ABSTRACT
The electronic cigarette (EC) is a new source of indoor airborne particles. To better understand the impacts of secondhand vaping (SHV) emissions on indoor air quality, real-time measurements of particle size distribution, particle number concentration (PNC), fine particulate matter (PM2.5), CO2, CO, and formaldehyde were conducted before, during, and after 10 min EC-use among 13 experienced users in an 80 m3 room. To assess particle transport in the room, multiple sampling locations were set up at 0.8, 1.5, 2.0, and 2.5 m away from the subjects. The arithmetic mean (standard deviation) of background PNC and PM2.5 concentrations in the room were 6.39 × 103 (1.58 × 102) particles/cm3 and 8 (1) μg/m3, respectively. At 0.8 m away from EC users, right after initiation of puffing, the PNC and PM2.5 concentrations can reach a peak of ∼105 particles/cm3 and ∼3 × 103 µg/m3, respectively, and then dropped quickly to background levels within 20 s due to dilution and evaporation. At the 0.8 m sampling location, the mean PNC and PM2.5 concentrations during puffing were 2.48 × 104 (2.14 × 104) particles/cm3 and 188 (433) µg/m3, respectively. In addition, two modes of SHV particles were observed at about 15 and 85 nm. Moreover, concentrations of SHV particles were negatively correlated with the distances to EC users. At the 1.5 m location, PNC and PM2.5 levels were 9.91 × 103 (1.76 × 103) particles/cm3 and 19 (14) µg/m3, respectively. Large variations of mean PNC levels exhaled per puff were observed both within and between EC users. Data presented in this study can be used for SHV particle exposure assessment.
Copyright © 2017 American Association for Aerosol Research
EDITOR:
1. Introduction
The electronic cigarette (EC) is an increasingly popular alternative to the tobacco cigarette (Ayers et al. Citation2011; Dawkins et al. Citation2013; Weaver et al. Citation2016). ECs are usually composed of two parts: a rechargeable battery and a cartridge, which contains a heating coil and e-liquid (i.e., a chemical mixture of propylene glycol and vegetable glycerol as the main components with small amounts of flavor additives and selected amounts of nicotine). Unlike tobacco cigarettes that generate particles by combustion, ECs produce particles mainly from vapor condensation. When ECs are puffed, the electric current provided by the battery can increase the temperature of the heating coil to evaporate the e-liquid into vapor, some of which subsequently condense into particles due to rapid cooling (Zhao et al. Citation2016). ECs are advertised as “healthy replacements” to tobacco cigarettes, which have attracted many former tobacco smokers to switch. In addition, the wide varieties of appealing flavor choices and large advertising investments have attracted a great number of consumers, including adolescents (Center for Disease Control and Prevention Citation2013; Farsalinos et al. Citation2013; Richardson et al. Citation2014). In the United States, the number of high school students using ECs has tripled from 4.5% (660,000 students) in 2013 to 13.4% (2 million students) in 2014. Meanwhile, the number of middle school EC users rose from 1.1% (120,000 students) in 2013 to 3.9% in 2014 (450,000 students) (Center for Disease Control and Prevention Citation2015).
Currently, the high concentrations of particles and detected toxic compounds in EC mainstream aerosols have generated concerns for indoor air quality and human health. Aldehydes (e.g., formaldehyde and acetaldehyde), which might be the product of the thermal dehydration of glycerin or glycols, have been detected in EC aerosols (McAuley et al. Citation2012; Uchiyama et al. Citation2013; Goniewicz et al. Citation2014; Jensen et al. Citation2015; Sleiman et al. Citation2016). Compared with those in mainstream tobacco smoke, higher levels of nickel, chromium, and lead were also found in EC aerosols (Pellegrino et al. Citation2012; Williams et al. Citation2013; Lerner et al. Citation2015; Mikheev et al. Citation2016). Furthermore, studies have reported extremely high particle number concentrations (PNC) ranging from 7.69 × 107 to 8.38 × 109 particles/cm3 in EC mainstream aerosols (Ingebrethsen et al. Citation2012; Schripp et al. Citation2013; Fuoco et al. Citation2014; Mikheev et al. Citation2016; Zhao et al. Citation2016).
Secondhand exposure from EC vaping, also called secondhand vaping (SHV), occurs when EC aerosols are exhaled. SHV aerosols are subject to transformations in the users' respiratory tract systems and in the ambient environments before reaching to the exposed bystanders. There have been limited studies that characterize SHV aerosols. McAuley et al. (Citation2012) found that the PNC of SHV emissions was lower than secondhand smoke (SHS) when they introduced emissions from an EC and a tobacco cigarette into a 0.56 m3 sampling bag. However, they used a puffing machine to generate mainstream EC aerosols, instead of actual SHV particles exhaled by human subjects. Moreover, the characteristics of EC particles, which would coagulate very fast in such a small volume of a sampling bag, cannot accurately represent those in a realistic indoor environment. Schripp et al. (Citation2013) recruited a subject to puff an EC in an 8 m3 chamber and found that the SHV particle size distribution was bimodal (i.e., 30 and 100 nm). Since breathing parameters (e.g., inhalation volume) were diverse and can affect exhaled particles, it is not clear if the results from only one subject were generalizable. Moreover, the volume of the 8 m3 chamber was constrained to study the spatial distribution of SHV particles. Czogala et al. (Citation2014), Schober et al. (Citation2014), and Maloney et al. (Citation2016) recruited more subjects and used larger rooms in real-setting (i.e., 39, 45, and 137 m3). They found that the mean PM2.5 mass concentration of SHV particles can reach a maximum of 273 µg/m3 (Czogala et al. Citation2014), the mean PNC can exceed 4.5 × 104 particles/cm3 (Schober et al. Citation2014), and the maximum PNC can reach 2.21 × 104 particles/cm3 (Maloney et al. Citation2016). Unfortunately, these studies did not present the temporal or spatial profiles of SHV particles.
Given these knowledge gaps, this study aims to investigate the temporal and spatial characteristics of SHV particles in a realistic room. A total of 13 experienced EC users from both genders were recruited. Real-time PNC, PM2.5, particle size distribution, CO, CO2 and formaldehyde levels were measured at different locations before, during, and after 10 min EC-use. To the authors' best knowledge, this is the first study that reports both temporal and spatial profiles of SHV aerosols in an indoor environment.
2. Methods and materials
2.1. Experimental setting and subjects
As part of a larger study on the cardiovascular effects of EC usage, we characterized SHV particles before, during, and after EC use among human subjects. The subjects were recruited using the following inclusion criteria: healthy male and female subjects who regularly use ECs (minimum 1 h/week). A total of 13 experienced EC users (seven females and six males, age: 23–39 years old) were tested. Every subject signed the written Informed Consent Form. The Institutional Review Board at University of California, Los Angeles approved the study protocol. Since the current study focuses on the SHV particle characteristics, only the particle-related methods and materials, results, and discussion are presented below.
The study was conducted from May 2015 to January 2016 in a patient room that was located in UCLA Clinical and Translational Research Center (schematic diagram is shown in Supporting Information Figure S1). The room is 80 m3 (5.8 × 5.3 × 2.6 m) and is furnished with a bed (1.2 m tall), two tables (0.8 m tall), and a water sink (1.2 m tall). There are no windows. Instead, there are two air conditioner outlets on the ceiling to keep the room temperature at 24 ± 2°C. During the experiments, the relative humidity ranged from 39% to 45%, and the mean air exchange rate was 4.1 h−1 based on the tracer gas method (Sherman Citation1990).
2.2. Study protocol
Before the experiments, the subjects became familiar with the EC products and experimental procedures through training under the instructions of a researcher (H.R.M.). A 30 min sampling session comprised of three 10 min intervals: before-puffing, puffing, and after-puffing. Before each sampling session, only one subject and the researcher (H.R.M) entered the room and kept the door closed. The researcher sat next to the bed and gave verbal instructions to the subject throughout the sampling session to maintain the uniform temporal pattern of vaping. During the 10 min puffing, the subject was asked to puff an EC continuously with a 3 s puffing, a 3 s holding, and a ∼24 s pause until the next puff. After the 10 min of puffing, the subject and the researcher remained seated for another 10 min to avoid any particle re-suspension that may be induced by walking (Qian et al. Citation2014).
The ECs used in this study were the rechargeable, ciga-like style from Greensmoke® (Richmond, VA, USA) with tobacco flavoring. Before each experiment, the EC battery was fully charged to avoid power drain. Two kinds of e-liquid cartridges, with nicotine (i.e., 2.4% [mass/volume] nicotine) and without nicotine, were used in this study. Among the 13 experienced EC users, five used ECs without nicotine, four used ECs with nicotine (2.4% nicotine in the e-liquid), and four used both (participated in this study twice). In total, seventeen experiment sessions were conducted. Among those who participated twice, the interval of their two tests was more than 1 day. The choice of nicotine use was not related to the main objective of this study, but was determined by the aforementioned larger study, which also aimed to examine the nicotine effects on EC users.
2.3. Measurements and instrumentation
2.3.1. Measurement of particle number and PM2.5 concentration
Measurement instruments were usually set up at two locations (as shown Figure S1 in the online supplementary information [SI]): 0.8 and 1.5 m away from the subjects. The sampling instruments were located on the right-hand side of the EC users at about 1.2 m above the ground. At each sampling location, a Condensation Particle Counter (CPC 3007, TSI Inc., Shoreview, MN, USA) and a DustTrak Aerosol Monitor (DustTrak 8532 and 8520, TSI Inc.) were used to measure PNC and PM2.5 every second, respectively. To study the spatial profile of SHV particles, these instruments were also used occasionally at two additional locations, 2.0 and 2.5 m away from the subjects.
To ensure that the data from different instrument units were comparable, collocation tests were conducted. The ratio of data from CPC2 to CPC1 (as the standard) was 0.86 ± 0.001 with the R2 of 0.98 (PNC range: ∼103–105 particles/cm3). The ratio of data from DustTrak2 (DustTrak 8520) to DustTrak1 (DustTrak 8532) (as the standard) was 0.61 ± 0.002 with the R2 of 0.95 (PM2.5 range: ∼10 µg/m3 to 100 mg/m3). It should be noted that the DustTrak 8532 is an upgraded model of DustTrak 8520, so we considered DustTrak 8532 as the standard. In addition, before each sampling session, the instruments were zero-checked and collocated for 2 or 3 min for data quality assurance. The aforementioned linear relationships have been applied to correct all the PNC and PM2.5 data throughout this study.
2.3.2. Dust trak calibration
Due to the notable measurement bias of optical PM2.5 monitors (Jenkins et al. Citation2004), the DustTrak 8532 was calibrated by the gravimetric method (U.S. EPA Citation1997). EC mainstream particles were puffed into a 320-L stainless steel chamber that had mixing fans inside. The temperature and relative humidity (RH) during the experiments in the chamber were 24.1 ± 0.8°C and 36.8 ± 1.9%, respectively. The ECs were puffed by a homemade puffing machine with a puff flow rate of 1 L/min and puff duration of 3 s. The same EC products that the subjects used during the SHV study were used in the calibration tests. We controlled puffing times to achieve various PM2.5 concentrations in the chamber. More details of the chamber operation and the puffing machine can be found elsewhere (Zhao et al. Citation2016). During the experiment, the DustTrak and a gravimetric sampler were placed outside the chamber. The particles were drawn into the DustTrak and gravimetric sampler through separate sampling lines, and the ends of the two lines on one side were collocated and placed in the center of the chamber. A personal cascade impactor was used to provide a cut-size of 2.5 μm (Sioutas Cascade Impactor, SKC Inc., Eighty Four, PA, USA) and to collect particles less than 2.5 μm on a 37 mm Teflon membrane filter (SKC Inc.). The sampling flow rate was 9.0 ± 0.14 L/min as designated by the impactor and calibrated by a volumetric flow meter (BGI Incorporated, Waltham, MA, USA). The filters were conditioned for ∼48 h pre- and post-sampling in a temperature (20°C) and RH (50%) controlled chamber. The collected filters were weighed by a mass balance (Mettler Toledo MX5, Columbus, OH, USA). The range of target PM2.5 concentrations in the chamber was wide, from 500 µg/m3 to 105 µg/m3 (DustTrak reading), which covered the peak concentration of each puff that was observed in the field study. The sampling time was pre-estimated so that the collected mass would be more than 0.1 mg on the filter each time. Finally, the collected mass on the filter, sampling time, and pump flow rate were used to determine the mean PM2.5 mass concentration during sampling. In total, 12 experiments were conducted, the data of which are shown in . The calibration ratio (slope) was 0.27 ± 0.004 (R2 = 0.99). We used this calibration ratio to correct all the DustTrak data during EC puffing periods.
2.3.3. Measurement of particle size distribution
A scanning mobility particle sizer (SMPS 3080, TSI Inc.) was used to measure particle size distribution. The sampling flow rate of SMPS was 0.6 L/min and the particle size measurement range was 7–289 nm (100 s up scan, 20 s down scan). The sampling inlet was placed at the 0.8 m sampling location for 12 samples and then at the 1.5 m sampling location for five samples. During the 100 s up scan, subjects typically puffed three times. Thus, a particle size distribution from one SMPS scan typically showed three peaks (as shown in Figure S2 in the SI), which are related to the three puffs and should not be viewed as three modes. We examined every available SMPS spectrum and picked the data points around each identified peak (usually two or three size bins, as the arrows indicated in Figure S2 in the SI). Based on 65 valid SMPS size distribution scans, 198 peaks with a total number of 402 data points were identified. Then, a bimodal log-normal distribution was fitted to these data, which represents the size distribution of SHV particles at the 0.8 m sampling location. The CMD (count median diameter) and GSD (geometric standard deviation) of each mode were also obtained.
In addition, an indoor air quality monitor (Q-Trak 8552 TSI Inc.) was used to measure temperature, RH, CO, and CO2 concentrations in the room every second. A formaldehyde monitor (FM-801, Graywolf Inc., Shelton, CT, USA) with a detection limit of 10 ppb was used to measure the formaldehyde concentration every 30 min.
2.4. Data and statistical analysis
For each subject, the “mean PNC per puff” was defined as the mean PNC level over a 30 s time period (including a 3 s puff and a 27 s puff interval) after subtracting the background PNC, which can be calculated as[1] where Cpuff (particles/cm3) is the “mean PNC over a 30 s time period,” C (particles/cm3) is the particle number concentration, Cb (particles/cm3) is the background PNC in the room, and t (s) is puffing start time. One Way ANOVA on Ranks was used to compare (1) the mean PNCs during puffing at 0.8, 1.5, 2, and 2.5 m away from the EC users (N = 4); (2) the mean PM2.5 concentrations during puffing at 0.8, 1.5, 2, and 2.5 m away from the EC users (N = 4); and (3) mean PNCs per puff from each subject (N = 13). The level of significance was taken as p < 0.05. We used R 3.4.0 to process the One Way ANOVA on Ranks test, Microsoft Excel 2016 (Microsoft, Seattle, WA, USA) to statistically summarize the results of mean PNC and PM2.5 levels (after subtracting the background levels) at all sampling locations (as shown in Table S1 in the SI), and Sigmaplot 12.5 (Systat Software Inc., San Jose, CA, USA) to generate the box plots in Sections 3.2 and 3.3.
3. Results and discussion
3.1. Temporal profile of particle number concentration (PNC) and PM2.5 mass concentration
The temporal variations of the mean PNC at two locations (i.e., 0.8 and 1.5 m away from EC users) are shown in . The background PNC level of 6.39 × 103 (1.58 × 102) particles/cm3 was measured in the first 600 s. As the subject started to use the EC at the 600th second, PNC increased immediately and dramatically to ∼105 particles/cm3 at the 0.8 m location, which was about 15-fold of the background. Immediately following each puff, PNC decreased to a near-background level of 1.07 × 104 (9.14 × 102) particles/cm3 within 20 s, which was only ∼10% of the peak value. PNC increased again with the next puff and continued in the same pattern. During puffing, the mean PNC peak value in was 7.98 × 104 (2.16 × 104) particles/cm3. The mean PNC during the 10 min puffing at the 0.8 m location was 2.48 × 104 (2.14 × 104) particles/cm3, which was more than three-fold of the background. After puffing, PNC continuously decreased in the next 10 min with a mean of 7.94 × 103 (3.83 × 103) particles/cm3, which was ∼15% higher than the background.
Figure 2. The time series of the mean value of (a) PNC and (b) PM2.5 mass concentration at two locations, 0.8 and 1.5 m away from EC users. The number of samples (n) is also provided.
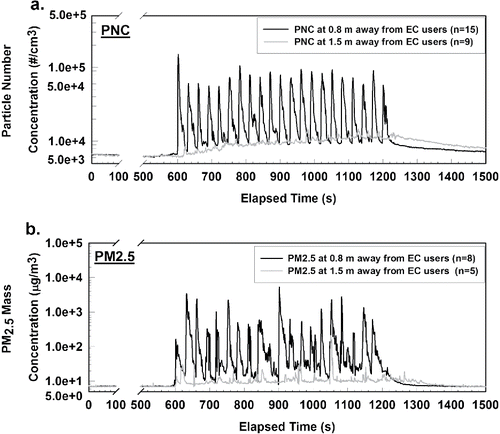
The fast decay of PNC after each puff (in ) is likely related to the dilution and evaporation of EC particles, which are mainly liquid droplets and composed of volatile compounds, such as propylene glycol, water, and flavor additives. It has been identified that significant evaporation can occur to EC constituents under high dilution conditions (Ingebrethsen et al. Citation2012; Mikheev et al. Citation2016). Thus, after the exhaled EC particles are released into the indoor air, they may go through a fast evaporation. In a study by Czogala et al. (Citation2014), evaporation of EC particles was also observed in that the concentration of EC particles decays much faster than tobacco cigarette particles in the same room. Thus, right after each puff, PNC dropped rapidly due to evaporation and resulted in the observed spikes. The evaporation rate is also expected to be higher right after a puff because of the low ambient partial pressure of e-liquid vapor in the air surrounding the droplet (Hinds Citation1999). With continuous puffing and evaporation, the ambient partial pressure would increase gradually, which would then reduce the evaporation rate. This may help explain why the PNCs decayed fast right after each puff and became slower as time went by.
As shown by the gray curve in , at 1.5 m away from EC users, the PNC increased immediately but slightly as the puffing began. The PNC accumulated gradually and reached a peak of 1.35 × 104 particles/cm3 at the end of the puffing period. This suggests that there may be less volatile particles in SHV aerosols that can transport relatively far and lead to a slight increase of PNC at the location 1.5 m away from EC users. During puffing, the mean PNC was 9.91 × 103 (1.76 × 103) particles/cm3, which was less than half of that at 0.8 m. After puffing, for 10 min, the mean PNC was 8.87 × 103 (1.44 × 103) particles/cm3. In addition, although tiny spikes can also be observed at the 1.5 m location, they were only ∼2% of the PNC peak value at 0.8 m.
The variations of mean PM2.5 mass concentrations at both locations are shown in . The mean background PM2.5 level was 8 µg/m3. At the 0.8 m location, PM2.5 followed the same trend as PNC. When puffing started at the 10th min, PM2.5 increased immediately and could reach peaks that were greater than 200 µg/m3, which was about 25 times that of the background. In , peak PM2.5 values during puffing ranged widely from 200 to 4796 µg/m3, and the mean PM2.5 peak value during puffing was 1286 µg/m3. Right after a puff, the PM2.5 dropped to near-background level (mean: 15 µg/m3) and then increased again with the next puff. In the 10 min puffing session, the mean PM2.5 mass concentration was 188 µg/m3, which was about 24-fold of the background. After the 10 min puffing session, the PM2.5 dropped to the background level within 4 min, which was faster than PNC. The spikes on the PM2.5 curve also reflect the quick dynamics of fine particles. At the 1.5 m location, the PM2.5 concentrations also increased immediately with puffing, but not as high as those measured at the 0.8 m location. During puffing, the mean PM2.5 concentration was only 19 µg/m3 and dropped to background level in about 4 min, similar to what was measured at the 0.8 m location.
3.2. Effects of distances to EC users
To further study the spatial profile of SHV particles in the room, additional samples were collected at 2.0 and 2.5 m away from EC users. Because of the small variation, only three samples were collected at each location. presents the mean PNC and PM2.5 levels (after subtracting the background) at all sampling locations. The detailed statistical results were summarized in Supporting Information Table S1. In , the mean PNCs were 1.70 × 104, 3.35 × 103, 2.05 × 103, and 1.26 × 103 particles/cm3 at 0.8, 1.5, 2, and 2.5 m from the EC users, respectively, which indicate that PNC of SHV emissions decreased quickly from the source. When the sampling location moved to 1.5, 2, and 2.5 m from the EC users, the PNC dropped to 20%, 12%, and 7% of what was measured at the 0.8 m location, respectively. Approximately 80% of PNC dropped between 0.8 and 1.5 m, and only 7% of PNC remained beyond 2.5 m. The four groups of PNC data were analyzed by one-way ANOVA on rank, and a significant difference was found (p < 0.05).
Figure 3. The mean (a) PNC and (b) PM2.5 of SHV emissions at increasing distances from the EC users. The dots above or below the boxes represent the outliers, which are beyond 1.5 IQR (Interquartile Range). The upper line above each box represents the maximum data within 1.5 IQR, and the lower line below each box represents the minimum data within 1.5 IQR. The solid line in each box represents the median value, and the dash line in each box represents the mean value.
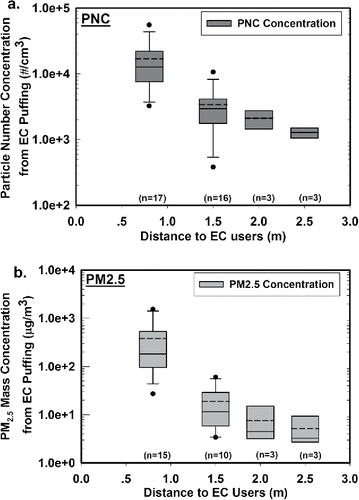
The sampling distance from EC users played a similar role for PM2.5 levels. In , the mean PM2.5 of SHV emissions was 375, 15, 7, and 5 µg/m3 at increasing distances. When the sampling location moved to 1.5, 2, and 2.5 m from the EC user, the PM2.5 dropped to 4%, 2%, and 1% of what was measured at the 0.8 m location, respectively. Approximately 96% of PM2.5 reduction occurred between 0.8 and 1.5 m, which was greater than that of PNC. The four groups of PM2.5 data were analyzed by one-way ANOVA on rank, and a significant difference was found (p < 0.05).
The above findings have two implications: (1) the distances to EC users should be considered in SHV particle exposure assessment, and (2) the data can be used to model the behaviors and transportation of SHV particles in indoor environments (Rostami et al. Citation2016). However, cautions must be given since the well-mixed assumption in the mass balance-based indoor air quality model is no longer valid.
3.3. Individual difference
For each subject, as shown in Equation (Equation1[1] ), the “mean PNC per puff” (Cpuff (particles/cm3)) was defined as the mean PNC over a 30 s time period (including a 3 s puff and a 27 s puff interval) after subtracting the background. We numbered the subjects from 1 to 13 and plotted their mean PNC per puff in the box plots in . We found a large variation between puffs produced by an individual EC user (intra-subject) and across different EC users (inter-subject). As shown in , the mean value of mean PNC per puff produced by each subject ranged from 8.05 × 103 to 4.0 × 104 particles/cm3, which demonstrated a large inter-subject variability. Moreover, the extent of each box shows the intra-subject variability, which was also large, especially for Subjects 4, 5, 6, 9, 11, 12, and 13. We did a one-way ANOVA on rank test to compare the mean PNCs per puff among the 13 subjects and found a significant difference (p < 0.05). As shown in one of our previous studies, the mean PNC per puff was determined by both the puff duration and puff flow rate (Zhao et al. Citation2016). Robinson et al. (Citation2016) reported significant intra-subject and inter-subject variabilities of both the puff duration and puff flow rate by comparing the puff flow rate and puff duration of 21 subjects under natural puffing conditions for a week. In our study, although we instructed each subject to puff exactly at 3 s intervals, the variances of puff flow rates within and across subjects is likely to result in the large variance in mean PNC per puff as observed in .
Figure 4. The mean PNC per puff (particles/cm3) for each subject. The dots above or below the boxes represent the outliers, which are beyond 1.5 IQR (Interquartile Range). The upper line above each box represents the maximum data within 1.5 IQR, and the lower line below each box represents the minimum data within 1.5 IQR. The solid line in each box represents the median value, and the dash line in each box represents the mean value.
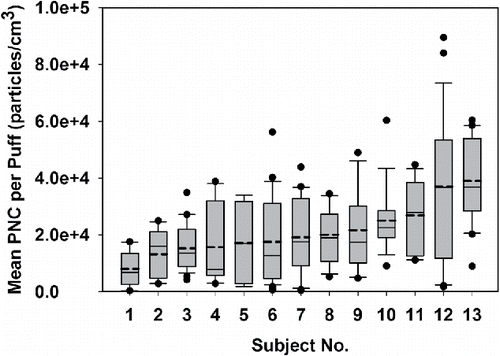
3.4. Particle size distribution
As shown in , at 0.8 m away from EC users, the particle size distribution was bimodal, with one mode located at 15 nm and the other at 85 nm. The GSDs of the two modes are 1.27 and 1.65, respectively. This result was similar to that found by Schripp et al. (Citation2013), who measured SHV particles with a fast mobility particle sizer (FMPS) in an 8 m3 chamber and reported two modes: 30 nm and 100 nm. Mikheev et al. (Citation2016) also confirmed that the EC particles have two modes with the CMD varying from 11 to 25 nm and from 96 to 175 nm. These two modes are likely composed of different compounds (Mikheev et al. Citation2016). The particle size distributions before and after the 10 min puffing period are also shown at the bottom of . They were both unimodal and much lower than those “during puffing.” At the 1.5 m sampling location, as shown in , the particle size distribution was unimodal before, during, and after the 10 min puffing period (data with uncertainties can be found in Figure S3 in the SI). It should be noted that our CMD data of SHV aerosols are likely to be underestimated because when using the SMPS to measure EC particles, the volatile part of the EC particles might evaporate as particles travel from the instrument's inlet to the measurement unit (Wright et al. Citation2016). A faster response instrument would provide more precise measurements of the size distribution for EC particles.
Figure 5. The particle size distributions of SHV aerosols measured at (a) 0.8 m and (b) 1.5 m away from EC users. CMD: count median diameter; GSD: geometric standard deviation.
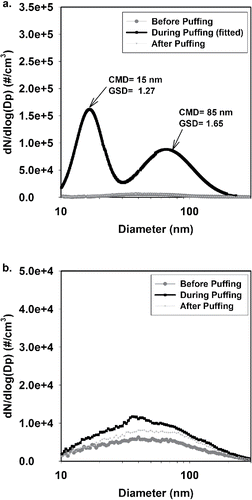
Formaldehyde, CO, and CO2 were also measured in this study. The formaldehyde concentrations during puffing were below the detection limit (10 ppb), which was well below the exposure limits of formaldehyde concentration in non-occupational settings (i.e., World Health Organization: 100 ppb in 30 min, US Department Housing and Urban Development: 300 ppb; State of California Office of Environmental Health Hazard Assessment: 23 ppb) (Center for Disease Control and Prevention Citation2008). The background concentrations of CO and CO2 across all the sampling days were between 0.1 and 2 ppm and between 320 and 525 ppm, respectively. No notable increase of CO or CO2 concentration was found during puffing (see Figure S4 in the SI for details).
4. Conclusions
This article presents the temporal and spatial profiles of SHV aerosols in an indoor environment. PNC, PM2.5, particle size distribution, CO, CO2, and formaldehydeconcentrations were measured before, during, and after 10-min EC-puffing from 13 human subjects. SHV produces a large amount of particles, which evaporate fast. At 0.8 m away from EC users, right after puffing began, the PNC and PM2.5 peak values reached up to ∼105 particles/cm3 and ∼ 3 × 103 µg/m3, respectively. These levels then dropped quickly to background levels within 20 s after each puff. The mean PNC level during the 10-min puffing was 2.48 × 104 (2.14 × 104) particles/cm3, about three-fold of the background PNC level. The mean PM2.5 level was 188 (433) µg/m3, about 24-fold of the background PM2.5 level. It took 10 and 4 min for PNC and PM2.5 to drop to background level after puffing, respectively. SHV particles have a sharp spatial profile in the room. Both PNC and PM2.5 levels were negatively correlated with the distances to EC users. Compared with levels measured at the 0.8 m location, PNC dropped to 20%, 12%, and 7%, and PM2.5 dropped to 4%, 2%, and 1%, at the 1.5 m, 2 m, and 2.5 m locations, respectively. These results can be used for modeling the SHV particle behaviors and transportation in indoor environments. Moreover, large variations were observed for both within and between subjects with respect to mean PNC exhaled per puff. These data can be used to estimate exposure to SHV aerosols in indoor environments.
UAST_1355548_Supplementary_File.zip
Download Zip (355.5 KB)Funding
This work was supported by the Tobacco-Related Disease Research Program (TRDRP) (Contract #23XT-0001) (Y.Z.), TRDRP-XT (Contract #320833) (H.R.M.), American Heart Association, Western States Affiliate, Grant-in-Aid (Contract #15GRNT22930022) (H.R.M.), and the UCLA Clinical and Translational Science Institute (CTSI) (Grant #UL1TR000124) (H.R.M). Tongke Zhao would like to thank the China Scholarship Council (No. 201406010038) for providing her with funding to visit and conduct this research at the University of California, Los Angeles.
References
- Ayers, J. W., Ribisl, K. M., and Brownstein, J. S. (2011). Tracking the Rise in Popularity of Electronic Nicotine Delivery Systems (Electronic Cigarettes) Using Search Query Surveillance. American Journal of Preventive Medicine, 40:448–453.
- Center for Disease Control and Prevention. (2008). Formaldehyde Exposure in Homes: A Reference for State Officials to Use in Decision-Making.
- Center for Disease Control and Prevention. (2015). E-Cigarette Use Triples Among Middle and High School Students in Just One Year. CDC Newsroom Release.
- Center for Disease Control and Prevention. (2013). Notes from the Field: Electronic Cigarette Use Among Middle and High School Students-United States, 2011–2012. MMWR. Morbidity and Mortality Weekly Report, 62:729.
- Czogala, J., Goniewicz, M. L., Fidelus, B., Zielinska-Danch, W., Travers, M. J., and Sobczak, A. (2014). Secondhand Exposure to Vapors from Electronic Cigarettes. Nicotine & Tobacco Research, 16:655–662.
- Dawkins, L., Turner, J., Roberts, A., and Soar, K. (2013). ‘Vaping’ Profiles and Preferences: An Online Survey of Electronic Cigarette Users. Addiction, 108:1115–1125.
- Farsalinos, K. E., Romagna, G., Tsiapras, D., Kyrzopoulos, S., Spyrou, A., and Voudris, V. (2013). Impact of Flavour Variability on Electronic Cigarette use Experience: An Internet Survey. International Journal of Environmental Research and Public Health, 10:7272–7282.
- Fuoco, F., Buonanno, G., Stabile, L., and Vigo, P. (2014). Influential Parameters on Particle Concentration and Size Distribution in the Mainstream of E-Cigarettes. Environmental Pollution, 184:523–529.
- Goniewicz, M. L., Knysak, J., Gawron, M., Kosmider, L., Sobczak, A., Kurek, J., Prokopowicz, A., Jablonska-Czapla, M., Rosik-Dulewska, C., and Havel, C. (2014). Levels of Selected Carcinogens and Toxicants in Vapour from Electronic Cigarettes. Tobacco Control, 23:133–139.
- Hinds, W. C. (1999). Aerosol Technology: Properties, Behavior, and Measurement of Airborne Particles. John Wiley & Sons, New York.
- Ingebrethsen, B. J., Cole, S. K., and Alderman, S. L. (2012). Electronic Cigarette Aerosol Particle Size Distribution Measurements. Inhalation Toxicology, 24:976–984.
- Jenkins, R. A., Ilgner, R. H., Tomkins, B. A., and Peters, D. W. (2004). Development and Application of Protocols for the Determination of Response of Real-Time Particle Monitors to Common Indoor Aerosols. Journal of the Air & Waste Management Association, 54:229–241.
- Jensen, R. P., Luo, W., Pankow, J. F., Strongin, R. M., and Peyton, D. H. (2015). Hidden Formaldehyde in e-Cigarette Aerosols. New England Journal of Medicine, 372:392–394.
- Lerner, C. A., Sundar, I. K., Watson, R. M., Elder, A., Jones, R., Done, D., Kurtzman, R., Ossip, D. J., Robinson, R., and McIntosh, S. (2015). Environmental Health Hazards of E-Cigarettes and their Components: Oxidants and Copper in e-Cigarette Aerosols. Environmental Pollution, 198:100–107.
- Maloney, J. C., Thompson, M. K., Oldham, M. J., Stiff, C. L., Lilly, P. D., Patskan, G. J., Shafer, K. H., and Sarkar, M. A. (2016). Insights from Two Industrial Hygiene Pilot E-Cigarette Passive Vaping Studies. Journal of Occupational and Environmental Hygiene, 13:1.
- McAuley, T. R., Hopke, P., Zhao, J., and Babaian, S. (2012). Comparison of the Effects of e-Cigarette Vapor and Cigarette Smoke on Indoor Air Quality. Inhalation Toxicology, 24:850–857.
- Mikheev, V. B., Brinkman, M. C., Granville, C. A., Gordon, S. M., and Clark, P. I. (2016). Real-Time Measurement of Electronic Cigarette Aerosol Size Distribution and Metals Content Analysis. Nicotine & Tobacco Research Official Journal of the Society for Research on Nicotine & Tobacco, 18:ntw128.
- Pellegrino, R., Tinghino, B., Mangiaracina, G., Marani, A., Vitali, M., Protano, C., Osborn, J., and Cattaruzza, M. (2012). Electronic Cigarettes: An Evaluation of Exposure to Chemicals and Fine Particulate Matter (PM). Annali Di Igiene: Medicina Preventiva E Di Comunita, 24:279–288.
- Qian, J., Peccia, J., and Ferro, A. R. (2014). Walking-Induced Particle Resuspension in Indoor Environments. Atmospheric Environment, 89:464–481.
- Richardson, A., Ganz, O., and Vallone, D. (2014). Tobacco on the Web: Surveillance and Characterisation of Online Tobacco and E-Cigarette Advertising. Tobacco Control, 25(3):246–249.
- Robinson, R. J., Hensel, E. C., Roundtree, K. A., Difrancesco, A. G., Nonnemaker, J. M., and Lee, Y. O. (2016). Week Long Topography Study of Young Adults Using Electronic Cigarettes in Their Natural Environment. Plos One, 11:e0164038.
- Rostami, A. A., Pithawalla, Y. B., Liu, J., Oldham, M. J., Wagner, K. A., Kimberly, F. P., and Sarkar, M. A. (2016). A Well-Mixed Computational Model for Estimating Room Air Levels of Selected Constituents from E-Vapor Product Use. International Journal of Environmental Research & Public Health, 13:828.
- Schober, W., Szendrei, K., Matzen, W., Osiander-Fuchs, H., Heitmann, D., Schettgen, T., Jörres, R. A., and Fromme, H. (2014). Use of Electronic Cigarettes (E-Cigarettes) Impairs Indoor Air Quality and Increases FeNO Levels of E-Cigarette Consumers. International Journal of Hygiene & Environmental Health, 217:628–637.
- Schripp, T., Markewitz, D., Uhde, E., and Salthammer, T. (2013). Does E-Cigarette Consumption Cause Passive Vaping? Indoor Air, 23:25–31.
- Sherman, M. (1990). Tracer-Gas Techniques for Measuring Ventilation in A Single Zone. Building and Environment, 25:365–374.
- Sleiman, M., Logue, J. M., Montesinos, V. N., Russell, M. L., Litter, M. I., Gundel, L. A., and Destaillats, H. (2016). Emissions from Electronic Cigarettes: Key Parameters Affecting the Release of Harmful Chemicals. Environmental Science & Technology, 50:9644–9651.
- Uchiyama, S., Ohta, K., Inaba, Y., and Kunugita, N. (2013). Determination of Carbonyl Compounds Generated from the E-Cigarette Using Coupled Silica Cartridges Impregnated with Hydroquinone and 2, 4-Dinitrophenylhydrazine, Followed by High-Performance Liquid Chromatography. Analytical Sciences, 29:1219–1222.
- U.S. EPA. (1997). Reference Method for The Determination of Fine Particulate Matter as PM2.5 in The Atmosphere. 40 CFR Part 50, Appendix L.
- Weaver, S. R., Majeed, B. A., Pechacek, T. F., Nyman, A. L., Gregory, K. R., and Eriksen, M. P. (2016). Use of Electronic Nicotine Delivery Systems and Other Tobacco Products Among USA Adults, 2014: Results from A National Survey. International Journal of Public Health, 61:177–188.
- Williams, M., Villarreal, A., Bozhilov, K., Lin, S., and Talbot, P. (2013). Metal and Silicate Particles Including Nanoparticles are Present in Electronic Cigarette Cartomizer Fluid and Aerosol. PloS One, 8:e57987.
- Wright, T. P., Chen, S., Sears, S., and Petters, M. D. (2016). Thermodynamic and Kinetic Behavior of Glycerol Aerosol. Aerosol Science and Technology, 50(12):1385–1396.
- Zhao, T., Shu, S., Guo, Q., and Zhu, Y. (2016). Effects of Design Parameters and Puff Topography on Heating Coil Temperature and Mainstream Aerosols in Electronic Cigarettes. Atmospheric Environment, 134:61–69.