Abstract
Background and purpose: Patients with low-grade glioma (LGG) have a prolonged survival expectancy due to better discriminative tumor classification and multimodal treatment. Consequently, long-term treatment toxicity gains importance. Contemporary radiotherapy techniques such as intensity-modulated radiotherapy (IMRT), volumetric modulated arc therapy (VMAT), tomotherapy (TOMO) and intensity-modulated proton therapy (IMPT) enable high-dose irradiation of the target but they differ regarding delivered dose to organs at risk (OARs). The aim of this comparative in silico study was to determine these dosimetric differences in delivered doses.
Material and methods: Imaging datasets of 25 LGG patients having undergone postoperative radiotherapy were included. For each of these patients, in silico treatment plans to a total dose of 50.4 Gy to the target volume were generated for the four treatment modalities investigated (i.e., IMRT, VMAT, TOMO, IMPT). Resulting treatment plans were analyzed regarding dose to target and surrounding OARs comparing IMRT, TOMO and IMPT to VMAT.
Results: In total, 100 treatment plans (four per patient) were analyzed. Compared to VMAT, the IMPT mean dose (Dmean) for nine out of 10 (90%) OARs was statistically significantly (p < .02) reduced, for TOMO this was true in 3/10 (30%) patients and for 1/10 (10%) patients for IMRT. IMPT was the prime modality reducing dose to the OARs followed by TOMO.
Discussion: The low dose volume to the majority of OARs was significantly reduced when using IMPT compared to VMAT. Whether this will lead to a significant reduction in neurocognitive decline and improved quality of life is to be determined in carefully designed future clinical trials.
Introduction
Gliomas are the most common tumors of the central nervous system (CNS), with an annual incidence of 5.4 per 100,000 in Europe [Citation1]. Low-grade gliomas (LGG), classified as World Health Organization (WHO) grade 2, typically occur in younger adults (2nd–5th decade) and are diffusely infiltrative tumors. Most LGG show a protracted disease course; however, significant differences in survival rates have been identified. Consecutively, molecular parameters, such as 1p19q co-deletions, IDH mutation, TERT promotor mutations, and EGFR-amplifications and -mutations have found their way into the novel WHO classification of CNS tumors, revised in 2016 [Citation2–4]. This integrated diagnosis of gliomas is now leading the neuro-oncology field toward multimodal treatment schedules capable of increasing overall survival of diffuse gliomas with an aggressive clinical behavior [Citation5,Citation6]. Multimodal treatment schedules include neurosurgical resection if feasible and adjuvant treatment with radiation therapy as well as chemotherapy [Citation6]. With increasing survival of LGG patients, therapy induced toxicity, such as neurocognitive decline caused by radiotherapy, which potentially decreases the quality of life of these patients, should be minimized [Citation7].
There has been an impressive progress in recent photon-based radiotherapy techniques, moving from 3D conformal radiotherapy (3D-CRT) to intensity-modulated radiotherapy (IMRT), volumetric modulated arc therapy (VMAT) and tomotherapy (TOMO), delivering high radiation dose to the target and low doses to surrounding organs at risk (OARs) [Citation8]. Examples of this progress are the RTOG 0933 phase 2 clinical study on conformal hippocampus sparing whole brain radiotherapy showing a preservation of memory and quality of life, and the ongoing trial on hippocampus-sparing prophylactic cranial irradiation using VMAT, IMRT or TOMO (NCT01780675) [Citation9].
As a result of their physical characteristics, protons deposit a low entry dose followed by maximum dose delivery the Bragg peak, and zero dose after the dose fall-off at the distal edge of the Bragg peak, resulting in a superior sparing surrounding OARs compared to photons. Conversely, the tumor control probability may be increased at maintained OAR dose or by exploiting the somewhat higher relative biological effectiveness (RBE) [Citation10–13]. Consequently, physicians, patients and health insurance companies are interested in the role of proton therapy (PT), e.g., regarding its possible reduction of toxicity in LGG and head-and-neck cancer patients, among others.
To assess the potential dosimetric gains of intensity-modulated proton therapy (IMPT) for individual LGG patients, we conducted an international multicenter in silico treatment planning study within the Radiation Oncology Collaborative Comparison (ROCOCO) group in a cohort of 25 LGG patients retrospectively retrieved from two Dutch radiotherapy departments [Citation14–16].
Material and methods
Study population
We retrospectively retrieved radiation treatment plans and underlying imaging data, i.e., computed tomography (CT) and magnetic resonance imaging (MRI), of 25 WHO grade 2 LGG patients who had undergone radiation treatment (VMAT or IMRT) at the Radboud University Medical Centre (Radboudumc), Nijmegen (The Netherlands), or at the Department of Radiation Oncology of Maastricht University Medical Center (MAASTRO clinic), Maastricht (The Netherlands). These patients had previously undergone a gross total resection or biopsy depending on the localization of the tumor. No prior systemic therapy had been administered. This ROCOCO in silico trial was approved by the MAASTRO clinic institutional review board and was registered on clinicaltrial.gov ID: NCT NCT02607397. Data are available on www.cancerdata.org [Citation17].
Target volume and OAR definition
An individual head support and thermoplastic mask was used in all patients. The gross tumor volume (GTV) was delineated as the resection cavity, encompassing any residual/recurrent macroscopic tumor on the planning-CT (2 or 3 mm slices) fused with the (pre- and post-surgical) MRI (T1-weighted with contrast agent (CA) and T2-weighted/FLAIR images) using image registration of Eclipse™ (v11.0 Varian Medical Systems, Palo Alto, CA). The clinical target volume (CTV) was defined by the treating radiation oncologist as the GTV with a 1 cm margin, corrected for anatomical boundaries, according to the local guidelines at Radboudumc and MAASTRO clinic. For this in silico study, the CTV was distributed to the participating centers. Taking into account different photon techniques and the individual institution’s margin recipes, the CTV was expanded to the planning target volume (PTV): linear accelerator (LINAC)-based VMAT and IMRT utilized 2 mm accounting for setup errors, and helical tomotherapy-based IMRT used a 3 mm margin based on the individual institution’s clinically derived margins. Intensity-modulated proton therapy used a setup uncertainty of 2 mm and a range uncertainty of 3.5%. For all 25 patients included in this in silico study, the OARs were outlined by one dosimetrist (M.G.) and supervised by a radiation oncologist (D.E.) according to the atlas by Eekers et al. [Citation18] (see for the list of OARs). A double-sided OAR was termed ‘contralateral’ when located at the contralateral hemisphere and not included in the CTV. Whenever the tumor was centrally located or both double-sided OARs were located within the CTV, this OAR was named ‘bilateral’. Dental fillings and associated artifacts were delineated and the density was overridden to that of teeth or soft tissue, respectively. Dental fillings within the treatment beam were an exclusion criterion for IMPT. There was no correction for the use of intravenous contrast during the planning-CT.
Table 1. Tolerance dose and planning priority per organ at risk in Gy (RBE).
Dose prescription
For simplicity reasons, all doses are reported in Gy equivalents (GyE), taking into account the RBE of 1 for photons and 1.1 for protons. The prescribed dose to the target was 50.4 Gy in 28 fractions of 1.8 Gy, such that at least 99% of the volume had to be covered by 95% of the dose (V95% = 99%). All proton (IMPT) and photon (VMAT, IMRT, TOMO) treatment plans were generated in centers with significant clinical experience in treatment planning.
Dose constraints
The dose limits and priorities for the OARs were defined in the protocol of this in silico comparative study (see ). The dose limits to the brainstem, brain, spinal cord, chiasm, optic nerves and retina were not to be exceeded. Attempts were made to minimize the dose to the other OARs without causing underdosage of the PTV50.4Gy.
Photon planning
The IMRT and VMAT treatment plans were created at MAASTRO clinic using Eclipse™ (v11.0 Varian Medical Systems, Palo Alto, CA). For VMAT plans, one or two 10 MV half- or whole arcs were used. For IMRT, the planner optimized the beam angles such that OAR were optimally spared, using 4–7 beams of 10 MV each. The TOMO treatment plans were created at Radiotherapiegroep Deventer using Accuray Hi-Art Planning Station (v5.1.0.4, Accuray, Sunnyvale, CA). The photon plans using a PTV margin were considered to be innately robust relative to PT, since more than 95% of the PTV received 99% of the prescribed dose for all three photon modalities. A grid size of 2–3 mm was used for all modalities depending on the slice spacing of the dataset.
Proton planning
Proton treatment plans were calculated at OncoRay (Dresden, Germany) using RayStation (v4.65.99, RaySearch Laboratories AB, Stockholm, Sweden) and IMPT for beam delivery with pencil beam scanning (PBS). Treatment plans were created for each patient using a robust optimized plan with mostly two radiation beams. The air-gap used between the patient surface and the range shifter was 2 cm. The beam direction was chosen individually for every patient in order to spare the OARs and healthy brain tissue, and to avoid passing through air cavities. The minimum energy of the beam model used was 100 MeV with a spot size sigma ranging from 4 mm (220 MeV) to 8 mm (100 MeV) in the isocenter. These robust plans were generated optimizing the target coverage for the CTV, thus no PTV concept was used, including a setup uncertainty of 2 mm and a range uncertainty 3.5%, and considering 21 scenarios for the optimization process. A range shifter of approximately 7.5 cm water equivalent and a calculation grid of 3 mm were used in all cases.
Storage of imaging datasets
The datasets were stored and exchanged through the secured collaborative MISTIR platform hosted by MAASTRO clinic. Quality assurance procedures were applied to assess the necessity of corrections of potential transformations during import and export in the respective treatment planning systems [Citation19].
Data evaluation and statistical analysis
For each treatment plan, the mean (Dmean) and maximum dose (Dmax) as well as the near-maximum dose, defined as the highest dose to 2% of the volume (D2%) or to 0.1% (D0.1cc) in case 2% was smaller than 2 cc, were calculated for each OAR and CTV [Citation20]. The mean dose to the imaged part of the patient (body contour) minus CTV was defined as the mean integral dose (ID).
For statistical analyses, an in-house developed script in Matlab (version 2017a, The MathWorks, Natick, MA) was used to extract dose-volume-histograms (DVH) metrics from the 3D dose distributions that were uploaded by the participating centers. To allow for a direct comparison between all treatment modalities, the doses to the CTV were considered as no PTV was used for protons. The doses were scaled such that 99% of the CTV received exactly 100% of the prescribed dose (50.4 Gy). Whenever needed, scaling was increased to be sure that the GTV was covered with at least 50.4 Gy. This was required in four patients with a factor between 0.98 and 1.16.
Considering the fact that VMAT was used for the actual treatment of the 25 LGG patients included in this study, it was considered the gold standard for the comparative analyses. The DVH metrics and doses to OARs were statistically compared using two-tailed, Wilcoxon’s signed-rank test to determine the significance of pairwise differences compared to VMAT. Accounting for multiple testing and applying a Bonferroni correction, a p value <.02 was considered statistically significant. The van't Riet et al. [Citation21] conformity number (0–1) was used to describe the conformity of the CTV coverage, with 1 indicating a perfect conformity.
To evaluate the TPS performance of robust IMPT planning, six random patients were evaluated with each having 26 scenarios for setup (2 mm) and density (3.5%) changes. Next, the V95% of the CTV prescribed dose was calculated. To quantify the variability in the results, the coefficients of variation (CV = standard deviation over mean) were determined.
Results
In total, 100 treatment plans for the 25 patients with LGG patients were calculated and analyzed. An example of the treatment plans for a patient is presented in . The dose coverage of the CTV50.4Gy was statistically significantly better for TOMO than for VMAT (p = .02; V95% = 99.9%), overall the coverage was excellent for all modalities with the volume receiving 95% of the prescribed dose (V95%) of the CTV50.4Gy ranging from 99.7 to 100% (comparison to gold standard VMAT given in ). The conformity expressed using the van't Riet et al. [Citation21] conformity number showed that VMAT plans had the highest conformity (0.74), followed by TOMO (0.72; p < .02), IMRT (0.69; p < .02) and IMPT (0.69; p < .02; ). In accordance with robust photon plans, the variability in robust planning for the six evaluated IMPT cases proved to be very limited with an average CV of 0.07% (range 0.05–0.12%; Suppl. Table I).
Figure 1. Example of a radiation treatment plans for a patient with a LGG parieto-occipitally in the left hemisphere. The CTV (pink), hippocampus (yellow) and dose distribution (ranging from low dose depicted in blue to high dose in red) are given for the VMAT (A), TOMO (B), IMRT (C) and IMPT (D) treatment plans, in the transverse (upper row), frontal (middle row) and sagittal (lower row) view. Of note is the large low-dose bath when using either of the photon techniques (A–C).
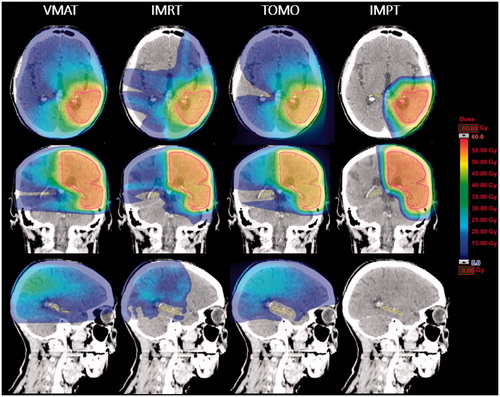
Table 2. Dose and coverage parameters per organ at risk or target volume per treatment modality (significance calculated in comparison to the gold standard VMAT).
The mean ID was significantly increased for TOMO (8.3 Gy; p < .02) and IMRT (7.9 Gy, p < .02) and decreased for IMPT (5.6 Gy, p < .02) compared to VMAT (7.8 Gy). shows the average scaled values of the Dmean and D2% for the OARs delivered by IMRT, TOMO and IMPT in comparison to VMAT; overall, the Dmean of the OARs statistically significantly differed from VMAT for IMRT, TOMO and IMPT, being 1/10 (10%), 3/10 (30%) and 9/10 (90%), respectively. For the D2%, a statistically significant dose reduction was found in 3/12 (25%), 6/12 (50%) and 10/12 (83%) of the OARs. IMPT was the prime modality reducing dose to the OARs followed by TOMO. The pituitary gland was best spared by TOMO ().
The brain volume receiving a dose up to 30 Gy (V5Gy – V30Gy) was statistically significantly reduced using IMPT compared to VMAT (comparison of the percentage of hippocampus, posterior cerebellum and brain to the gold standard VMAT is given in ); the V5Gy being 85 cc (SD 12 cc) for VMAT versus 49 cc (SD 16.3 cc) for IMPT and the V20Gy 56 cc (SD 19 cc) versus 39 cc (SD 15 cc), respectively (see for 20 Gy (RBE) volume). Besides for brain, the low dose volumes to the hippocampus (bilateral and contralateral) and posterior cerebellum were statistically significantly reduced using IMPT whereas the high dose volumes to hippocampus bi- and ipsilateral, posterior cerebellum and brain increased using IMPT compared to VMAT ().
Figure 2. Three-dimensional representations of the cerebrum and cerebellum of a LGG patient highlighting the CTV in red and the 20 Gy isodose (purple/brown) of the different treatment techniques studied (VMAT, TOMO, IMRT and IMPT).
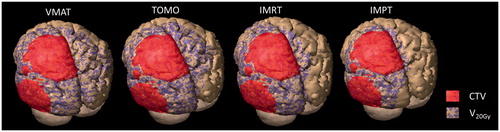
Figure 3. The percentage of organs at risk volume (related to cognition) receiving a radiation dose between 5 Gy and 50 Gy (V5 Gy to V50 Gy, respectively).
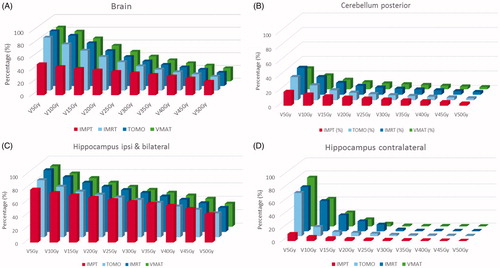
Table 3. Absolute percentage of the hippocampus, posterior cerebellum and brain volumes receiving a dose between 5 and 50 Gy (RBE) presented per treatment modality (statistical comparison versus the gold standard being VMAT).
The mean CTV50.4Gy volume in the included 25 patients was 240 cc (SD 112 cc) with a range of 92–456 cc and a median of 171 cc. With increasing volume of the CTV50.4Gy, the volume of irradiated brain tissue increased for all treatment modalities. Even the slopes for all photon modalities (VMAT, IMRT, TOMO) were identical to that of the IMPT plans. The dose given to a certain volume, e.g., Brain V10Gy, was lower with IMPT than with photon-based treatment modalities, whereas the curves for Brain V40Gy overlapped (Supplementary Figure I).
Discussion
This ROCOCO study is the first to compare VMAT with IMRT, TOMO and IMPT in brain irradiation. IMPT was superior in sparing most OARs compared to VMAT and delivered the lowest ID. IMPT resulted in a significant dose reduction for structures related to cognition, such as non-target brain tissue, the hippocampus (bilateral and contralateral) and the posterior cerebellum. TOMO was the photon technique achieving the lowest dose to the OARs, while VMAT achieved the highest CI.
Thus far, there is limited experience comparing the different treatment techniques for LGG. Koca et al. [Citation22] published an IMRT versus TOMO plan comparison of 20 glioblastoma patients showing TOMO to be superior to IMRT plans in sparing of OARs with slightly broader low dose ranges. Cao et al. [Citation23] compared VMAT with TOMO for 10 body sites concluding comparable plan qualities of VMAT compared to TOMO in most of the cases. Skórska et al. [Citation24] stated in their retrospective plan comparison of 15 brain tumor patients, that the advantage of TOMO was in the highly conformal uniform doses to the target volume, even though these could only be delivered in a coplanar mode. When comparing TOMO to coplanar (IMRT) and non-coplanar (ncIMRT), the median CI were best for TOMO and worst for IMRT. They reported that the largest reduction of Dmax for lenses and Dmean for both eyes was achieved using ncIMRT. While Dmean for the optic chiasm and the ipsilateral optic nerve were best spared using TOMO, the contralateral optic nerve with ncIMRT [Citation25].
Proton therapy has a dosimetric advantage due to its physical characteristics and is known to reduce the ID as was also seen in our in silico trial with a comparable coverage of the target volume and a lower dose to the OARs. Moreover, we found that in particular the dose to the contralaterally located OARs could be reduced. Thus far, publications on clinical experience treating LGG with PT are scarce. Harrabi et al. [Citation25] published the largest retrospective study on 74 LGG patients (median PTV volume 185 cc, range 12–710 cc) treated with PT, generating a non state-of-the art conventional three-dimensional radiotherapy plan for plan comparison. The coverage of the target volume was comparable, also showing a reduction in maximal, mean and IDs to the OARs when using protons, especially in structures located contralaterally to the tumor. Other plan comparison studies concluded that PT reduced the dose to surrounding normal tissues resulting in a significantly reduced whole-brain and -body irradiation [Citation26–28].
In our study, the pituitary gland could impressively be spared using TOMO due to the planning strategy in which all direct photon fluence passing the pituitary gland was abolished using the features of the TOMO binary multileaf collimator [Citation29–31].
There are some shortcomings in our study, which may influence our results. First, all treatment plans were prepared in three different institutes, with their own routine and protocols besides the planning goals specifications which were prescribed by the study protocol. In line with international recommendations, e.g., the different PTV margins for each modality were determined locally based on local uncertainty data. However, this approach was chosen to include experts in their specific fields in this ROCOCO in silico study and, moreover, to reflect actual clinical practice. Second, one may debate about the required number of proton beams and their angles used in IMPT. There is an increasing awareness of the RBE uncertainty at the end of the Bragg peak, causing many proton centers to be cautious and avoiding overlapping spots at the end of the beam and using at least two beams [Citation13].
Third, treatment uncertainties (setup, range and anatomical uncertainties) might significantly influence the difference between planned and delivered dose as was shown by Kraan et al. [Citation32]. It could also be argued that a smaller grid size in IMPT could slightly increase the maximum target dose, but will probably have no effect on the OARs as Rana and Zheng [Citation33] reported in their study varying the grid size from 1 mm to 3 mm for IMPT techniques. They recommend using a grid size of 2.5–3 mm for dose calculations with regard to the calculation time. Fourth, since there is currently no consensus on how to report robustness in a uniform manner, e.g., the suggestions of Liu et al. [Citation34], we only performed a small evaluation using the CV, fully aware of the fact that no absolute limit of this value is known. Further analysis based on a voxel wise minimum and maximum are still under investigation.
Whether PT is indicated in neuro-oncology and outweighs the increased costs is still a matter of debate since no randomized control trials are published demonstrating a clinical benefit of PT. Combs [Citation35] recently summarized and discussed the data on PT for tumors of the CNS in comparison to modern photon therapy, showing that there are only few early data for LGG patients, underlining safety and low toxicity comparable to photons.
Will avoiding a low dose bath to the brain translate into a clinical benefit for the patient, especially regarding one of the most feared side effects: neurocognitive decline? This irreversible toxicity directly affects the patient’s independence and wellbeing [Citation36]. Variable mechanism can influence late cognitive toxicity to the brain related to radiotherapy such as vascular damage, demyelination and white matter changes as well as neurocognitive revalidation strategies [Citation36–40]. Patients mostly exhibited overall stability in cognitive functioning after 5 years follow-up with, in some, more impairment on verbal measurements in tumors located in the left hemisphere and some on endocrine dysfunction. More data on late neurocognitive toxicity after PT as well as photon therapy are needed in relation to quality of life. Notably, the location of radiation dose deposition is considered important, defining type and level of radiation induced toxicity [Citation41]. Moreover, it has to be considered that early side effects of proton and photon therapy for LGG are similar and transient, e.g., alopecia (81%), dermatitis (78%), fatigue (47%) and headache (40%), but bear the potential to adversely affect the patient’s quality of life for several weeks [Citation42,Citation43]. Well-designed prospective studies including endpoints related to neurocognitive functioning and imaging are needed to determine the clinical relevance of low dose deposition to large CNS volumes.
In our study, we did not correct for contrast enhancement. Iodine CAs used during CT imaging, lead to an increase of the Hounsfield units in tissue with increased CA uptake depending on the CA concentration. This causes errors in the approximation of the tissue composition and thus in the calculation of the proton ranges as described by Wertz and Jäkel [Citation44]. Consequently, this in theory leads to an exaggeration of the ion ranges during irradiation of 1.3 mm, for a tumor with a size of 5 cm. When OARs are close to the target volume this could be relevant [Citation44]. Thus, the use of iodine CA in a planning-CT to be used for PT is highly discouraged. If urgently required in clinical practice, though, two sequential CT scans may be obtained, with the native CT scan being the first.
In order to predict the benefit of dose reduction to OARs, normal tissue complication probability (NTCP) models are needed. For cognition, Gondi et al. [Citation45] described a statistically significant correlation between the dose to 40% of both hippocampi (D40%) and cognitive decline in 18 patients with CNS tumors treated with stereotactic radiotherapy. This model needs validation in proton beam therapy and possible extension concerning the dose to each separate hippocampus, in particular when the ipsilateral hippocampus is part of the CTV.
Besides the hippocampi more regions in the brain related to neurocognition need to be identified, e.g., the posterior cerebellum. In our recent review [Citation46], we illustrated that there is growing evidence from structural and functional imaging studies that the cerebellum plays an eminent role in neurocognition and that radiation to the posterior cerebellum has a negative effect on neurocognitive outcomes in long-term pediatric brain survivors, besides the multimodality approach.
Since there is the problem of equipoise, the chance of ever performing a randomized trial, besides the costs of such a trial, seems very low. In the Netherlands, the model-based approach has therefore been adopted by care givers and health insurance companies in order to provide an ‘objective’ tool to determine whether a patient is eligible for PT [Citation47]. In this model, a 10% reduction in a grade 2 toxicity is needed using a validated (currently photon based) NTCP model. Unfortunately, there are currently no validated models for CNS. Therefore, uniform prospective collection of future toxicity data in a standardized way is urgently required for photon as well as PT especially for low-dose-large-volume conditions. This will enable upfront assessment of a patient’s likelihood to benefit from particle treatment. Uniform delineation and consensus on the tolerance on OARs are the first steps to achieve this besides a structured follow up including uniform neuro-cognitive tests [Citation18,Citation48]. This potentially improved quality of life ought to be outweighed against the additional costs of particles (protons, carbon ions) over technically advanced photon treatments. Changing the priorities as set in this study could alter outcome.
We conclude that IMPT can overall better spare organs than the other techniques, especially those OARs located contralateral to the target volume. In absence of NTCP models, an alternative approach, which will be implemented for supratentorial gliomas in The Netherlands, is to look at the reduction in mean dose to the brain and both hippocampi, excluding the CTV, achieved when using PT. However, the correlation of dose reduction to clinically relevant gain needs to be further investigated. Whether this will eventually lead to a significant improvement of quality of life needs to be determined in carefully designed future multicenter clinical studies.
Supplemental Material
Download Zip (271.2 KB)Disclosure statement
The authors D.B.P. Eekers MD, E. Roelofs PhD, M. Cubillos-Mesías MSc, C. Niёl MD, R.J. Smeenk PhD MD, A. Hoeben PhD MD, A. Minken PhD, M. Granzier, G.O.R.J. Janssens PhD MD, Professor J.H.A.M. Kaanders PhD MD, Professor E.G.C. Troost PhD MD declared no conflict of interest. Professor P. Lambin PhD MD is a recipient of research grant from www.pttheragnostic.com. Dr. Philippe Lambin reports, within and outside he submitted work, grants/sponsored research agreements from Varian medical, Oncoradiomics, ptTheragnostic, Health Innovation Ventures and DualTpharma. He received an advisor/presenter fee and/or reimbursement of travel costs/external grantwriting fee and/or in kind manpower contribution from Oncoradiomics, BHV, Merckand Convert pharmaceuticals. Dr. Lambin has shares in the company Oncoradiomics SA and Convert pharmaceuticals SA and is co-inventor of two patents onradiomics (PCT/NL2014/050248, PCT/NL2014/050728) licensed to Oncoradiomics and one patent on mtDNA (PCT/EP2014/059089) licensed to ptTheragnostic/DNAmito, three non-pate table invention (softwares) licensed to ptTheragnostic/DNAmito, Oncoradiomics and Health Innovation Ventures.
References
- Crocetti E, Trama A, Stiller C, et al. Epidemiology of glial and non-glial brain tumours in Europe. Eur J Cancer. 2012;48:1532–1542.
- Eckel-Passow JE, Lachance DH, Molinaro AM, et al. Glioma groups based on 1p/19q, IDH, and TERT promoter mutations in tumors. Cancer Res. 2015;75:4884–4894.
- Brat DJ, Verhaak RG, Aldape KD, et al. Cancer genome atlas research network. comprehensive, integrative genomic analysis of diffuse lower-grade gliomas. N Engl J Med. 2015;372:2481–2498.
- Wesseling P, Capper D. WHO 2016 classification of gliomas. Neuropathol Appl Neurobiol. 2018;44:139–150.
- Van der Vlis TAMB, Hoeben A, Beckervordersandforth JC, et al. Impact of the revised WHO classification of diffuse low-grade glioma on clinical decision making: a case report. Surg Neurol Int. 2017;8:223.
- Buckner JC, Shaw EG, Pugh SL, et al. Radiation plus procarbazine, CCNU, and vincristine in low-grade glioma. N Engl J Med. 2016;374:1344–1355.
- Edelstein K, Richard NM, Bernstein LJ. Neurocognitive impact of cranial radiation in adults with cancer: an update of recent findings. Curr Opin Support Palliat Care. 2016;11:1–37.
- Chakraborty S, Patil VM, Babu S, et al. Locoregional recurrences after post-operative volumetric modulated arc radiotherapy (VMAT) in oral cavity cancers in a resource constrained setting: experience and lessons learned. BJR. 2015;88:20140795.
- Gondi V, Pugh SL, Tomé WA, et al. Preservation of memory with conformal avoidance of the hippocampal neural stem cell compartment during whole-brain radiotherapy for brain metastases (RTOG 0933): a phase 2 multi-institutional trial. JCO. 2014;32:3810–3816.
- Tian X, Liu K, Hou Y, et al. The evolution of proton beam therapy: current and future status. Mol Clin Oncol. 2017;8:15–21.
- Sakurai H, Ishikawa H, Okumura T. Proton beam therapy in Japan: current and future status. Jpn J Clin Oncol. 2016;46:885–892.
- Austin AM, Douglass MJJ, Nguyen GT, et al. A radiobiological Markov simulation tool for aiding decision making in proton therapy referral. Phys Med. 2017;44:72–82.
- Lühr A, von Neubeck C, Krause M, et al. Relative biological effectiveness in proton beam therapy – current knowledge and future challenges. Clin Transl Radiat Oncol. 2018;9:35–41.
- Eekers DB, Roelofs E, Jelen U, et al. Benefit of particle therapy in re-irradiation of head and neck patients. Results of a multicentric in silico ROCOCO trial. Radiother Oncol. 2016;121:387–394.
- Van der Laan HP, van de Water TA, van Herpt HE, et al. The potential of intensity-modulated proton radiotherapy to reduce swallowing dysfunction in the treatment of head and neck cancer: a planning comparative study. Acta Oncol. 2013;52:561–569.
- Roelofs E, Engelsman M, Rasch C, et al. ROCOCO Consortium. Results of a multicentric in silico clinical trial (ROCOCO): comparing radiotherapy with photons and protons for non-small cell lung cancer. J Thorac Oncol. 2012;7:165–176.
- Eekers DBP, Roelofs E, Mesías C, et al. Data from: intensity-modulated proton therapy decreases dose to organs at risk in low-grade glioma patients: results of a multicentric in silico ROCOCO trial. Cancer Data. 2017. DOI:10.17195/candat.2018.05.1
- Eekers DBP, in ’t Ven L, Roelofs E, et al. The EPTN consensus-based atlas for CT- and MR-based contouring in neuro-oncology. Radiother Oncol. 2018. DOI:10.1016/j.radonc.2017.12.013
- Roelofs E, Persoon L, Qamhiyeh S, et al. Design of and technical challenges involved in a framework for multicentric radiotherapy treatment planning studies. Radiother Oncol. 2010;97:567–571.
- ICRU. International Commission on Radiation Units and Measurements. Prescribing, recording, and reporting photon-beam intensity-modulated radiation therapy (IMRT). ICRU Report 83. J ICRU. 2010;10:1–106.
- Van't Riet A, Mak AC, Moerland MA, et al. A conformation number to quantify the degree of conformality in brachytherapy and external beam irradiation: application to the prostate. Int J Radiat Oncol Biol Phys. 1997;37:731–736.
- Koca T, Basaran H, Sezen D, et al. Comparison of linear accelerator and helical tomotherapy plans for glioblastoma multiforme patients. Asian Pac J Cancer Prev. 2014;15:7811–7816.
- Cao D, Holmes TW, Afghan MK, et al. Comparison of plan quality provided by intensity-modulated arc therapy and helical tomotherapy. Int J Radiat Oncol Biol Phys. 2007;69:240–250.
- Skórska M, Piotrowski T, Kaźmierska J, et al. A dosimetric comparison of IMRT versus helical tomotherapy for brain tumors. Phys Med. 2014;30:497–502.
- Harrabi SB, Bougatf N, Mohr A, et al. Dosimetric advantages of proton therapy over conventional radiotherapy with photons in young patients and adults with low-grade glioma. Strahlenther Onkol. 2016;192:759–769.
- Dennis ER, Bussiere MR, Niemierko A, et al. A comparison of critical structure dose and toxicity risks in patients with low grade gliomas treated with IMRT versus proton radiation therapy. Technol Cancer Res Treat. 2013;12:1–9.
- Beltran C, Roca M, Merchant TE. On the benefits and risks of proton therapy in pediatric raniopharyngioma. Int J Radiat Oncol Biol Phys. 2012;82:e281–e287.
- Schneider U, Lomax A, Pemler P, et al. The impact of dose escalation on secondary cancer risk after radiotherapy of prostate cancer. Int J Radiat Oncol Biol Phys. 2007;68:892–897.
- Sherman JC, Colvin MK, Mancuso SM, et al. Neurocognitive effects of proton radiation therapy in adults with low-grade glioma. J Neurooncol. 2016;126:157–164.
- Shih HA, Sherman JC, Nachtigall LB, et al. Proton therapy for low-grade gliomas: results from a prospective trial. Cancer. 2015;121:1712–1719.
- Brown PD, Buckner JC, Uhm JH, et al. The neurocognitive effects of radiation in adult low-grade glioma patients. Neuro-oncology. 2003;5:161–167.
- Kraan AC, van de Water S, Teguh DN, et al. Dose uncertainties in IMPT for oropharyngeal cancer in the presence of anatomical, range, and setup errors. Int J Radiat Oncol Biol Phys. 2013;87:888–896.
- Rana S, Zheng Y. Impact of grid size on uniform scanning and IMPT plans in XiO treatment planning system for brain cancer. J Appl Clin Med Phys. 2015;16:447–456.
- Liu W, Patel SH, Shen JJ, et al. Robustness quantification methods comparison in volumetric modulated arc therapy to treat head and neck cancer. Pract Radiat Oncol. 2016;6:e269–e275.
- Combs SE. Does proton therapy have a future in CNS tumors? Curr Treat Options Neurol. 2017;19:12.
- Klein M. Treatment options and neurocognitive outcome in patients with diffuse low-grade glioma. J Neurosurg Sci. 2015;59:383–392.
- Douw L, Klein M, Fagel SS, et al. Cognitive and radiological effects of radiotherapy in patients with low-grade glioma: long-term follow-up. Lancet Neurol. 2009;8:810–818.
- Armstrong CL, Hunter JV, Ledakis GE, et al. Late cognitive and radiographic changes related to radiotherapy: initial prospective findings. Neurology. 2002;59:40–48.
- Ravn S, Holmberg M, Sørensen P, et al. Differences in supratentorial white matter diffusion after radiotherapy – new biomarker of normal brain tissue damage? Acta Oncol. 2013;52:1314–1319.
- Olson JD, Riedel E, DeAngelis L. Long-term outcome of low-grade oligodendroglioma and mixed glioma. Neurology. 2000;54:1442–1448.
- Peiffer AM, Leyrer CM, Greene-Schloesser DM, et al. Neuroanatomical target theory as a predictive model for radiation-induced cognitive decline. Neurology. 2013;80:747–753.
- Hauswald H, Rieken S, Ecker S, et al. First experiences in treatment of low-grade glioma grade I and II with proton therapy. Radiat Oncol. 2012;7:189.
- Wilkinson B, Morgan H, Gondi V, et al. Low levels of acute toxicity associated with proton therapy for low-grade glioma: a Proton Collaborative Group study. Int J Radiat Oncol Biol Phys. 2016;96:E135.
- Wertz H, Jäkel O. Influence of iodine contrast agent on the range of ion beams for radiotherapy. Med Phys. 2004;31:767–773.
- Gondi V, Hermann BP, Mehta MP, et al. Hippocampal dosimetry predicts neurocognitive function impairment after fractionated stereotactic radiotherapy for benign or low-grade adult brain tumors. Int J Radiat Oncol Biol Phys. 2013;85:348–354.
- Eekers DBP, in 't Ven L, Deprez S, et al. The posterior cerebellum, a new organ at risk? Clin Transl Radiat Oncol. 2018;8:22–26.
- Langendijk JA, Lambin P, De Ruysscher D, et al. Selection of patients for radiotherapy with protons aiming at reduction of side effects: the model-based approach. Radiother Oncol. 2013;107:267–273.
- Lambrecht M, Eekers DBP, Alapetite C, et al. Radiation dose constraints for organs at risk in neuro-oncology. Radiother Oncol. 2018. DOI:10.1016/j.radonc.2018.05.001