monitor) or an array of electrometers (DMS). Their reason for using a unipolar corona charger rather than a bipolar charger was the higher charging efficiency compared to bipolar diffusion charging and regulative limitations in the use of radioactive materials. The attempt of developing miniaturized unipolar charger has also been made for portable electrical aerosol instruments measuring personal exposure to nanoparticles (CitationQi et al. 2008). The Electrical Low Pressure Impactor (Dekati ELPI, CitationKeskinen et al. 1992) uses a unipolar charger prior to size segregated particle deposition in a low pressure cascade impactor. Each impaction stage of the ELPI is equipped with an electrometer and the current from the deposition of charged particles is measured to determine the number concentration of particles in the corresponding size range.
All aforementioned instruments measure a current and infer a particle concentration (number, length, or surface area) from it, assuming that the average number of elementary charges per particle is a known function of particle size. Charging efficiency and resulting charge distribution have been intensively studied for initially uncharged or neutralized particles; however, the potential effect of an initially asymmetric or even unipolar charge distribution has not yet been investigated. While equilibrium charge distribution can usually be assumed for aged atmospheric particles, freshly produced particles are commonly highly, often asymmetrically or unipolarly charged. Particle charge can be generated by a variety of different mechanisms, including thermal ionization (e.g., CitationBarnes 1934), collision with gaseous ions, and static electrification (CitationHinds 1999). Soot particles from incomplete hydrocarbon combustion often show high charge levels (CitationSodha et al. 1975; CitationBurtscher et al. 1986; CitationSavel'ev and Starik 2006). CitationJung and Kittelson (2005b) found that 60–80% of particles in the accumulation mode (peak diameter approximately 100 nm) of Diesel emission were charged. CitationMaricq (2006) investigated the electrical charge of exhaust particles from gasoline and Diesel powered vehicles and also found 60–80% of the particles to be charged with nearly equal amount of positively and negatively charged particles. With a Diesel particle filter, the charged fraction even increased to 90%. In all cases the charge distribution was in accordance with Boltzmann distribution at temperatures between 800 and 1100 K, i.e., particles could bear up to ±4 units of electrical charge. Similarly large charged fractions of charged particles were also observed in the exhaust of aircraft gas-turbines (CitationSorokin and Arnold 2004).
In the atmosphere, also aged particles can get charged in the presence of an ion source. Such sources may be of anthropogenic origin, such as AC powerlines (CitationFews et al. 1999) or electrical substations (CitationJ-Fatokun et al. 2008), or natural, e.g., thunder storms (CitationStolzenburg and Marshall 1998) or sea-spray (CitationBlanchard 1955, Citation1958; CitationReiter 1994).
This shows that the usually assumed equilibrium charge level with most particles being uncharged and an almost equal amount of positively and negatively charged particles cannot always be provided. Several processes during the particle generation or transmission can lead to particle charging and result in asymmetric or unipolar charge distribution. While processes in the atmosphere seem to produce rather lower charge levels, especially combustion processes can lead to very high levels of charged particles. In atmospheric air such particles can originate from engine or heating exhaust. In workplace air, these particles may originate from either synthesis of engineered nanoparticles using, e.g., flame pyrolisis or hot wall reactors, or from work activities like welding, soldering, and so on. The aim of the study presented here is to investigate the effect of pre-existing particle charges on unipolar diffusion charging. A model based on Fuchs theory (CitationFuchs 1963) was developed and experimentally verified using a unipolar diffusion charger that is implemented in three commercial instruments (TSI NSAM, model 3550/Aerotrak 9000, TSI EAD, model 3070A, and TSI UFP Monitor, model 3031).
2. THE EFFECT OF PARTICLE PRE-EXISTING CHARGE ON UNIPOLAR CHARGING
2.1. Experimental Setup
shows the experimental setup of producing test particles (NaCl) in the range of 20–200 nm using the atomizer-Differential Mobility Analyzer (DMA)–classification technique (CitationLiu and Pui 1974). The sheath air flow and sample flow in the DMA were 15.0 and 1.5 L/min, respectively. Particles exiting the DMA have the same electrical mobility, and almost all of them have the desired size set by the DMA with either positive or negative single charge depending on the voltage polarity applied on the DMA. Very few classified particles are larger than the desired one with more than one elementary charge on them. These multiple charged particles may complicate the subsequent charging experiment. In this study, we focused on particles equal to or larger than 50 nm. Hence we adjusted the atomized solution so that the size distributions of particles from the atomizer have the peak size smaller than 50 nm. This approach was reported by CitationRogak and Flagan (1992) to be effective to reduce the multiply-charged fraction. For example, 17.2% of 50 nm particles bear a single positive charge upon neutralization (CitationWiedensohler 1988). Doubly charged 73 nm have the same electrical mobility as singly charged particles and therefore also exit the DMA when the voltage is set to classify 50 nm particles. However, according to CitationWiedensohler (1988), the probability of 73 nm to bear two positive charges is only approximately 1.8%, higher charge levels are negligible. If 50 nm and 73 nm particles appeared in the same concentration, this means that approximately one out of ten classified particles would have been larger than 50 nm. However, the mode diameter of the produced particle size distribution was below 50 nm, i.e., the concentration of 73 nm particles was substantially lower than that of 50 nm particles and therefore below 10% of the classified particles were larger than 50 nm.
FIG. 1 Experimental Setup (a) generation system of test particles (b) test system for unipolar charging and NSAM response against particles carrying a defined number and polarity of pre-existing charges.
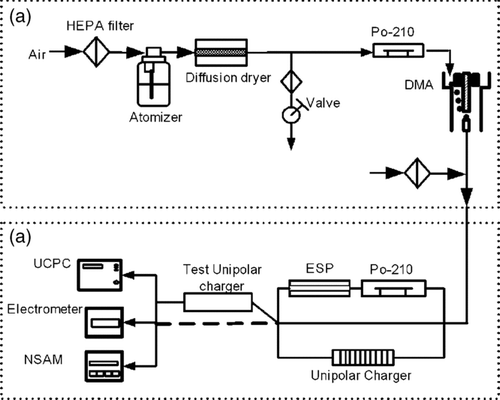
The particle number concentration in the classified aerosol ranged from 2,000 to 60,000 particles/cm3 depending on the particle size. This is a desired concentration range because it is high enough for a negligible statistical error (the sampling time was 1.0 minute in this study, so the highest statistical error was 0.4% when concentration was 2,000 particles/cm3) and it is much smaller than the ion concentration used in the studied charger (larger than 107 #/cm3), which is required in the charging model used in this study.
Since one main objective of this study was to investigate the effect of particle pre-existing charge, great effort was taken to precisely control the charge level on the test particles as shown in . Firstly, singly charged particles exiting the DMA were passed through a 210Po neutralizer and an electrostatic precipitator (ESP) to provide uncharged particles. The case of uncharged particles was used as a reference in this study to demonstrate the charge effect. To provide a wide range of charge levels on particles, a unipolar charger modified from the charger by CitationChen and Pui (1999) was used. Depending on the voltage polarity applied to this unipolar charger, test particles passing through it obtained multiple positive or negative charges. The charge level can be easily controlled by adjusting the voltage to the charger, which alters the ion concentration and hence the N ion t (the product of ion concentration and particle residence time) level inside. The average number of elementary charge on particles was used to examine the charging results in this study and it was obtained by measuring aerosol electrical current using an aerosol electrometer (model 3068, TSI Inc.) and particle number concentration using a ultrafine condensation particle counter (UCPC, model 3025A, TSI inc.).
The conditioned aerosol was then sent into the test unipolar charger, which is the corona diffusion charger installed, e.g., in NSAM. The operational flow rates for the downstream aerosol electrometer and the UCPC is 1.0 and 1.5 L/min, respectively. So the total flow rate passing through the test charger is 2.5 L/min, the same as the flow rate used in the corresponding commercial instruments. This charger delivers positive ions, generated from a corona wire, into a charging chamber by a turbulent jet and charges the sampled particles there by diffusion. Upstream of the charger, the total flow rate of 2.5 L/min is split. The flow rate of the ion jet is 1.0 L/min, so the rest of the sampling flow, i.e., 1.5 L/min, forms an aerosol jet. The total volume of the charging chamber is about 41.7 cm3, resulting in a particle residence time in the chamber of about 0.41 second. An ion trap, which has an electrical rod applied with a certain voltage, is installed downstream of the charging chamber. In our charging study, an ion trap voltage of 20 Volt was used to completely eliminate the highly mobile residual ions in the aerosol stream but ensure a negligible charged particle loss in the ion trap. This ion trap voltage, i.e., 20 Volt, is used for the same charger incorporated in the Electrical Aerosol Detector (EAD, model 3070A, TSI Inc.).
To accurately obtain the information of average charge on particles provided by the test unipolar charger, the charged particle loss in the charger needs to be minimized. Most unipolar chargers have an electrical field in the charging zone and it leads to substantial charged particle loss. The measurement of average charge or charge distribution can be made only for those charged particles that survived through the charger. There is no technique available to obtain information about charged particles lost in the charger. Hence, it is desirable to make such a measurement in a charger with negligible charged particle loss. The prototype of the test unipolar charger operating in a different flow rate was reported to have very high transmission efficiency due to the elimination of electrical field in the charging zone (CitationMedved et al. 2000). In this study, particle penetration in the test unipolar charger was also experimentally obtained to confirm the level of charged particle loss. The quantity, P flow , which corresponds to the particle loss in the charger due to diffusion and flow mixing, was obtained by measuring uncharged particle number concentrations downstream and upstream of the charger with the charger off; and P charger , which corresponds to the charged particle loss in the charger due to the space charge effect, was obtained by measuring particle number concentration downstream of the charger with the charger and the ion trap voltage on and off, respectively. Since the aerosol is actually diluted in the charging chamber by the ion jet flow, a dilution factor of 0.6, i.e., 1.5 L/min aerosol flow over 2.5 L/min total flow, was used when obtaining P flow .
2.2. Particle Penetrations in the Test Unipolar Charger
The experimental results of P flow and P charger using uncharged test particles are shown in . Both penetrations are above approximately 95% for particles larger than 50 nm. In this study, the measurement of average charge was made for particles of four different sizes, 50 nm, 100 nm, 150 nm, and 200 nm. Hence, the average charge obtained is believed to be very accurate and comparable with what is calculated from charging theories. It was suspected that P charger might be affected by the number of pre-existing charges because particles carrying more charges have higher electrical mobility and could be affected more by the same space charge level. However, such an effect is negligible in our observation for particles larger than 20 nm in the tested charge range. With the knowledge of P flow and P charger , we can easily obtain the actual particle penetration through this charger during operation, which is P total = P flow × P charger , and also illustrated in . The overall penetration is above approximately 90% for particles larger than 50 nm.
2.3. Average Charge on Particles after the Test Unipolar Charger for Initially Uncharged Particles
Initially uncharged particles obtain some charges after passing through the test unipolar charger. As mentioned previously, such information is the reference for investigating the effect of particle pre-existing charge, and is shown in . The relationship between average charge and particle diameter is linear in the tested size range. 200 nm particles obtained on average about 9 elementary charges. This result is very consistent with that obtained from the same charger used in Electrical Aerosol Detector (EAD, model 3070A, TSI Inc.) (CitationKaufman et al. 2002).
2.4. Average Charge on Particles after the Test Unipolar Charger for Initially Charged Particles
illustrates the results of average charge per particle after passing the test unipolar charger for particles carrying different number of pre-existing charges of both polarities. Obviously, the particles initially carrying negative charges have almost the same average charge after passing the charger as those initially uncharged particles. This is because the unipolar ions in the test charger are positive and they have very large combination coefficients with negatively charged particles due to the attractive Coulomb force. Hence, the negatively charged particles become uncharged almost immediately (time span is negligible compared to the total charging time) and then start charging as uncharged particles. The particles initially carrying more positive charges have more final charges. And it appears that the amount of pre-existing positive charge needs to reach a certain level to obviously observe an increase of final average charge, and this needed pre-existing charge increases with particle size. This is because the positively charged particles have smaller combination coefficients with positive ions due to the repulsive Coulomb force. When the pre-existing charge is smaller than a certain level, the reduced charging by smaller combination coefficients almost cancels out the addition from the pre-existing charge. However, when the pre-existing charge is close to or even over the charger's capacity for initially uncharged particles, the reduced charging cannot compensate the increased charge from pre-existing charge anymore. An obvious increase on final charge level can thus be observed. Larger uncharged particles obtain more charges in the charger, so they need a larger amount of pre-existing charge to see the increase of final charge level. To better understand this phenomenon, a model describing the charging process was developed and presented in the following section.
FIG. 4 Average number of elementary charge on particles after the test unipolar charger for initially charged particles.
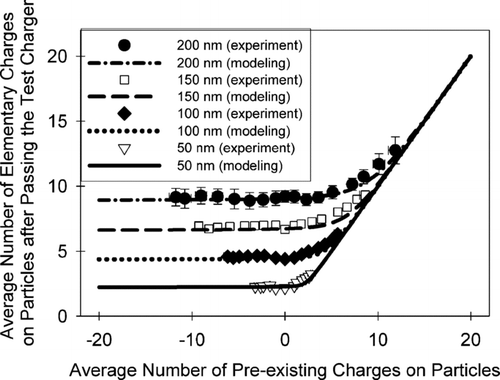
The result shown in suggests that those aerosol instruments involving unipolar chargers to deliver a predictable charge level may have a deflected response for initially charged particles. For example, the electrometer on each impaction stage of the Electrical Low Pressure Impactor (Dekati ELPI, CitationKeskinen et al. 1992) is used to measure the electrical current from the deposition of charged particles and determine the number concentration of particles on this stage assuming a well-defined average charge for each stage. When the sampled particles are initially charged with the same polarity as the unipolar charger used in ELPI, they may bear a substantially higher final average charge than the initially uncharged particles, thus produce a higher electrical current, leading to an overestimated number concentration.
2.5. Modeling of Unipolar Charging for Initially Charged Particles
Fuchs' theory (CitationFuchs 1963) has been widely used to calculate the combination coefficients of particles and ions because of its good agreements with various experimental studies (CitationAdachi et al. 1985; CitationRomay and Pui 1992; CitationReischl et al. 1996; CitationBiskos et al. 2005). The birth-and-death theory proposed by CitationBoisdron and Brock (1970) describes the approach usually used to solve particle charge distribution from unipolar charging process. Analytical solutions assuming that the initial particles are uncharged were also provided (CitationBoisdron and Brock 1970). An assumption for using the birth-and-death theory is that the particle number concentration is much lower than the ion number concentration, which is the case for this study as mentioned previously. For particles carrying a certain number of pre-existing charges, the basic equations for unipolar charging based on the birth-and-death theory can be written as follows:
In these equations, n denotes particle number concentration, N ion , the ion concentration, and β denotes the combination coefficient of a positive ion with a particle. The subscripts denote the number of elementary charge with its polarity and i is the number of pre-existing charge, which could be an integer between −∞ and +∞. Similar to the case of initially uncharged particles (CitationBoisdron and Brock 1970), the following analytical solutions can be obtained:
The average charge on particles can be easily computed by:
All the combination coefficients can be calculated from Fuchs' theory (CitationFuchs 1963). So the above equations can be simply solved with the knowledge of N ion t of the test charger, which, unfortunately, is not straightforward to measure accurately by experiment. This is because the test charger has a significantly turbulent flow due to two cross jets (CitationMedved et al. 2000), which is also very likely to lead to a very inhomogeneous ion distribution and incomplete mixing in the charging zone. Hence, we obtained the N ion t value by fitting the experimental results of initially uncharged particles as shown in . The ion properties used in the calculation are: ion mobility of 1.15 cm2/V-s and ion mass of 290 amu (CitationWiedensohler 1988). Obviously, a good fitting was obtained by setting the N ion t value at 2.5 × 107 s/cm3.
This fitting value was then used to calculate the average charge of initially charged particles following Equations (3–5). The modeling results are also presented in and show very good agreements with the experimental results for all four test sizes. This observation confirms that Fuchs' theory has a good level of accuracy regarding combination coefficient of positive ions with particles having a certain number of positive or negative charges. Also, for any aerosol instrument involving unipolar charging, a model can be developed based on the above one to examine the effect of particle pre-existing charge on the instrument's response, if N ion t values are known.
The modeling results in also suggest that when pre-existing positive charge is high enough, the final charge is almost the same as the pre-existing one. This is because the particles carrying a large number of positive pre-existing charge have too low combination coefficients with positive ions so that the charger can barely put any more positive charges onto them. Smaller particles have smaller combination coefficients, so they reach to such a level with a smaller pre-existing charge.
3. IMPLICATION: THE EFFECT OF PARTICLE PRE-EXISTING CHARGE ON NSAM RESPONSE
The test unipolar charger is an important component of the electrical aerosol instruments mentioned previously. The impact of particle pre-existing charges may be modified by other components in these instruments. In the following we describe the analysis needed to describe the pre-existing charge effect on the performance of the NSAM as an example. In this study, we examined NSAM response using particles carrying different number of pre-existing charges. Similar approach can be used to investigate such effects on any other aerosol instruments involving unipolar charging.
NSAM uses the studied unipolar charger to electrically charge the sampled particles. The positively charged aerosol with residual ions is then introduced into the ion trap. In NSAM, the ion trap is used to not only eliminate the residual ions but also remove some charged particles to match the response functions of different weightings of lung deposition. CitationFissan et al. (2007) found that when the ion trap voltage was set at 100 and 200 Volt, the NSAM response function for particles below 100 nm can fit the needed one for tracheobronchial and alveolar deposition, respectively, thus delivers lung deposited surface area concentrations according to the International Commission on Radiological Protection model (CitationICRP 1995). Obviously, the matched response functions for tracheobronchial and alveolar deposition are obtained by different charged particle loss in the ion trap, which is applied with different voltages.
3.1. Experimental Setup and Results
The experimental setup to examine NSAM response is also presented in . The methods of generating test particles and controlling charge levels are the same as those described in section 2.1. Differently from the charging experiment, test particles carrying different numbers of pre-existing charges were directly sampled by three instruments: the aerosol electrometer, UCPC and NSAM. The readings from the aerosol electrometer and UCPC were used to calculate the average charge on the test particles, and the corresponding NSAM response (either alveolar or tracheobronchial deposition) was recorded simultaneously.
The investigation was done by challenging NSAM using particles carrying different number of pre-existing charges as what we did in the section 2 for the charging study. It was found that NSAM response was not affected by particles initially carrying negative charges. This was observed for particles of all four sizes and was expected based on the results shown in . Hence, only the results for particles initially carrying positive charges were presented in and for alveolar and tracheobronchial deposition, respectively. As mentioned previously, the case of uncharged particles was used as a reference to demonstrate the charge effect. Hence, the NSAM responses were normalized against those for the uncharged particles, and are illustrated in .
FIG. 5 Experimental result of normalized NSAM response against the number of pre-existing charges on particles (a) alveolar deposition; (b) tracheobronchial deposition.
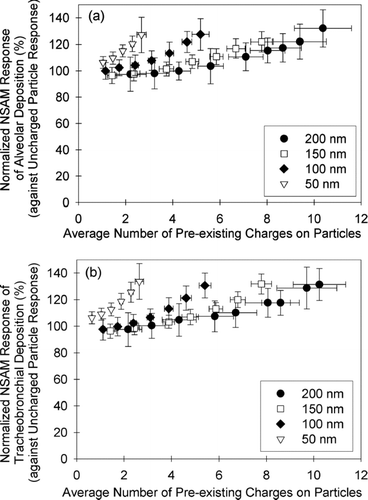
As shown in , the response deviation increases with the pre-existing positive charge on particles and can reach above 20% for all the sizes of test particles in the examined charge range. When carrying the same amount of pre-existing positive charges, smaller particles give larger response deviations. Similar to the observation in , the pre-existing positive charge on particles needs to reach a certain level so that the normalized response is obviously increased. This level increases with increasing particle size.
3.2. Development of a Semi-Empirical Model to Estimate the Effect of Particle Pre-Existing Charge on NSAM Response
The trends of experimental data shown in and are similar. But it is helpful to examine how they are quantitatively related. Also, it should be noted that the examined charge range in this study is limited by the unipolar charger used for controlling pre-existing charge levels, e.g., less than 3 elementary charges for 50 nm and about 12 elementary charges for 200 nm particles. These charge levels, however, are relatively low compared to Rayleigh limit, e.g., about 500 charges for 50 nm particles (CitationHinds 1999). In practical applications, much higher charge level than the examined one could be faced. Hence, it would be helpful to estimate the instrument's response against particles carrying higher charge levels.
NSAM converts the electrometer current reading into nanoparticle surface area deposited in human lung by using a calibration factor (CF) for alveolar and tracheobronchial depositions. Its response for monodisperse particles can be described by the following equation:
From Equation (Equation6), there are two competing mechanisms in NSAM affecting its response against initially charged particles. Particles carrying more pre-existing positive charges have higher C as shown in . But the increased C also increases particles' electrical mobility, leading to more losses in the ion trap of the NSAM thus a lower P ion − trap . Hence, the normalized NSAM response (NR) can be modeled as:
The subscripts denote the pre-existing charge on particles, i.e., 0 is the case of initially uncharged particles and i is the case of particles carrying i pre-existing charges.
The quantity, C, was solved by Fuchs' charging theory and the birth-death theory as described in section 2.5 and shown in . A simple experiment was carried out to characterize P ion − trap by using singly charged monodisperse particles of different sizes. The relationships between P ion − trap and particle electrical mobility for different ion-trap voltages (i.e., 100 and 200 Volt for tracheobronchial and alveolar deposition, respectively) are plotted in and appear to be linear (the plot is in log scale to view the data points better). This linear relationship is expected because the ion-trap acts as an electrical precipitator for the charged particles, the penetration of charged particles for such a rod-type electrical precipitator can be described as (CitationForsyth et al. 1998):
FIG. 6 Particle penetration through the NSAM Ion-trap against the electrical mobility of particles under different ion-trap voltages.
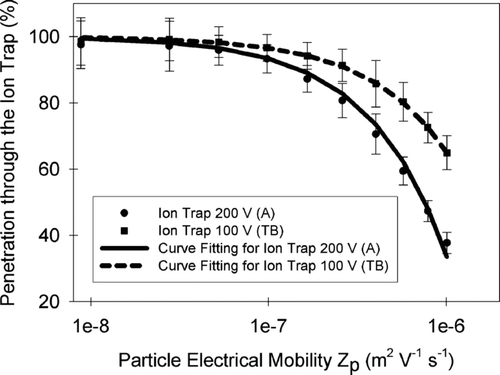
With this relationship, we can easily obtain P ion − trap for monodisperse particles carrying different number of charges. In this study, we calculated the charge distribution of particles using Equations (3) and (4), and then obtained the corresponding P ion − trap according to Equation (Equation8). Hence, a semi-empirical model was developed with all known information regarding the parameters in Equation (Equation7). Normalizing the results following Equation (Equation7), the NSAM response deviations from this semi-empirical model were illustrated in and for alveolar and tracheobronchial deposition, respectively. The experimental result presented in was also included for comparison. It can be seen that the modeling results on the NSAM response deviation agree with the experimental data reasonably well. The response can be increased as much as about 5 times for alveolar deposition and about 9 times for tracheobronchial deposition. The maximum charge level examined in the model is still smaller than Rayleigh limit.
In the modeling results shown in , it is interesting to observe that when pre-existing charge is over a certain amount, the NSAM response deviation starts decreasing. As explained previously, there are two mechanisms affecting NSAM deviation. Higher pre-existing charges gives more charges on particles downstream of the unipolar charger. This should lead to a higher electrical current. But when particles are carrying more charges, their electrical mobilities become larger and they will have more losses in the ion-trap, resulting in a lower electrical current. When pre-existing charge is less than a certain amount, the first mechanism dominates so we can see an increased deviation. But when pre-existing charge is over a certain value, the second mechanism starts taking over. When this happens, the whole NSAM is working more like an Electrostatic Precipitator, charging the particles and then removing them in the ion trap, thus there would be smaller electrical current output and a smaller NSAM reading.
4. CONCLUSION
The effect of particle pre-existing charge on unipolar charging was investigated. Experimental study using a unipolar diffusion charger with positive ions shows that the particles initially carrying negative charges have almost the same amount of positive charges as the initially uncharged particles after passing the charger, and the particles initially carrying more positive charges have more final charges. This result suggests that if the particles are either positively charged or have a very high charge level with the same amount of positive ones and negative ones, e.g., the case of engine exhaust particles (CitationJung and Kittelson 2005b; CitationMaricq 2006), the final average charge would be larger than the case of initially uncharged or neutralized (Boltzmann equilibrium at, e.g., 300 K) particles. The relationship between pre-existing charge and final charge on particles agree very well with the modeling results based on Fuchs' charging theory (50–200 nm in this study). It indicates that Fuchs' charging theory can be used to describe the unipolar charging process for initially charged particles, and the developed model can be used to predict the response deviation caused by particle pre-existing charges on aerosol instruments involving unipolar charging. Our experimental results demonstrate that NSAM could have a large response deviation (more than 20% in the tested relatively low charge level) against positively charged particles depending on the particle size and the amount of pre-existing charges. Modeling of NSAM response showed similar deviation and predicted that when pre-existing charge is high enough, the NSAM response can be as large as 5 and 9 times of the uncharged particle response for alveolar and tracheobronchial deposition, respectively. Similar approaches can be utilized in examining the response deviation of other electrical aerosol instruments against pre-existing charges on particles.
Acknowledgments
The authors are grateful for the financial support provided by the NSF Grant G2006-Star-F2.
REFERENCES
- Adachi , M. , Kousaka , Y and Okuyama , K. 1985 . Unipolar and Bipolar Diffusion Charging of Ultrafine Aerosol Particles . J. Aerosol Sci. , 16 : 109 – 122 .
- Barnes , L. L. 1934 . The Emission of Positive Ions from Heated Metals . Phys. Rev. , 42 : 487 – 491 .
- Biskos , G. , Reavell , K. and Collings , N. 2005 . Description and Theoretical Analysis of a Differential Mobility Spectrometer . Aerosol Sci. Technol. , 39 : 527 – 541 .
- Blanchard , D. C. 1955 . Electrified Droplets from the Bursting of Bubbles at an Air–Sea Interface . Nature. , 175 : 334 – 336 .
- Blanchard , D. C. 1958 . Electrically Charged Drops from Bubbles in Sea Water and their Meteorological Significance . J. Meteorol. , 15 : 383 – 396 .
- Boisdron , Y. and Brock , J. R. 1970 . On the Stochastic Nature of the Acquisition of Electrical Charge and Radioactivity by Aerosol Particles . Atmos. Environ. , 4 : 35 – 50 .
- Burtscher , H. , Reis , A. and Schmidt-Ott , A. 1986 . Particle Charge in Combustion Aerosols . J. Aerosol Sci. , 17 : 47 – 51 .
- Chen , D. R. and Pui , D. Y. H. 1999 . A High Efficiency, High Throughput Unipolar Aerosol Charger for Nanoparticles . J Nanoparticle Res. , 1 : 115 – 126 .
- Donaldson , K. , Li , X. Y. and MacNee , W. 1998 . Ultrafine (Nanometer) Particle Mediated Lung Injury . J. Aerosol Sci. , 29 : 553 – 560 .
- Fews , A. P. , Henshaw , D. L. , Wilding , R. J. and Keitch , P. A. 1999 . Corona Ions from Powerlines and Increased Exposure to Pollutant Aerosols . International J. Radiation Biology. , 75 : 1523 – 1531 .
- Fissan , H. , Neumann , S. , Trampe , A. , Pui , D. Y. H. and Shin , W. G. 2007 . Rationale and Principle of an Instrument Measuring Lung Deposited Nanoparticle Surface Area . J. Nanoparticle Res. , 9 : 53 – 59 .
- Forsyth , B. , Liu , B. Y. H. and Romay , F. J. 1998 . Particle Charge Distribution Measurement for Commonly Generated Laboratory Aerosols . Aerosol Sci. Technol. , 28 : 489 – 501 .
- Fuchs , N. A. 1963 . On the Stationary Charge Distribution on Aerosol Particles in Bipolar Ionic Atmosphere . Geofis. Pura Appl. , 56 : 185 – 193 .
- Hinds , W. C. 1999 . Aerosol Technology: Properties, Behavior, and Measurement of Airborne Particles , New York : John Wiley & Sons .
- ICRP . 1995 . “ International Commission on Radiological Protection Publication 66 Human Respiratory Tract Model for Radiological Protection ” . Oxford, Pergamon : Elsvier Science Ltd .
- J-Fatokun , F. O. , Jayaratu , E. R. , Morawska , L. , Rachman , R. and Birthwhistle , D. 2008 . Characterization of the Atmospheric Electrical Environment Near a Corona Ion-Emitting Source . Atm. Environ. , 42 : 1607 – 1616 .
- Jung , H. and Kittelson , D. B. 2005a . Characterization of Aerosol Surface Instruments in Transition Regime . Aerosol Sci. Technol. , 39 : 902 – 911 .
- Jung , H. and Kittelson , D. B. 2005b . Measurement of Electrical Charge on DIESEL PARTICLES . Aerosol Sci. Technol. , 39 : 1129 – 1135 .
- Kaufman , S. L. , Medved , A. , Pöcher , A. , Hill , N. , Caldow , R. and Quant , F. R. 2002 . “ An Electrical Aerosol Detector Based on the Corona-Jet Charger ” . Presented at 21st American Association for Aerosol Research Conference, Charlotte, NC.
- Keskinen , J. , Pietarinen , K. and Lehtimäki , M. 1992 . Electrical Low Pressure Impactor . J. Aerosol Sci. , 23 : 353 – 360 .
- Liu , B. Y. H. and Pui , D. Y. H. 1974 . A Submicrion Aerosol Standard and the Primary, Absolute Calibration of the Condensation Nuclei Counter . J. Coll. Interface Sci. , 47 : 155 – 171 .
- Maricq , M. 2006 . On the Electrical Charge of Motor Vehicle Exhaust Particles . J. Aerosol Sci. , 37 : 858 – 874 .
- Medved , A. , Dorman , F. , Kaufman , S. L. and Pocher , A. 2000 . A New Corona–Based Charger for Aerosol Particles . J. Aerosol Sci. , 31 : S616 – S617 .
- Oberdörster , G. 2000 . Toxicology of Ultrafine Particles. In Vivo Studies . Phil. Trans. R. Soc. Lond. A. , 358 : 2719 – 2740 .
- Qi , C. , Chen , D. R. and Pui , D. Y. H. 2007 . Experimental Study of a New Corona–Based Unipolar Aerosol Charger . J. Aerosol Sci. , 38 : 775 – 792 .
- Qi , C. , Chen , D. R. and Greenberg , P. 2008 . Performance Study of a Unipolar Aerosol Mini Charger for a Personal Nanoparticle Sizer . J. Aerosol Sci. , 39 : 450 – 459 .
- Reischl , G. P. , Makela , J. M. , Harch , R. and Necid , J. 1996 . Bipolar Charging of Ultrafine Particles in the Size Range Below 10 nm . J. Aerosol Sci. , 27 : 931 – 949 .
- Reiter , R. 1994 . Charges on Particles of Different Size from Bubbles of Mediterranean Sea Surf and from Waterfalls . J. Geophys. Res. , 99 : 10807 – 10812 .
- Rogak , S. and Flagan , R. 1992 . Bipolar Diffusion Charging of Spheres and Agglomerate Aerosol Particles . J. Aerosol Sci. , 23 : 693 – 710 .
- Romay , F. J. and Pui , D. Y. H. 1992 . On the Combination Coefficient of Positive Ions with Ultrafine Neutral Particles in the Transition and Free-Molecule Regime . Aerosol Sci. Technol. , 17 : 134 – 147 .
- Savel'ev , A. M. and Starik , A. M. 2006 . Interaction of Ions and Electrons with Nanoparticles in Hydrocarbon Combustion Plasmas . Technical Phys. , 51 : 444 – 452 .
- Shin , W. G. , Pui , D. Y. H. , Fissan , H. , Neumann , S. and Trampe , A. 2007 . Calibration and Numerical Simulation of Nanoparticle Surface Area Monitor (TSI model 3550 NSAM) . J. Nanoparticle Res. , 9 : 61 – 69 .
- Sodha , M. S. , Sagoo , M. S. , Chandra , A. and Ghatak , A. K. 1975 . Electrical Properties of Rich Hydrocarbon Flames . J. Applied Phys. , 46 : 3806 – 3808 .
- Sorokin , A. and Arnold , F. 2004 . Electrically Charged Small Soot Particles in the Exhaust of an Aircraft Gas-Turbine Engine Combustor . Atmos. Environ. , 38 : 2611 – 2618 .
- Stolzenburg , M. and Marshall , T. C. 1998 . Charged Precipitation and Electric Filed in Two Thunderstorms . J. Geophys. Res. , 103 : 19777 – 19790 .
- Vivas , M. M. , Hontanón , E. and Schmidt-Ott , A. 2008 . Reducing Multiple Charging of Submicron Aerosols in a Corona Diffusion Charger . Aerosol Sci. Technol. , 42 : 97 – 109 .
- Wiedensohler , A. 1988 . An Approximation of the Bipolar Charge Distribution for Particles in the Submicron Size Range . J. Aerosol Sci. , 19 : 387 – 389 .