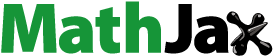
Abstract
Purpose
Conventional techniques (3D-CRT) for craniospinal irradiation (CSI) are still widely used. Modern techniques (IMRT, VMAT, TomoTherapy®, proton pencil beam scanning [PBS]) are applied in a limited number of centers. For a 14-year-old patient, we aimed to compare dose distributions of five CSI techniques applied across Europe and generated according to the participating institute protocols, therefore representing daily practice.
Material and methods
A multicenter (n = 15) dosimetric analysis of five different techniques for CSI (3D-CRT, IMRT, VMAT, TomoTherapy®, PBS; 3 centers per technique) was performed using the same patient data, set of delineations and dose prescription (36.0/1.8 Gy). Different treatment plans were optimized based on the same planning target volume margin. All participating institutes returned their best treatment plan applicable in clinic.
Results
The modern radiotherapy techniques investigated resulted in superior conformity/homogeneity-indices (CI/HI), particularly in the spinal part of the target (CI: 3D-CRT:0.3 vs. modern:0.6; HI: 3D-CRT:0.2 vs. modern:0.1), and demonstrated a decreased dose to the thyroid, heart, esophagus and pancreas. Dose reductions of >10.0 Gy were observed with PBS compared to modern photon techniques for parotid glands, thyroid and pancreas. Following this technique, a wide range in dosimetry among centers using the same technique was observed (e.g., thyroid mean dose: VMAT: 5.6–24.6 Gy; PBS: 0.3–10.1 Gy).
Conclusions
The investigated modern radiotherapy techniques demonstrate superior dosimetric results compared to 3D-CRT. The lowest mean dose for organs at risk is obtained with proton therapy. However, for a large number of organs ranges in mean doses were wide and overlapping between techniques making it difficult to recommend one radiotherapy technique over another.
Introduction
Craniospinal irradiation (CSI) is indicated for medulloblastoma and some rarer tumors with signs of leptomeningeal spread, particularly germ-cell tumors, atypical teratoid rhabdoid tumors and ependymomas [Citation1–8].
The technique most commonly used for treating the craniospinal axis is a combination of two lateral opposed photon beams for the brain, matched to one or more posterior photon fields to treat the spine [Citation9,Citation10]. This approach results in dose inhomogeneity, especially at the beam junction(s), and a significant dose anterior to the spinal target volume. Over the last decade, other techniques for CSI have been investigated in order to decrease the dose to the organs outside the target volume, in particular the thyroid, heart and intestines [Citation11–15]. Intensity-Modulated Radiation Therapy (IMRT), Volumetric Modulated Arc Therapy (VMAT) and TomoTherapy® are highly conformal techniques, which can reduce the dose to the structures anterior to the vertebrae at the expense of a larger volume of low-dose irradiation to the entire body. Due to the steep dose gradient, both electron and proton beam radiation provide substantial sparing of non-target tissues anterior to the spinal target volume compared to photons [Citation16,Citation17].
In clinical practice, the reason for using more conformal techniques is better sparing of healthy tissue. However, the vast majority of late effects reported after CSI in childhood arise from irradiation of the target volume [Citation18–21]. Dose and age influence toxicity outcome and are the justification for dose reduction, altered fractionation regimens, a combination with systemic agents or target volume adaptations [Citation22–26]. Further decrease of late toxicity, e.g., second malignancies outside the target volume, primary hypothyroidism, cardiovascular events, restrictive lung disease and metabolic syndrome might be obtained with modern radiotherapy techniques that lower the dose to the structures anterior to the vertebrae without compromising the target coverage [Citation21,Citation27–32].
The lack of exit dose and high conformity observed with protons are potential reasons for referring patients with a CSI indication to proton therapy centers. However, when referring for proton therapy it is important to balance other factors, such as treatment delay, accessibility, associated financial issues, social disruption of the family and secondary malignancy estimation.
The question we tried to answer in this work was how radiation type and technique influences target dose coverage and OAR dose burden, and how these variables vary when such techniques are executed by different institutions.
In this study, we compare dose distributions of five CSI techniques currently applied across Europe, generated for a single patient and according to the participating institute protocols; therefore, representing daily practice.
To the authors’ knowledge, this is the first time a CSI dose distribution comparison has been performed using the same patient data and with three different institutes plan each of the considered delivery techniques.
Material and methods
A CT scan from a 14-year-old boy, previously irradiated for high-risk medulloblastoma, was selected. Approval for the study was obtained from the University Medical Center Utrecht, Research Ethics Committee.
An individual head-neck support with five-point fixation mask (Civco Medical Solutions, Kalona, IA, USA), vacuum mattress (BlueBagTM Vacuum Cushion, Elekta, Stockholm, Sweden) and a customized knee-feet fixation (MacroMedics BV, Waddinxveen, The Netherlands) were used to scan (slice thickness 3 mm) the patient in a supine position for radiotherapy.
Contouring of the clinical target volume (CTV) and organs at risk (OAR) was performed at one center (Utrecht, The Netherlands). The cranial part of the CTV comprised the entire brain, cranial nerves and meninges. The spinal part of the CTV contained the spinal canal as observed on CT scan including the cerebrospinal fluid extension to the spinal ganglia. The inferior limit of the spinal CTV was defined by a co-registered MRI at the caudal extent of the thecal sac.
The planning target volume (PTV) consisted of an uniform expansion around the CTV of 5 mm for the brain (PTVbrain) and the spinal levels C1-L2 (PTVspine), and of 8 mm for the levels L3-S3 (PTVspine). PTVtotal is defined as the combination of PTVbrain and PTVspine. Outlined OARs included: scalp, left/right lenses, left/right parotid and submandibular glands, thyroid, larynx and proximal esophagus, esophagus, heart, left/right lungs, intestines and stomach, pancreas and left/right kidneys. The total normal tissue volume (TNTV) corresponds to the external contour of the body, imaged on the CT scan, minus PTVtotal.
Treatment planning
The radiotherapy department of the University Medical Center Utrecht, The Netherlands, sent the CT-scan with contours to 14 additional SIOP-E-linked institutes participating in this study. Each center used either 3D-CRT, IMRT, VMAT, TomoTherapy® (in the following Tomotherapy), or PBS for CSI, and three centers per technique were included. Selection of participating centers was based on participation in the radiotherapy working group meeting of the SIOP-E-Brain Tumor Group and the availability to generate a respective treatment plan for CSI. Three institutes per technique were randomly identified.
All participating institutes were asked to return the best treatment plan, applicable in daily practice, for a dose prescription of 36.0 Gy in 20 fractions of 1.8 Gy, and meeting the following criteria: (1) high weighing for PTVtotal coverage (at least 95% of PTVtotal should receive 95% of the prescribed dose), and (2) maximal sparing of the OARs.
An overview of the major characteristics per technique and per center is listed in . An overview of the constraints used by the centers is given in Table S1.
Table 1. Overview of the treatment planning geometry per technique, and per center.
In order to quantify inter-patient dosimetric differences on organs at risk, five patients with indication for CSI, previously irradiated at the radiotherapy department of the University Medical Center Utrecht, were re-planned using VMAT by the same planner for a dose-prescription of 36.0 Gy in 20 fractions of 1.8 Gy.
Plan evaluation
Radiotherapy treatment plans were compared per technique and each specific technique also between centers. Dose-volume histograms were evaluated for the PTVs (PTVtotal, PTVbrain and PTVspine) and the OARs. Conformity index (CI) and homogeneity index (HI) were calculated by using the van‘t Riet formula [Citation33] (CI: range 0–1, with 1 being highly-conformal) and Kataria formula [Citation34] (HI: range 0–1, with 1 being highly heterogeneous):
In the formula: V95% represents the volume receiving at least 95% of the prescribed dose; Dx% the dose received by x% of the volume of the PTV.
For the TNTV, the percentage of volume receiving at least 1.0, 2.0, 5.0, 34.2 and 36.0 Gy was calculated. The median and range (minimum/maximum) of each of the dosimetric parameters were computed for each technique.
Superiority of the different techniques was assessed based on the highest conformity (highest CI) and homogeneity (lowest HI) for the PTV, in combination with the lowest mean dose to the OARs.
For the purpose of this study, a difference between techniques is considered of ‘potential clinical significance’ if a mean dose difference ≥5.0 Gy is observed for the OARs. This threshold is chosen based on a consensus between the participating institutes.
Results
represents the dose distribution in a sagittal plane for a 14-year-old boy, receiving 36.0 Gy by the five different radiotherapy techniques considered in this work.
Figure 1. Craniospinal axis dose distribution with photons (3D-CRT, IMRT, VMAT, Tomotherapy) and protons. Only one out of three generated plans per technique is depicted.

Conformity and homogeneity
The median CI for the PTVtotal of all modern radiotherapy techniques was superior compared to 3D-CRT, and this was attributable to the spinal part of the target volume (). The median HI for PTVtotal was similar for all techniques when considering the range of data per technique; however, better median HI values for PTVspine were observed with modern radiotherapy techniques ().
Table 2. Dosimetric parameters for PTVs and total normal tissue volume per technique.
In particular, for the 3D-CRT technique, hot spots within the PTVspine (V107%: 10.6–27.1%) and absolute doses above 40.0 Gy (111%) were observed ().
The largest variation between centers using the same technique for the CI of the PTVbrain was found for IMRT (0.8–1.0) and PBS (0.7–0.9). For the CI of the PTVspine, largest variation was observed for VMAT (0.6–0.8), Tomotherapy (0.5–0.7) and PBS (0.5–0.7). PBS dose distributions showed the widest range in D2% (PTVbrain: 36.4–40.0 Gy; PTVspine: 36.4–39.6) while VMAT dose distributions in D98% (PTVbrain: 33.7–35.5 Gy; PTVspine: 33.7–35.2 Gy) ( and ).
Normal tissue sparing
Compared with 3D-CRT, a decrease in the mean dose to the thyroid by more than 10.0 Gy (28.5 Gy vs. 15.1* Gy) (* represents average of the Dmean median value of the three modern photon techniques). was observed for all modern photon radiotherapy techniques, while a decrease between 5.0 and 10.0 Gy for the mean dose of both parotid glands (20.5 Gy vs. 14.9* Gy), heart (13.4 Gy vs. 8.1* Gy), esophagus (29.9 Gy vs. 20.7* Gy) and pancreas (17.1 Gy vs. 11.5* Gy) was seen (, ).
Figure 3. Median Dmean (Gy) for the organs at risk surrounding the brain (A) and the spine (B). Error bars show the range (min, max) per technique.
[Tomo: Tomotherapy; PBS: proton pencil beam scanning].
![Figure 3. Median Dmean (Gy) for the organs at risk surrounding the brain (A) and the spine (B). Error bars show the range (min, max) per technique.[Tomo: Tomotherapy; PBS: proton pencil beam scanning].](/cms/asset/a4d6e807-49ed-4fa2-b42b-7bcb355931f0/ionc_a_1465588_f0003_b.jpg)
Table 3. Dmean (Gy) for organs at risk with individual techniques.Table Footnote*
With respect to modern photon techniques, PBS further reduced the mean dose to the OARs by more than 10.0 Gy for the average of both parotid glands (14.9* Gy vs. 4.0 Gy), thyroid (15.1* Gy vs. 0.8 Gy), esophagus (20.7* Gy vs. 2.3 Gy) and pancreas (11.5* Gy vs. 0.0 Gy) while mean dose benefits between 5.0 to 10.0 Gy were observed for the lenses (9.2* Gy vs. 1.8 Gy), submandibular glands (7.9* Gy vs. 1.4 Gy), larynx and proximal esophagus (11.1* Gy vs. 2.3 Gy), heart (8.1* Gy vs. 0.0 Gy), lungs (8.3* Gy vs. 2.2 Gy) and intestines (9.6* Gy vs. 0.4 Gy) (, ).
When comparing one specific radiotherapy technique among the three participating centers, a wide range in mean doses delivered to the OARs was found (). Ranges of >10.0 Gy were observed for the lenses (Tomotherapy), thyroid (VMAT, Tomotherapy), larynx + proximal esophagus (3D-CRT, VMAT, Tomotherapy, PBS) and esophagus (VMAT, Tomotherapy). Differences larger than 10 Gy for D1cc between centers applying the same technique were even more frequent (). Dmean ranges between 5.0 and 10.0 Gy were seen for the lenses (3D-CRT, VMAT, PBS), parotid and submandibular glands (3D-CRT, VMAT, PBS), thyroid (IMRT, PBS), heart (VMAT), intestines-stomach, pancreas and esophagus (VMAT, Tomotherapy), and kidneys (PBS). The range in mean doses for OARs of the spine was the narrowest for 3D-CRT.
Table 4. D1cc (Gy) for organs at risk with individual techniques.Table Footnote*
For all photon techniques, 3D-CRT provided the smallest V1Gy, V2Gy and V5Gy of the TNTV but the highest V34.2Gy and V36Gy. Overlap in TNTV dose was observed for the three modern photon techniques. The lowest TNTV dose was observed with PBS ().
The largest inter-patient difference (maximum minus minimum value) found in Dmean for all OARs, considered in the manuscript, is 3 Gy (data not shown).
Discussion
This multicenter dosimetric comparison of five different radiotherapy techniques (3D-CRT, IMRT, VMAT, Tomotherapy and PBS) currently applied for CSI demonstrates improved dose conformity and homogeneity of the target volume with all modern radiotherapy techniques compared with 3D-CRT, as well as a reduction in mean dose of >5.0 Gy to organs such as the thyroid, heart, esophagus and pancreas. Compared to IMRT, VMAT and Tomotherapy, an additional decrease in mean dose (>5.0 Gy) is found with PBS for lenses, parotid- and submandibular glands, larynx, thyroid, lungs, heart, intestines, stomach and pancreas. However, caution is needed in the interpretation of these results since ranges in mean dose for a number of OARs are wide per technique and also overlapping between different techniques. For example, the mean thyroid dose can range between 5.6 and 24.6 Gy with VMAT and between 0.3 and 10.1 Gy with PBS, depending on the treatment center.
In the literature, several reports demonstrate improved CI and HI for the PTV and field-junctions by the use of modern radiotherapy techniques compared with 3D-CRT [Citation11,Citation13,Citation17,Citation35,Citation36]. However, it should be mentioned that knowledge on the uncertainties related to possible motion of the target and correct target volume delineation are pre-requisites for highly-conformal techniques. The latter becomes relevant at the meningeal surfaces and cerebrospinal fluid in the dural reflections of the cranial nerves [Citation37,Citation38].
In clinical practice, the reason for using more conformal techniques is better sparing of healthy tissue outside the planning target volume. However, nearly all published data on late toxicity after CSI concern neuro-cognitive decline, endocrinopathies or growth retardation, in fact problems inherent to the treatment of the target volume [Citation18–21]. In contrast, fewer results have been published on late toxicity outside the craniospinal target volume despite the use of the conventional 3D-CRT for decades [Citation27–32]. As the introduction of modern radiotherapy techniques is of more recent date, it is still too early to be able to demonstrate a clinical benefit due to better sparing of the OARs surrounding the craniospinal PTV. Nevertheless, for the thyroid, heart, lung and pancreas, it may be relevant to improve organ sparing even at relatively low dose levels [Citation21,Citation29–32].
Techniques like IMRT, VMAT and Tomotherapy have the potential to decrease the dose to the thyroid, heart, esophagus and pancreas compared with 3D-CRT at the cost of a higher integral dose and therefore a higher potential risk of second malignancies induction. For this reason, a higher TNTV dose with modern photon techniques is often used as the argument for 3D-CRT continuation. Proton beam therapy is therefore very attractive, as it offers both high conformity and reduction of integral dose. In the literature, several papers report on the estimated risk for secondary malignancies based on empirical models [e.g., Citation39]. However, the authors believe that this risk estimation should be based on clinical data. Unfortunately, very little clinical information on dose dependency for second malignancy induction is available. With a median follow-up of 10 years, two reports on second malignancies after 3D-CRT have suggested tumor induction mainly within or adjacent to the PTV [Citation27,Citation28]. Therefore, it is uncertain whether a significant increase in second malignancies will be observed due to low dose irradiation to structures anterior to the vertebrae with modern photon techniques. However, although studies did not show that the unintended dose outside the target volume causes clinically significant side effects including secondary cancer, attempts should be made to keep dose to the OARs as low as possible. The same is true when administering protons by maximally limiting the scattered contribution from secondary neutrons, i.e., by preferably using PBS technology rather than passive scattered beams [Citation40]. Additional reasons to refer patients for proton therapy are further dosimetric reductions in mean dose to the organs at risk compared to modern photon techniques. However, it might be questioned whether any clinical benefit will be observed if the doses received by the organs at risk remain far below the expected normal tissue tolerances [Citation21,Citation31,Citation41,Citation42]. Although the dosimetric outcome of this work is in favor of proton therapy and to a lesser extent of modern photon techniques, significant range in mean doses (up to 20 Gy) to the OARs are found between centers using a similar technique. This inter-center variation in mean doses to the OARs is larger than the differences in OARs doses reported by other published studies comparing irradiation techniques [Citation12,Citation14,Citation35,Citation36]. On the one hand, the large dose range points towards an effect of mastering a technique to a different extent, as already observed for VMAT dose distributions by Fogliata et al. [Citation43]. On the other hand, these differences can be attributed to the choice of the optimization criteria made by the centers, prioritizing one objective over another (Table S1). For this planning study, no fixed list of constraints for the OARs was provided to the participants in order to reflect daily practice in different centers using similar techniques. This means that in absence of an international guideline on dose-constraints for OARs related to CSI, a significant dose-range will persist between centers using similar techniques. However, this observation also impacts the potential benefit of one technique compared to another. Knowledge-based planning systems could help reducing the differences in OAR sparing between institutions and techniques [Citation44,Citation45].
As no consensus on dose constraints to vertebral bodies does exist at present time, an adolescent patient was chosen for this study to avoid discussions related to growth problems between centers. Including the vertebrae in the target volume will increase the dose to the structures antero-lateral of the vertebral bodies to some extent. However, it is not expected that the observations/conclusions from this study will alter by additional dose steering on the vertebrae. In addition, selecting an adolescent patient with a larger spinal target volume is technically more challenging.
Although we are aware of the fact that this work is based on the analysis of one patient only, we do not expect that expanding the number of patients will change our findings given the fact that the CSA target volume is quite consistent in between patients, and in relation to the surrounding structures [Citation46]. The widest range of OARs mean doses for five different patients planned by VMAT at our department was 3 Gy. The latter value is smaller than the variation observed for some OARs in between centers using the same technique or in between techniques. This observation supports the methodology of the study to focus on one patient for assessing inter-center variation as it reflects the daily reality for one patient.
The variation in dosimetry could be reduced if the treatment planning exercise would have been repeated using the same constraints for all centers, as already demonstrated by Verbakel et al. [Citation47], However, this re-optimization of the treatment planning technique does not reflect current situations across different centers and techniques.
For comparison purposes the same PTV margin was used for all techniques. We acknowledge that this uncertainty margin is inherent to a technique, equipment and institutional protocols (e.g., patient immobilization methods, patient setup error correction protocols) [Citation48]. Locally adopted PTV margins will have a potential impact on OARs dose in proximity of the target volume. However, it is expected that the found dosimetric range per institution and per technique will persist. Furthermore, the effect of patient (re)positioning uncertainties on the dose distribution has not been taken into account in this analysis. In fact, one technique might be more robust than another resulting in smaller detrimental effects on the ideal static dose distribution calculated by the treatment planning system [Citation49–51]. Comparing the robustness of the different techniques is part of a future work. Finally, this is an in-silico treatment planning study and it has been demonstrated that a robust in-silico planning study may overestimate the potential dosimetric benefits of one technique over another [Citation52,Citation53].
Conclusion
Compared with 3D-CRT, modern radiotherapy techniques demonstrate a superior dose distribution often at the cost of a higher integral dose. With protons, a further dosimetric reduction is observed for the OARs and integral body dose. Nevertheless, a wide range of doses to the OARs is found even between centers using similar techniques. In addition, an international guideline with dose constraints for CSI is essential to ensure comparable outcome between different centers.
Enrica_Seravalli_et_al._Supplementary_files.zip
Download Zip (1,016.6 KB)Disclosure statement
No potential conflict of interest was reported by the author(s).
References
- Gajjar A, Chintagumpala M, Ashley D, et al. Risk-adapted craniospinal radiotherapy followed by high-dose chemotherapy and stem-cell rescue in children with newly diagnosed medulloblastoma (St Jude Medulloblastoma-96): long-term results from a prospective, multicentre trial. Lancet Oncol. 2006;7:813–820.
- Packer R, Gajjar A, Vezina G, et al. Phase III study of craniospinal radiation therapy followed by adjuvant chemotherapy for newly diagnosed average-risk medulloblastoma. JCO. 2006;24:4202–4208.
- Lannering B, Rutkowski S, Doz F, et al. Hyperfractionated versus conventional radiotherapy followed by chemotherapy in standard-risk medulloblastoma: results from the randomized multicenter HIT-SIOP PNET 4 trial. JCO. 2012;30:3187–3193.
- Pizer BL, Weston CL, Robinson KJ, et al. Analysis of patients with supratentorial primitive neuro-ectodermal tumours entered into the SIOP/UKCCSG PNET 3 study. Eur J Cancer. 2006;42:1120–1128.
- Calaminus G, Bamberg M, Jurgens H, et al. Impact of surgery, chemotherapy and irradiation on long term outcome of intracranial malignant non-germinomatous germ cell tumors: results of the German Cooperative trial MAKEI 89. Klin Padiatr. 2004;216:141–149.
- Chi SN, Zimmerman MA, Yao X, et al. Intensive multimodality treatment for children with newly diagnosed CNS atypical teratoid rhabdoid tumor. JCO. 2009;27:385–389.
- Tekautz TM, Fuller CE, Blaney S, et al. Atypical teratoid/rhabdoid tumors (ATRT): improved survival in children 3 years of age and older with radiation therapy and high-dose alkylator-based chemotherapy. J Clin Oncol. 2005;23:1491–1499.
- Merchant TE, Boop FA, Kun LE, et al. A retrospective study of surgery and reirradiation for recurrent ependymoma. Int J Radiat Oncol Biol Phys. 2008;71:87–97.
- Parker WA, Freeman CR. A simple technique for craniospinal radiotherapy in the supine position. Radiother Oncol. 2006;78:217–222.
- Tatcher M, Glicksman A. Field matching considerations in craniospinal irradiation. Int J Radiat Oncol Biol Phys. 1989;17:865–869.
- Parker W, Filion E, Roberge D, et al. Intensity-modulated radiotherapy for craniospinal irradiation: target volume considerations, dose constraints, and competing risks. Int J Radiat Oncol Biol Phys. 2007;69:251–257.
- Pai Panandiker A, Ning H, Likhacheva A, et al. Craniospinal irradiation with spinal IMRT to improve target homogeneity. Int J Radiat Oncol Biol Phys. 2007;68:1402–1409.
- Kusters JM, Louwe RJ, van Kollenburg PG, et al. Optimal normal tissue sparing in craniospinal axis irradiation using IMRT with daily intrafractionally modulated junction. Int J Radiat Oncol Biol Phys. 2011;81:1405–1414.
- Lee YK, Brooks CJ, Bedford JL, et al. Development and evaluation of multiple isocentric volumetric modulated arc therapy technique for craniospinal axis radiotherapy planning. Int J Radiat Oncol Biol Phys. 2012;82:1006–1012.
- Lopez Guerra JL, Marrone I, Jaen J, et al. Outcome and toxicity using helical tomotherapy for craniospinal irradiation in pediatric medulloblastoma. Clin Transl Oncol. 2014;16:96–101.
- Chang EL, Allen P, Wu C, et al. Acute toxicity and treatment interruption related to electron and photon craniospinal irradiation in pediatric patients treated at the University of Texas M.D. Anderson Cancer Center. Int J Radiat Biol Phys. 2002;52:1008–1016.
- St Clair WH, Adams JA, Bues M, et al. Advantage of protons compared to conventional X-ray or IMRT in the treatment of a pediatric patient with medulloblastoma. Int J Radiat Oncol Biol Phys. 2004;58:727–734.
- Ris MD, Packer R, Goldwein J, et al. Intellectual outcome after reduced-dose radiation therapy plus adjuvant chemotherapy for medulloblastoma: a Children’s Cancer Group Study. JCO. 2001;19:3470–3476.
- Camara-Costa H, Resch A, Kieffer V, et al. Neuropsychological outcome of children treated for standard-risk medulloblastoma in the PNET-4 European randomized controlled trial of hyperfractionated versus standard radiation therapy and maintenance chemotherapy. Int J Radiat Oncol Biol Phys. 2015;92:978–985.
- Yock TI, Yeap BY, Ebb DH, et al. Long-term toxic effects of proton radiotherapy for paediatric medulloblastoma: a phase 2 single-arm study. Lancet Oncol. 2016;17:287–298.
- Laughton SJ, Merchant TE, Sklar CA, et al. Endocrine outcomes for children with embryonal brain tumors after risk-adapted craniospinal and conformal primary-site irradiation and high-dose chemotherapy with stem-cell rescue on the SJMB-96 trial. JCO. 2008;25:1112–1118.
- Thomas PR, Deutsch M, Kepner JL, et al. Low-stage medulloblastoma: final analysis of trial comparing standard-dose with reduced-dose neuraxis irradiation. JCO. 2002;18:3004–3011.
- Packer RJ, Goldwein J, Nicholson HS, et al. Treatment of children with medulloblastoma with reduced-dose craniospinal radiation therapy and adjuvant chemotherapy: a Children's Cancer Group Study. JCO. 1999;17:2127–2136.
- Carrie C, Muracciole X, Gomez F, et al. Conformal radiotherapy, reduced boost volume, hyperfractionated radiotherapy, and online quality control in standard-risk medulloblastoma without chemotherapy: results of the French M-SFOP 98 protocol. Int J Radiat Oncol Biol Phys. 2005;63:711–716.
- Vanuytsel L, Brada M. The role of prophylactic spinal irradiation in localized intracranial ependymoma. Int J Radiat Oncol Biol Phys. 1991;21:825–830.
- Rogers SJ, Mosleh-Shirazi MA, Saran FH. Radiotherapy of localised intracranial germinoma: time to sever historical ties? Lancet Oncol. 2005;6:509–519.
- Packer RJ, Zhou T, Holmes E, et al. Survival and secondary tumors in children with medulloblastoma receiving radiotherapy and adjuvant chemotherapy: results of Children’s Oncology Group trial A9961. Neuro Oncol. 2013;15:97–103.
- von Hoff K, Hinkes B, Gerber NU, et al. Long-term outcome and clinical prognostic factors in children with medulloblastoma treated in the prospective randomised multicentre trial HIT91. Eur J Cancer. 2009;45:1209–1217.
- Jakacki RI, Goldwein JW, Larsen RL, et al. Cardiac dysfunction following spinal irradiation during childhood. J Clin Oncol. 1993;11:1033–1038.
- Guldner L, Haddy N, Pien F, et al. Radiation dose and long term risk of cardiac pathology following radiotherapy and anthracyclin for a childhood cancer. Radiother Oncol. 2006; 81:47–56.
- Jakacki RI, Schramm CM, Donahue BR, et al. Restrictive lung disease following treatment for malignant brain tumors: a potential late effect of craniospinal irradiation. J Clin Oncol. 1995;13:1478–1485.
- Nottage KA, Ness KK, Li C, et al. Metabolic syndrome and cardiovascular risk among long-term survivors of acute lymphoblastic leukaemia-from the St. Jude lifetime cohort. Br J Haematol. 2014;165:364–374.
- van't Riet A, Mak AC, Moerland MA, et al. A conformation number to quantify the degree of conformality in brachytherapy and external beam irradiation: application to the prostate. Int J Radiat Oncol Biol Phys. 1997;37:731–736.
- Kataria T, Sharma K, Subramani V, et al. Homogeneity Index: an objective tool for assessment of conformal radiation treatments. J Med Phys. 2012;37:207–213.
- Studenski MT, Shen X, Yu Y, et al. Intensity-modulated radiation therapy and volumetric-modulated arc therapy for adult craniospinal irradiation – a comparison with traditional techniques. Med Dosim. 2013;38:48–54.
- Yoon M, Shin DH, Kim J, et al. Craniospinal irradiation techniques: a dosimetric comparison of proton beams with standard and advanced photon radiotherapy. Int J Radiat Oncol Biol Phys. 2011;81:637–646.
- Noble DJ, Ajithkumar T, Lambert J, et al. Highly conformal craniospinal radiotherapy techniques can underdose the cranial clinical target volume if leptomeningeal extension through skull base exit foramina is not contoured. Clin Oncol (R Coll Radiol). 2017;29:439–447.
- Carrie C, Hoffstetter S, Gomez F, et al. Impact of targeting deviations on outcome in medulloblastoma: study of the French Society of Pediatric Oncology (SFOP). Int J Radiat Oncol Biol Phys. 1999;45:435–439.
- Ho ESQ, Barrett SA, Mullaney LM. A review of dosimetric and toxicity modeling of proton versus photon craniospinal irradiation for pediatrics medulloblastoma. Acta Oncol. 2017; 56:1031–1042.
- Taddei PJ, Mahajan A, Mirkovic D, et al. Predicted risks of second malignant neoplasm incidence and mortality due to secondary neutrons in a girl and boy receiving proton craniospinal irradiation. Phys Med Biol. 2010;55:7067–7080.
- Wolden SL. Protons for craniospinal radiation: are clinical data important? Int J Radiat Oncol Biol Phys. 2013;78:231–232.
- Brodin NP, Munck Af Rosenschold P, Aznar MC, et al. Radiobiological risk estimates of adverse events and secondary cancer for proton and photon radiation therapy of pediatric medulloblastoma. Acta Oncol. 2011;50:806–816.
- Fogliata A, Bergström S, Cafaro I. Cranio-spinal irradiation with volumetric modulated arc therapy: a multi-institutional treatment experience. Radiother. Oncol. 2011; 99:79–85.
- Good D, Lo J, Lee WR, et al. A knowledge-based approach to improving and homogenizing intensity modulated radiation therapy planning quality among treatment centers: an example application to prostate cancer planning. Int J Radiat Oncol Biol Phys. 2013; 87:176–181.
- Tol JP, Delaney AR, Dahele M, et al. Evaluation of a knowledge-based planning solution for head and neck cancer. Int J Radiat Oncol Biol Phys. 2015;91:612–620.
- Bandurska-Luque A, Piotrowski T, Skrobała A, et al. Prospective study on dosimetric comparison of helical tomotherapy and 3DCRT for craniospinal irradiation – a single institution experience. Rep Pract Oncol Radiother. 2015;20:145–152.
- Verbakel WFAR, Doornaert PA, Raaijmakers CP, et al. National planning comparison results in improved plan quality for head and neck radiotherapy. Radiother Oncol. 2018, submitted.
- ICRU report 83, Prescribing, Recording, and Reporting Intensity-Modulated Photon-Beam Therapy (IMRT). J ICRU. 2010.
- Myers P, Stathakis S, Mavroidis P, et al. Evaluation of localization errors for craniospinal axis irradiation delivery using volume modulated arc therapy and proposal of a technique to minimize such errors. Radiother Oncol. 2013;108:107–113.
- Lin H, Ding X, Kirk M, et al. Supine craniospinal irradiation using a proton pencil beam scanning technique without match line changes for field junctions. Int J Radiat Oncol Biol Phys. 2014; 90:71–78.
- Farace P, Bizzocchi N, Righetto R, et al. Supine craniospinal irradiation in pediatric patients by proton pencil beam scanning. Radiother Oncol. 2017;123:112–118.
- Urie MM, Goitein M, Doppke K, et al. The role of uncertainty analysis in treatment planning. Int J Radiat Oncol Biol Phys. 1991;21:91–107.
- Kraan AC, van de Water S, Teguh DN, et al. Dose uncertainties in IMPT for oropharyngeal cancer in the presence of anatomical, range, and setup errors. Int J Radiat Oncol Biol Phys. 2013;87:888–896.