Abstract
Human exposure to particulate matter (PM) air pollution is associated with human morbidity and mortality. The mechanisms by which PM impacts human health are unresolved, but evidence suggests that PM intake leads to cellular oxidative stress through the generation of reactive oxygen species (ROS). Therefore, reliable tools are needed for estimating the oxidant generating capacity, or oxidative load, of PM at high temporal resolution (minutes to hours). One of the most widely reported methods for assessing PM oxidative load is the dithiothreitol (DTT) assay. The traditional DTT assay utilizes filter-based PM collection in conjunction with chemical analysis to determine the oxidation rate of reduced DTT in solution with PM. However, the traditional DTT assay suffers from poor time resolution, loss of reactive species during sampling, and high limit of detection. Recently, a new DTT assay was developed that couples a particle-into-liquid-sampler with microfluidic-electrochemical detection. This “on-line” system allows high temporal resolution monitoring of PM reactivity with improved detection limits. This study reports on a laboratory comparison of the traditional and on-line DTT approaches. An urban dust sample was aerosolized in a laboratory test chamber at three atmospherically relevant concentrations. The on-line system gave a stronger correlation between DTT consumption rate and PM mass (R 2 = 0.69) than the traditional method (R 2 = 0.40) and increased precision at high temporal resolution, compared to the traditional method.
Copyright 2014 American Association for Aerosol Research
1. INTRODUCTION
Extensive research has established a link between airborne particulate matter (PM) exposure and increased morbidity and mortality in humans (Schlesinger Citation2007; Mauderly and Chow Citation2008). Epidemiologic evidence has associated PM exposure with health outcomes including myocardial infarction (Peters et al. Citation2001; Brook et al. Citation2010), asthma (Li et al. Citation2003a), birth defects (Ritz et al. Citation2002), and lung cancer (Dockery et al. Citation1993). Toxicological studies in animals and humans have observed elevations in cardiorespiratory inflammation (Fujii et al. Citation2002; Nurkiewicz et al. Citation2006; Becher et al. Citation2007), immune response (van Eeden et al. Citation2001; Becher et al. Citation2007; Mutlu et al. Citation2007; Tamagawa et al. Citation2008), and autonomic nervous system (ANS) imbalance (Rhoden et al. Citation2005; Ghelfi et al. Citation2008) resulting from both short- and long-term PM exposures. Mechanisms by which PM induces adverse health effects are unclear, yet evidence suggests multiple pathways. Proposed mechanisms include PM interference with lung receptors and nerves leading to dysfunction of the ANS (Stone and Godleski Citation1999; Timonen et al. Citation2006), ultrafine particle diffusion across alveolar membranes into the bloodstream (Nemmar et al. Citation2002), and excess generation of reactive oxygen species (ROS) by redox-active PM components (Squadrito et al. Citation2001; Sioutas et al. Citation2005). However, all of these proposed mechanisms are associated with ROS generation and oxidative stress in cells (Schafer and Buettner Citation2001; Brook et al. Citation2010). A prolonged state of cellular oxidative stress may initiate a cascade of inflammatory events leading to cellular damage, cell death, and subsequent disease (Li et al. Citation2002; Brook et al. Citation2010).
Ambient PM is a complex mixture of redox-active chemicals known to participate in various electron-transfer reactions (Kumagai et al. Citation1997; Veronesi et al. Citation1999; Wu et al. Citation1999); it has been shown to produce ROS both in vitro and in vivo (Alessandrini et al. Citation2009; Vidrio et al. Citation2009). Atmospheric PM also contains organic compounds known to induce cellular oxidative stress through ROS generation such as polycyclic aromatic hydrocarbons (PAHs) that are transformed into quinones both in the atmosphere and in the body (Kumagai et al. Citation1997; Kumagai et al. Citation2002; Cho et al. Citation2005; Chung et al. Citation2006) and metals (Liljelind et al. Citation2003). Therefore, a method for reliable measurement of PM redox activity (or oxidative load) is needed to advance our understanding of the role PM plays in human disease (De Vizcaya-Ruiz et al. Citation2006; Chahine et al. Citation2007; Ntziachristos et al. Citation2007). Chemical assays offer potential for describing the oxidative load of PM (Ichoku et al. Citation1999; Bernardoni et al. Citation2011). One approach is to analyze the chemical composition of PM directly to quantify species possessing redox-active moieties (Poschl Citation2005). However, characterization of specific PM components is costly, time consuming, and may miss important contributions, as not all redox-active species in PM are known. An alternative approach is to measure the redox activity of PM directly using solution-based methods. The most widely reported technique for measuring PM reactivity is the dithiothreitol (DTT) assay (Rappaport et al. Citation2003; Li et al. Citation2003b; Cho et al. Citation2005; De Vizcaya-Ruiz et al. Citation2006; Li et al. Citation2009b). The DTT assay is considered biologically relevant because the rate of DTT consumption has been correlated with cellular oxidative stress in vitro (Li et al. Citation2003b) and because several components of ambient PM (e.g., redox-active quinones) have been shown to catalyze the generation of superoxide radicals from DTT in solution (Kumagai et al. Citation2002).
However, the traditional solution-based DTT method has significant limitations including high detection limits that require relatively large PM sample masses (typically, 5–40 μg/mL in the DTT assay) (Cho et al. Citation2005; De Vizcaya-Ruiz et al. Citation2006). To collect sufficient mass generally requires long sample times (e.g., 10 days as in Charrier and Anastasio, Citation2012 or weekly samples as in Hu et al., Citation2008) or very high flow rates (e.g., the VACES system used by Cho et al., Citation2005) and results in a loss of temporal resolution that hinders our ability to identify sources and events responsible for redox-active PM emissions. The time lag between sample collection and analysis also poses a problem because some redox-active PM components are highly volatile and/or reactive (with half-lives ranging from minutes to hours [Kiruri et al. Citation2014]) and are likely lost or chemically altered during sample collection, transport, storage, and extraction (Foucaud et al. Citation2007; Hu et al. Citation2008). Extraction techniques used to separate particles from filters can also alter PM composition and reactivity estimates due to the wide range of solubilities and polarities present in PM constituents. Different solvents are known to extract reactive compounds with varied efficiency, leading to inconsistent measures of DTT reactivity (Truong et al. Citation2010; Rattanavaraha et al. Citation2011; McWhinney et al. Citation2013; Yang et al. Citation2014).
Recently, a novel electrochemical sensor for reduced DTT quantification was coupled to a particle-into-liquid-sampler (PILS) for on-line reactivity analysis (Sameenoi et al. Citation2012). This technique employed a cobalt(II) phthalocyanine (CoPC) working electrode integrated within a microfluidic device (Sameenoi et al. Citation2011) for highly sensitive and selective quantification of reduced DTT in micro-volumes of solution (). Compared with the traditional method, the CoPC electrodes improved detection of DTT by reducing the required sample mass (nanograms vs. micrograms) in reduced reaction volumes (10 μL vs. ∼1 mL). The CoPC electrodes were integrated within poly(dimethylsiloxane) (PDMS)–based microfluidic chips allowing for portable, inexpensive, and rapid monitoring of DTT consumption by PM (Sameenoi et al. Citation2012). The objective of the present study was to compare the PILS-CoPC microfluidic chip with the traditional DTT method for monitoring oxidative load of airborne PM at higher temporal resolution than typically used for DTT analysis (i.e., a 3-h sampling duration).
FIG. 1 (a) Picture of a PDMS microfluidic chip infused with CoPC electrodes for sensing reduced DTT quantity in solution. (b) Chip schematic, 10 μL injections of the DTT + PM solution enter the inlet and are pushed through the flow channel by a syringe pump. CoPC electrodes measure electrical conductivity of the solution at a potential of +200 mV. Conductivity of the solution is directly proportional to DTT concentration.
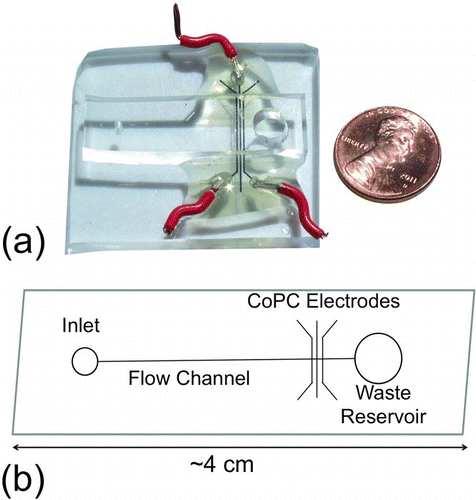
2. EXPERIMENTAL SECTION
2.1. Chamber Testing
A standard reference aerosol (urban dust; NIST SRM 1649b, Washington D.C., USA) was nebulized (Collison Nebulizer; BGI, Waltham, MA, USA) in water (deionized water was used for all solutions) to generate three atmospherically relevant concentrations (nominally 60, 30, 15 μg/m3) within a ∼1 m3 test chamber (). The test aerosol is representative of PM typically found in urban air and is composed of chemical species correlated with DTT consumption including transition metals (Costa and Dreher Citation1997), PAHs, chlorinated pesticides, and polychlorinated biphenyl's (Wise and Watters Citation2009). Aerosol was dispersed at constant periodicity throughout a well-mixed acrylic chamber and diluted with filtered, compressed air. The mass concentration within the chamber was continuously monitored with a DustTrak (TSI Model #8250, Shoreview, MN, USA) that was calibrated by a gravimetric reference sample. Particle size distribution within the chamber was monitored using a sequential mobility particle sizer (SMPS; Grimm Technologies, Douglasville, GA, USA). Aerosol sampling and DTT reactivity analyses were performed in parallel to compare the traditional DTT method with the electrochemical approach. Chamber PM was sampled and analyzed continuously by the electrochemical monitoring system and, concurrently, filters were collected for the traditional DTT method. Three tests were performed at each concentration level for nine total experiments; each experiment included at least three sample replicates (i.e., three filters extracted for traditional DTT analysis and at least three injections into the electrochemical system).
FIG. 2 Schematic of the experimental setup. Aerosolized urban dust was generated at high, middle, and low concentration levels. A fan, dilution/exhaust manifold and timing switch were installed to mix PM and hold mass concentration steady. Reactivity analysis was performed by both the traditional and on-line techniques in parallel for comparison.
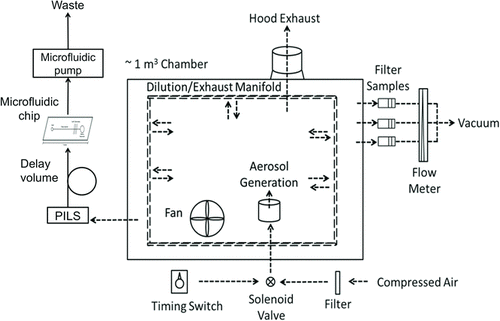
2.2. Traditional DTT Method
2.2.1. PM Sampling and Handling
Urban dust was collected for 180 min onto 37-mm Teflon-coated glass fiber filters (Pall Corp., Port Washington, NY, USA). For tests with nominal chamber concentrations of 60 or 30 μg/m3, filters were placed in closed-faced 37-mm sampling cassettes operated at 10 L/min. Low concentration (15 μg/m3) tests required a higher sampling rate to collect sufficient sample mass for analysis using the traditional DTT method. Therefore, sampling cassettes were operated in the open-faced configuration and PM was collected at 20 L/min. Two laboratory blanks were carried for each test. Following gravimetric analysis, sample and blank filters were transferred into 15 mL centrifuge tubes and mixed with 3 mL methanol for extraction. Preliminary reactivity tests of the urban dust found that DTT consumption rates were approximately twice as high with methanol extraction compared to water extractions (see in the online supplementary information [SI]), consistent with other studies that suggested the reactive species are better extracted with methanol (Wise and Watters Citation2009; Yang et al. Citation2014). However, other studies have found that the reactivity of the water-soluble fraction was higher for certain aerosol types, even for a different urban dust reference standard (McWhinney et al. Citation2013). Filter extracts were sonicated for 90 min and then cooled at room temperature (approximately 22°C) for a minimum of 60 min. Extracts were then syringe filtered (Acrodisc Glass Fiber, 1-μm pore size, 25-mm diameter filter, Pall Corp., Port Washington, NY, USA) into a clean centrifuge tube and concentrated under nitrogen gas (N2) to a final volume of 2 mL. The additional concentration step was necessary to allow sufficient mass to be added to the assay given the reduced PM mass on the filters at relatively short sampling times (3 h). Even with these additional steps, our assay typically contained 0.3–0.8 μg/mL in the DTT assay, a factor of 6–100 less than used in other DTT studies (Cho et al. Citation2005; De Vizcaya-Ruiz et al. Citation2006).
The mass of PM added to each assay, M assay, was calculated by:
2.2.2. DTT Assay Procedure
The DTT assay was conducted similar to procedures outlined by Cho et al. (Citation2005) with additional treatment of the phosphate buffer with Chelex resin (Rappaport et al. Citation2003). Chelex treatment improves assay repeatability by removing trace transition metals from stock buffers and lowering background DTT oxidation (Rappaport et al. Citation2003). The traditional DTT assay measures the oxidation of reduced DTT to its disulfide form over time (Kumagai et al. Citation2002). Oxidation of DTT is typically reported either as a raw rate (pmol DTT/min) or a mass-normalized rate (pmol DTT/min/mass PM).
PM was allowed to react with DTT in solution for a specified period of time (0, 5, or 10 min) and then the remaining unreacted (reduced) DTT was quenched by an addition of Ellman's reagent (5,5′-dithibis-2-nitrobenzoic acid or DTNB) in molar excess. The reaction of DTT with DTNB produced a yellow chromophore (5-mercapto-2-nitrobenzoic acid or TNB), in direct proportion to the amount of reduced DTT remaining in solution. The chromophore was quantified with UV/Vis spectroscopy and the rate of DTT consumption, ΔDTT was calculated as:
For comparison with other studies, urban dust reactivity was reported both in terms of raw DTT consumption rate (DTT amount consumed/min) and DTT consumption rate normalized by PM mass in the reaction volume. Raw DTT consumption rate was calculated as the linear decline in TNB absorbance over time minus the corresponding decrease for a similarly treated sample blank. The blank-corrected slope, , was converted to units of DTT amount (nmol) consumed based on a calibration curve of absorbance measurements from stock DTT solutions prepared via serial dilution.
2.3. On-Line DTT Monitoring
2.3.1. System Design
The on-line reactivity monitoring system was designed to collect PM directly into a solution containing DTT, with subsequent continuous measurement of reduced DTT concentration using electrochemical detection (). Aerosol was collected into solution using a Particle-Into-Liquid-System (PILS; ADI 2081, Metrohm, Riverview, FL, USA). The PILS samples ambient PM and immediately mixes the sampled aerosol stream with a turbulent flow of steam (∼1.5 mL/min of deionized water at 100°C). The water-saturated aerosol rapidly cools as it travels through the PILS, creating a supersaturated flow. Vapor deposits on the particles, so that they are large enough to impact onto a plate at the back of the PILS. A buffered reagent solution was introduced into the PILS that carried PM out through a liquid exit. The non-chelated buffer solution contained 50 μM of DTT and 100 μM of sodium fluoride (NaF), which was used as an internal standard. A T-junction immediately split the PM + DTT reaction mixture into equal-volume sample lines using a multichannel peristaltic pump (Ecoline, IDEX Health and Science, Glattbrug, Switzerland). One sample line was collected for ion chromatography (IC) to measure F− ion concentration as an internal standard to account for DTT dilution due to mixing with PM condensate. The second sample line was pumped downstream through a long tube to provide an approximately 18-min residence time for reaction between the PM and DTT, then 10 uL of the solution was injected to a CoPC microfluidic chip for electrochemical analysis. The temperature decreases from approximately 37°C at the PILS impaction plate to room temperature at the microfluidic chip. Sameenoi et al. (Citation2012) found that the temperature dependence of the DTT assay over this range was minimal.
The electrochemical current signal for reduced (unreacted) DTT from each injection was measured amperometrically using a commercially available potentiostat (CHI812, CH Instruments, Austin, TX, USA) (Sameenoi et al. Citation2012). Peak height of DTT oxidation current was measured and related to DTT mass based on a calibration curve constructed from direct injection of reduced DTT. Raw consumption rate, ΔDTT, was calculated with EquationEquation (3):
FIG. 3 Aerosolized urban dust size distribution (count fraction) averaged over three test replicates; one from each concentration level (high, middle, low). Error bars represent one standard deviation.
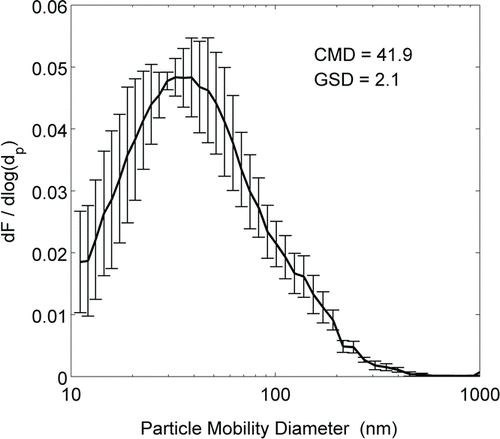
FIG. 4 Correlation diagrams of raw DTT consumption versus PM mass for each experiment. Estimates for slope, intercept, and Pearson correlation coefficients are shown for (a) on-line detection of raw DTT consumption versus M injection and (b) traditional measurement of raw DTT consumption versus M assay. Error bars represent (a) one standard deviation of reactivity for multiple injections in the microfluidic chip and (b) one standard deviation of reactivity for triplicate measurements of three collected filters per experiment on the y-axis and one standard deviation of PM mass per assay among the three collected filter masses per experiment on the x-axis.
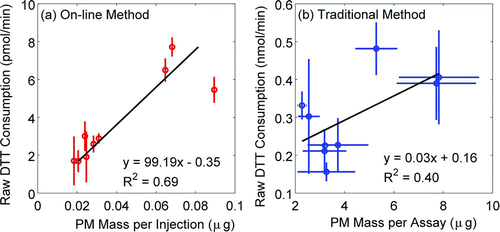
2.4. Statistical Analysis
A 3 × 2 factorial experiment (high, middle, and low PM concentrations, traditional, and on-line DTT monitoring) was conducted to evaluate the two measurement techniques. All statistical analyses performed with Matlab 2013a (The MathWorks Inc., Natick, MA, USA) and SAS 9.2 (SAS Institute Inc., Cary, NC, USA). The linear relationship between raw DTT consumption rate and assay or injection PM mass for each measurement technique were made using Deming regression to account for uncertainty in both the injection/assay mass and the reactivity values. Due to differences in PM mass between the measurement approaches, direct comparison of the two methods was made only in terms of mass-normalized DTT consumption rate. Mass-normalized reactivity was modeled as a function of chamber concentration level (high, middle, and low) and DTT measurement approach using PROC MIXED. The mixed model was constructed using a random statement to account for repeated measures at each injection mass by the on-line system and unbalanced data. An lsmeans statement was used to make pairwise comparisons between the on-line and traditional techniques at each concentration level.
3. RESULTS
The size distribution of aerosolized urban dust was similar across all concentration levels and is shown in . The urban dust size distribution was approximately lognormal with mass median diameter (MMD) of 215 nm, a count median diameter of 42 nm, and geometric standard deviation of 2.1.
3.1. Raw DTT Consumption Rates
Raw DTT consumption rates detected by both DTT analysis techniques, as a function of PM mass per injection, M injection, or mass per assay, M assay, are shown in . Raw DTT consumption rates measured by the on-line system strongly correlated with M injection (R 2 = 0.69) with a small and statistically insignificant intercept. The traditional DTT method detected a significant linear relationship between raw DTT consumption rate and M assay (R 2 = 0.40) and a small, but statistically significant intercept (b = 0.16 pmol DTT, 95% CI [0.08 0.28]). Stronger correlations were observed with the on-line system despite the significantly smaller PM mass quantities than observed for the traditional method. Sample mass per on-line injection, M injection, averaged 38 ± 23 ng (one standard deviation). Concentrated PM mass per traditional assay, M assay, was nearly three orders of magnitude larger and averaged 4.3 ± 2.2 μg (one standard deviation) and ranged from 1.9 to 9.3 μg.
3.2. Mass-Normalized DTT Consumption Rate
Box-whisker plots comparing mass-normalized DTT consumption rates by each technique versus chamber concentration as a categorical variable are shown in . The boxplots show median, quartile, and 95% confidence limits of the mean DTT consumption rate for each chamber concentration level. The on-line system suggested a characteristic DTT consumption rate of 92.7 ± 5.7 pmol DTT/min/μgpm for urban dust and showed no significant difference between concentration levels. Mass normalization of filter-based DTT analysis by M assay also demonstrated overlapping consumption rates between concentration levels (p > 0.08). The traditional method suggested a characteristic DTT consumption rate of 80.4 ± 7.0 pmol DTT/min/μgpm for urban dust, not statistically significantly different than suggested by the on-line method. When stratified by chamber concentration, the on-line system yielded similar average mass-normalized DTT consumption rates as the traditional method at all concentration levels ().
TABLE 1 Pairwise comparisons of mass-normalized DTT consumption rate estimates for each method, separated by concentration level
4. DISCUSSION
This study demonstrated the utility of the on-line monitoring approach for aerosol reactivity and the difficulties associated with the traditional DTT approach when higher temporal resolution is sought (3 h). The on-line monitoring system exhibited improved performance compared with the traditional method with limited PM mass, as demonstrated by multiple metrics. A stronger linear correlation was observed between raw DTT consumption rate and PM mass by the on-line system compared with the traditional assay (represented as both the airborne chamber concentration, not shown, and the mass in the reaction volume). For mass-normalized data, on-line measurements were more precise than corresponding traditional measures at all concentration levels and most experimental replicates (±5.7 overall SE on-line vs. ±7.0 SE traditional). Coefficients of variation for replicate measures of reactivity at the same concentration level by the traditional method (37–47%) were higher than reported by Sauvain et al. (Citation2012) likely due to the additional handling steps required to increase sample mass for the traditional method at low sampling times. The variability of the on-line measurements increased as PM mass decreased (the coefficient of variation increased from 12% at the high concentration to 75% at the low concentration), suggesting that an aerosol concentration of 10 μg/m3 for this aerosol sample (corresponding to approximately 2 pmol DTT consumed per minute) is near the limit of detection (LOD) for this technique at these sample/buffer flow rates. The LOD also depends on aerosol composition. Future work will examine whether optimization of the sensor electrode and/or modulation of system flow rates (both PILS and sample buffer) can increase detection sensitivity.
Injection masses measured by the on-line system ranged from 20 to 500 times less than masses used in the traditional assay. This is because the on-line system injection volume was reduced to 10 μL opposed to a reaction volume of 600 μL used for traditional analysis. On-line monitoring permitted near-continuous analysis of PM reactivity. Injections were analyzed at ∼3 min intervals, while each traditional DTT measurement described a 3-h filter time-weighted average.
Reduced precision of the DTT assay by the traditional method is likely due to filter handling, extraction, and concentration steps. These steps were necessary to give reactivity above the level of the blanks, when low PM masses were collected. Filter samples were processed individually; therefore, the magnitude of loss was random. Estimates of raw DTT consumption rates of traditional sample extracts may have been accurate; however, correlation with uncertain PM masses would weaken linear strength suggesting assay variability due to random error. In comparison, the PILS collection method uses a contained system likely reducing PM losses and resulting in stronger correlations between reactivity and PM mass and consistently higher mass-normalized reactivity estimates. The on-line system also employed a DTT dilution factor using the internal standard (F−) providing more accurate estimates of PM mass per injection.
4.1. Limitations
Several differences in PM handling between the traditional and on-line systems should be noted. In Sameenoi et al. (Citation2012), relatively high concentrations of PM extract solutions were used to compare the traditional assay to the electrochemical chip. As a result, Sameenoi et al. found good agreement between the electrochemical method (operated offline) and the traditional DTT method. In this study, the goal was to operate the electrochemical method on-line (taking advantage of its time-resolved capability). At relatively short sampling times (3 h sampling at either 10 or 20 L/min of flow), we needed to increase the sensitivity of the traditional method when sampling relatively low PM mass concentrations. Therefore, several additional experimental steps were conducted to increase the traditional assay sensitivity above that of the blanks. First, we extracted the filters in methanol, as preliminary tests (see the SI) showed an ∼102% increase in PM reactivity using methanol (as opposed to water). Several groups have reported similar findings with methanol (Wise and Watters Citation2009; Yang et al. Citation2014), although it may depend on aerosol type (McWhinney et al. Citation2013). Second, a sample concentration step was added to increase the extract concentration prior to DTT analysis. Although this step may have increased the variability between replicates, it also increased the relative reactivity of the traditional assay by a factor of 2. Only buffer was supplied to the on-line system; however, methanol could be added to the solution in future studies. The filter extracts were also filtered prior to the addition to the DTT solution to remove any insoluble particles, as is often done for the DTT assay (Li et al. Citation2009a; Sameenoi et al. Citation2012). The on-line system does not have a filtering step. The effect of removing the insoluble PM on reactivity is unclear but may have increased the reactivity measured by the on-line system. We treated the buffer for the traditional assay with Chelex to reduce sample variability according to literature protocols (Charrier and Anastasio Citation2012) but did not treat the buffer for the on-line system. This last difference should have a minimal effect on reported reactivity levels, since both blanks and samples were handled consistently within a given method (any residual contamination would be accounted though blank correction, which was done for both methods). Finally, the temperature of the traditional system was maintained at 37°C, but the temperature in the on-line system decreased from ∼40°C at the exit of the PILS impaction plate to room temperature at the microfluidic chip. Sameenoi et al. (Citation2012) found that the temperature dependence of the DTT assay over this range was very small, so we do not expect this difference to have a strong result, but would tend to increase the reactivity in the traditional assay.
After implementing methodological changes to ensure reactivity above the level of the blanks in the traditional assay, the on-line system detected similar mass-normalized reactivity of aerosolized urban dust than the traditional method but with greater precision. Our results suggest that the on-line system is better able to assess PM reactivity at high temporal resolution than the traditional assay. Both methods indicated higher mass-normalized reactivity for this urban dust sample (SRM 1649b) compared to another standard reference material (SRM 1648a, <5 pmol DTT/min/μgpm) (McWhinney et al. Citation2013). These PM reference samples were collected in different cities and may reflect the high variability of ambient particle reactivity by location and day (Cho et al. Citation2005; Sameenoi et al. Citation2013).
5. CONCLUSIONS
A laboratory comparison of two systems to measure PM oxidative load was completed. A PILS was coupled with a CoPC-microfluidic chip to permit continuous, on-line measurement of DTT consumption by PM. The on-line system outperformed traditional techniques, when limited by sampling time, measuring the reactivity standardized urban dust in several regards. The on-line system more strongly correlated raw DTT consumption rate with injected PM mass and the on-line data were less variable than corresponding traditional measures for both raw and normalized DTT consumption measures. The on-line system improved DTT monitoring from reduced sample quantities, reaction volumes, and PM handling time compared to the traditional technique. These results suggest on-line monitoring for DTT reactivity may provide an improved tool for researchers investigating the impact of PM on human health.
FUNDING
Research reported in this publication was supported by the National Institute of Environmental Health Sciences under award number R21ES019264. The content is solely the responsibility of the authors and does not necessarily represent the official views of the National Institutes of Health.
SUPPLEMENTAL MATERIAL
Supplemental data for this article can be accessed on the .
Supplementary_Information.zip
Download Zip (241.3 KB)REFERENCES
- Alessandrini , F. , Beck-Speier , I. , Krappmann , D. , Weichenmeier , I. , Takenaka , S. Karg , E. 2009 . Role of Oxidative Stress in Ultrafine Particle-induced Exacerbation of Allergic Lung Inflammation . Am. J. Resp. Crit. Care, , 179 : 984 – 991 .
- Becher , R. , Bucht , A. , Ovrevik , J. , Hongslo , J. K. Dahlman , H. J. 2007 . Involvement of NADPH Oxidase and iNOS in Rodent Pulmonary Cytokine Responses to Urban Air and Mineral Particles . Inhal. Toxicol., , 19 : 645 – 655 .
- Bernardoni , V. , Cuccia , E. , Calzolai , G. , Chiari , M. , Lucarelli , F. Massabo , D. 2011 . ED-XRF Set-up for Size-Segregated Aerosol Samples Analysis . X-Ray Spectrom., , 40 : 79 – 87 .
- Brook , R. D. , Rajagopalan , S. , Pope , C. A. , Brook , J. R. , Bhatnagar , A. Diez-Roux , A. V. 2010 . Particulate Matter Air Pollution and Cardiovascular Disease: An Update to the Scientific Statement From the American Heart Association . Circulation, , 121 : 2331 – 2378 .
- Chahine , T. , Baccarelli , A. , Litonjua , A. , Wright , R. O. , Suh , H. Gold , D. R. 2007 . Particulate Air Pollution, Oxidative Stress Genes, and Heart Rate Variability in an Elderly Cohort . Environ. Health Perspect., , 115 : 1617 – 1622 .
- Charrier , J. G. and Anastasio , C. 2012 . On Dithiothreitol (DTT) as a Measure of Oxidative Potential for Ambient Particles: Evidence for the Importance of Soluble Transition Metals . Atmos. Chem. Phys., , 12 : 9321 – 9333 .
- Cho , A. K. , Sioutas , C. , Miguel , A. H. , Kumagai , Y. , Schmitz , D. A. Singh , M. 2005 . Redox Activity of Airborne Particulate Matter at Different Sites in the Los Angeles Basin . Environ. Res., , 99 : 40 – 47 .
- Chung , M. Y. , Lazaro , R. A. , Lim , D. , Jackson , J. , Lyon , J. Rendulic , D. 2006 . Aerosol-Borne Quinones and Reactive Oxygen Species Generation by Particulate Matter Extracts . Environ. Sci. Technol., , 40 : 4880 – 4886 .
- Costa , D. L. and Dreher , K. L. 1997 . Bioavailable Transition Metals in Particulate Matter Mediate Cardiopulmonary Injury in Healthy and Compromised Animal Models . Environ. Health Perspect., , 105 : 1053 – 1060 .
- De Vizcaya-Ruiz , A. , Gutierrez-Castillo , M. E. , Uribe-Ramirez , M. , Cebrian , M. E. , Mugica-Alvarez , V. Sepulveda , J. 2006 . Characterization and In Vitro Biological Effects of Concentrated Particulate Matter from Mexico City . Atmos. Environ., , 40 : S583 – S592 .
- Dockery , D. W. , Pope , C. A. , Xu , X. P. , Spengler , J. D. , Ware , J. H. Fay , M. E. 1993 . An Association Between Air-Pollution and Mortality in 6 United-States Cities . New Engl. J. Med., , 329 : 1753 – 1759 .
- Foucaud , L. , Wilson , M. R. , Brown , D. M. and Stone , V. 2007 . Measurement of Reactive Species Production by Nanoparticles Prepared in Biologically Relevant Media . Toxicol. Lett., , 174 : 1 – 9 .
- Fujii , T. , Hayashi , S. , Hogg , J. C. , Mukae , H. , Suwa , T. Goto , Y. 2002 . Interaction of Alveolar Macrophages and Airway Epithelial Cells Following Exposure to Particulate Matter Produces Mediators That Stimulate the Bone Marrow . Am. J. Resp. Cell. Mol., , 27 : 34 – 41 .
- Ghelfi , E. , Ramos-Rhoden , C. , Wellenius , G. A. , Lawrence , J. and Gonzalez-Flecha , B. 2008 . Cardiac Oxidative Stress and Electrophysiological Changes in Rats Exposed to Concentrated Ambient Particles are Mediated by TRP-Dependent Pulmonary Reflexes . Toxicol. Sci., , 102 : 328 – 336 .
- Hu , S. , Polidori , A. , Arhami , M. , Shafer , M. M. , Schauer , J. J. Cho , A. 2008 . Redox Activity and Chemical Speciation of Size Fractioned PM in the Communities of the Los Angeles-Long Beach Harbor . Atmos. Chem. Phys., , 8 : 6439 – 6451 .
- Ichoku , C. , Andreae , M. O. , Andreae , T. W. , Meixner , F. X. , Schebeske , G. Formenti , P. 1999 . Interrelationships Between Aerosol Characteristics and Light Scattering During Late Winter in an Eastern Mediterranean Arid Environment . J. Geophys. Res.-Atmos., , 104 : 24371 – 24393 .
- Kiruri , L. W. , Khachatryan , L. , Dellinger , B. and Lomnicki , S. M. 2014 . Effect of Copper Oxide Concentration on the Formation and Persistency of Environmentally Persistent Free Radicals (EPFRs) in Particulates . Environ. Sci. Technol. , 48 ( 4 ) : 2212 – 2217 .
- Kumagai , Y. , Arimoto , T. , Shinyashiki , M. , Shimojo , N. , Nakai , Y. Yoshikawa , T. 1997 . Generation of Reactive Oxygen Species During Interaction of Diesel Exhaust Particle Components with NADPH-Cytochrome P450 Reductase and Involvement of the Bioactivation in the DNA Damage . Free Radical Bio, Med., , 22 : 479 – 487 .
- Kumagai , Y. , Koide , S. , Taguchi , K. , Endo , A. , Nakai , Y. Yoshikawa , T. 2002 . Oxidation of Proximal Protein Sulfhydryls by Phenanthraquinone, A Component of Diesel Exhaust Particles . Chem. Res. Toxicol., , 15 : 483 – 489 .
- Li , N. , Hao , M. Q. , Phalen , R. F. , Hinds , W. C. and Nel , A. E. 2003a . Particulate Air Pollutants and Asthma - A Paradigm for the Role of Oxidative Stress in PM-Induced Adverse Health Effects . Clin. Immunol., , 109 : 250 – 265 .
- Li , N. , Kim , S. , Wang , M. , Froines , J. , Sioutas , C. and Nel , A. 2002 . Use of a Stratified Oxidative Stress Model to Study the Biological Effects of Ambient Concentrated and Diesel Exhaust Particulate Matter . Inhalat. Toxicol., , 14 : 459 – 486 .
- Liljelind , I. , Rappaport , S. , Eriksson , K. , Andersson , J. , Bergdahl , I. A. Sunesson , A. L. 2003 . Exposure Assessment of Monoterpenes and Styrene: A Comparison of Air Sampling and Biomonitoring . Occup. Environ. Med., , 60 : 599 – 603 .
- Li , N. , Sioutas , C. , Cho , A. , Schmitz , D. , Misra , C. Sempf , J. 2003b . Ultrafine Particulate Pollutants Induce Oxidative Stress and Mitochondrial Damage . Environ. Health Perspect., , 111 : 455 – 460 .
- Li , N. , Wang , M. Y. , Bramble , L. A. , Schmitz , D. A. , Schauer , J. J. Sioutas , C. 2009a . The Adjuvant Effect of Ambient Particulate Matter Is Closely Reflected by the Particulate Oxidant Potential . Environ. Health Perspect., , 117 : 1116 – 1123 .
- Li , Q. F. , Wyatt , A. and Kamens , R. M. 2009b . Oxidant Generation and Toxicity Enhancement of Aged-Diesel Exhaust . Atmos. Environ. , 43 : 1037 – 1042 .
- Mauderly , J. L. and Chow , J. C. 2008 . Health Effects of Organic Aerosols . Inhal Toxicol., , 20 : 257 – 288 .
- McWhinney , R. D. , Badali , K. , Liggio , J. , Li , S.-M. and Abbatt , J. P. D. 2013 . Filterable Redox Cycling Activity: A Comparison Between Diesel Exhaust Particles and Secondary Organic Aerosol Constituents . Environ. Sci. Technol., , 47 : 3362 – 3369 .
- Mutlu , G. M. , Green , D. , Bellmeyer , A. , Baker , C. M. , Burgess , Z. Rajamannan , N. 2007 . Ambient Particulate Matter Accelerates Coagulation via an IL-6-Dependent Pathway . J. Clin. Invest., , 117 : 2952 – 2961 .
- Nemmar , A. , Hoet , P. H. M. , Vanquickenborne , B. , Dinsdale , D. , Thomeer , M. Hoylaerts , M. F. 2002 . Passage of Inhaled Particles into the Blood Circulation in Humans . Circulation, , 105 : 411 – 414 .
- Ntziachristos , L. , Froines , J. R. , Cho , A. K. and Sioutas , C. 2007 . Relationship Between Redox Activity and Chemical Speciation of Size-Fractionated Particulate Matter . Particle Fibre Toxicol., , 4, Article 5
- Nurkiewicz , T. R. , Porter , D. W. , Barger , M. , Millecchia , L. , Rao , K. M. K. Marvar , P. J. 2006 . Systemic Microvascular Dysfunction and Inflammation After Pulmonary Particulate Matter Exposure . Environ. Health Perspect., , 114 : 412 – 419 .
- Peters , A. , Dockery , D. W. , Muller , J. E. and Mittleman , M. A. 2001 . Increased Particulate Air Pollution and the Triggering of Myocardial Infarction . Circulation, , 103 : 2810 – 2815 .
- Poschl , U. 2005 . Atmospheric Aerosols: Composition, Transformation, Climate and Health Effects . Angew. Chem. Int. Edit., , 44 : 7520 – 7540 .
- Rappaport , S. M. , Goldberg , M. , Susi , P. and Herrick , R. F. 2003 . Excessive Exposure to Silica in the US Construction Industry . Ann. Occup. Hyg., , 47 : 111 – 122 .
- Rattanavaraha , W. , Rosen , E. , Zhang , H. F. , Li , Q. F. , Pantong , K. and Kamens , R. M. 2011 . The Reactive Oxidant Potential of Different Types of Aged Atmospheric Particles: An Outdoor Chamber Study . Atmos. Environ., , 45 : 3848 – 3855 .
- Rhoden , C. R. , Wellenius , G. A. , Ghelfi , E. , Lawrence , J. and Gonzalez-Flecha , B. 2005 . PM-Induced Cardiac Oxidative Stress and Dysfunction are Mediated by Autonomic Stimulation . Bba-Gen Subjects, , 1725 : 305 – 313 .
- Ritz , B. , Yu , F. , Fruin , S. , Chapa , G. , Shaw , G. M. and Harris , J. A. 2002 . Ambient Air Pollution and Risk of Birth Defects in Southern California . Am. J. Epidemiol., , 155 : 17 – 25 .
- Sameenoi , Y. , Koehler , K. , Shapiro , J. , Boonsong , K. , Sun , Y. L. Collett , J. 2012 . Microfluidic Electrochemical Sensor for On-Line Monitoring of Aerosol Oxidative Activity . J. Am. Chem. Soc., , 134 : 10562 – 10568 .
- Sameenoi , Y. , Mensack , M. M. , Boonsong , K. , Ewing , R. , Dungchai , W. Chailapakul , O. 2011 . Poly(dimethylsiloxane) Cross-Linked Carbon Paste Electrodes for Microfluidic Electrochemical Sensing . Analyst, , 136 : 3177 – 3184 .
- Sameenoi , Y. , Panymeesamer , P. , Supalakorn , N. , Koehler , K. , Chailapakul , O. Henry , C. S. 2013 . Microfluidic Paper-Based Analytical Device for Aerosol Oxidative Activity . Environ. Sci. Technol., , 47 : 932 – 940 .
- Sauvain , J.-J. , Rossi , M. J. and Riediker , M. 2012 . Comparison of Three Acellular Tests for Assessing the Oxidation Potential of Nanomaterials . Aerosol Sci. Technol., , 47 : 218 – 227 .
- Schafer , F. Q. and Buettner , G. R. 2001 . Redox Environment of the Cell as Viewed Through the Redox State of the Glutathione Disulfide/Glutathione Couple . Free Radical Bio. Med., , 30 : 1191 – 1212 .
- Schlesinger , R. B. 2007 . The Health Impact of Common Inorganic Components of Fine Particulate Matter (PM2.5) in Ambient Air: A Critical Review . Inhal. Toxicol., , 19 : 811 – 832 .
- Sioutas , C. , Delfino , R. J. and Singh , M. 2005 . Exposure Assessment for Atmospheric Ultrafine Particles (UFPs) and Implications in Epidemiologic Research . Environ. Health Perspect., , 113 : 947 – 955 .
- Squadrito , G. L. , Cueto , R. , Dellinger , B. and Pryor , W. A. 2001 . Quinoid Redox Cycling as a Mechanism for Sustained Free Radical Generation by Inhaled Airborne Particulate Matter . Free Radical Bio. Med., , 31 : 1132 – 1138 .
- Stone , P. H. and Godleski , J. J. 1999 . First Steps Toward Understanding the Pathophysiologic Link Between Air Pollution and Cardiac Mortality . Am. Heart. J. , 138 : 804 – 807 .
- Tamagawa , E. , Bai , N. , Morimoto , K. , Gray , C. , Mui , T. Yatera , K. 2008 . Particulate Matter Exposure Induces Persistent Lung Inflammation and Endothelial Dysfunction . Am. J. Physiol.-Lung. C, , 295 : L79 – L85 .
- Timonen , K. L. , Vanninen , E. , De Hartog , J. , Ibald-Mulli , A. , Brunekreef , B. Gold , D. R. 2006 . Effects of Ultrafine and Fine Particulate and Gaseous Air Pollution on Cardiac Autonomic Control in Subjects with Coronary Artery Disease: The ULTRA study . J. Expo. Sci. Env. Epid., , 16 : 332 – 341 .
- Truong , H. , Lomnicki , S. and Dellinger , B. 2010 . Potential for Misidentification of Environmentally Persistent Free Radicals as Molecular Pollutants in Particulate Matter . Environ. Sci. Technol., , 44 : 1933 – 1939 .
- van Eeden , S. F. , Tan , W. C. , Suwa , T. , Mukae , H. , Terashima , T. Fujii , T. 2001 . Cytokines Involved in the Systemic Inflammatory Response Induced by Exposure to Particulate Matter Air Pollutants (PM10) . Am. J. Resp. Crit. Care, , 164 : 826 – 830 .
- Veronesi , B. , Oortgiesen , M. , Carter , J. D. and Devlin , R. B. 1999 . Particulate Matter Initiates Inflammatory Cytokine Release by Activation of Capsaicin and Acid Receptors in a Human Bronchial Epithelial Cell Line . Toxicol. Appl. Pharm., , 154 : 106 – 115 .
- Vidrio , E. , Phuah , C. H. , Dillner , A. M. and Anastasio , C. 2009 . Generation of Hydroxyl Radicals from Ambient Fine Particles in a Surrogate Lung Fluid Solution . Environ. Sci. Technol. , 43 : 922 – 927 .
- Wise , S. and Watters , R. 2009 . Certificate of Analysis: Standard Reference Material 1649b . Urban Dust. , Available online at: https://www-s.nist.gov/srmors/view_detail.cfm?srm=1649b
- Wu , W. D. , Graves , L. M. , Jaspers , I. , Devlin , R. B. , Reed , W. and Samet , J. M. 1999 . Activation of the EGF Receptor Signaling Pathway in Human Airway Epithelial Cells Exposed to Metals . Am. J. Physiol.-Lung. C, , 277 : L924 – L931 .
- Yang , A. , Jedynska , A. , Hellack , B. , Kooter , I. , Hoek , G. Brunekreef , B. 2014 . Measurement of the Oxidative Potential of PM2.5 and Its Constituents: The Effect of Extraction Solvent and Filter Type . Atmos. Environ. , 83 : 35 – 42 .