Abstract
Seventeen-year periodical cicadas represent an extreme life history, with individuals spending 17 years as juveniles and less than 45 days as adults. The implications of this life history on investment in immunity are unknown. Using monofilament implants, we examined sex differences in melanization and encapsulation-based immunity in Brood X Magicicada septendecim and M. cassini periodical cicadas. In many insects, females devote more effort to immunity than males which allows females additional time to lay eggs before death. In 17-year cicadas, the functional difference in lifespan between males and females is trivial as compared to their total lifespan. However, we found that in both species, adult females had better immunity (encapsulation response) than males. In both species, male immunity (melanization and encapsulation) declined as they aged, as did female encapsulation. However, female immunity (melanization) either did not decline or improved with age. We conclude that although male immunosenescence resembles that of shorter-lived insects, for females, melanization-based immunity is preserved as they age, potentially due to their unique life history. We also examined whether being housed in mixed-sex versus single-sex enclosures affected immunity but did not find an effect of reproductive opportunity on either melanization or encapsulation in males or females of either species. This is the first study to assay melanization or encapsulation in a cicada, so our results provide a first look at sex- and age-based immunity in this group.
INTRODUCTION
Magicicada periodical cicadas are remarkable as they are the longest-lived of all insects (Lloyd & Dybas Citation1966a; Simon et al. Citation2022). Magicicada live for 13 or 17 years, synchronously emerging from the ground in the final months of their lives to pupate into adults, reproduce and die. Classical models of pace of life predict that a fast pace of life is associated with high predation risk, and these individuals develop rapidly into small adults who produce many small offspring. Conversely, when there is a low daily risk of mortality, individuals can develop slowly into large adults who produce fewer, larger offspring (Williams Citation1957; Brown et al. Citation2022). However, these models fail to adequately describe periodical cicadas that develop very slowly into small adults that produce many small offspring (Brown et al. Citation2022). One key difference between periodical cicadas and other slow-paced species is that periodical cicadas, like many insects with complete development, experience two morphologically and ecologically distinct life stages. In periodical cicadas, the underground juvenile stage is characterized by low predation risk and slow growth; the above ground adult stage is characterized by high predation risk, the production of many small offspring and rapid senescence (Karban Citation2022). In its fast pace of life, the adult life stage of Magicicada cicadas superficially resembles that of many insects that live a single season. As adults, seasonal insects experience trade-offs between somatic maintenance (including immunity) and reproduction, and rapidly shift their investment in reproduction versus immunity as their reproductive potential peaks and then diminishes (Jervis & Ferns Citation2004). One (of many) unknowns about periodical cicadas is whether adults experience the same pattern of trade-offs between reproduction and immunity as other insects, or whether their extended lifespans confer traits associated with longevity, including increased investment in somatic maintenance and delayed immunosenescence. Further, trade-offs between immunity and reproduction can differ between the sexes (Zuk & Stoehr Citation2002; Nunn et al. Citation2009) and nothing is known about sex differences in immunity in periodical cicadas.
In vertebrates, females frequently have superior immunity to males due to the immunosuppressive effects of testosterone (Roved et al. Citation2017). Insects do not have sex-specific hormones. However, there can still be sex differences in immunity. In many studies, female insects have better immune responses than males (Gray Citation1998; Adamo et al. Citation2001; Gershman et al. Citation2010; Bagchi et al. Citation2021; Letendre et al. Citation2022). These differences can be attributed to differences between males and females in their life history strategies (Zuk & Stoehr Citation2002; Nunn et al. Citation2009). While males need only live long enough to secure matings, females need to mate and then produce offspring. Consequently, males can maximize their fitness by sacrificing longevity to increase mating effort. However, females need to invest more in somatic maintenance, including immunity, to maximize their reproductive output. Thus, it is predicted that females should invest more in immunity than males (Rolff Citation2002; Zuk & Stoehr Citation2002). However, there is substantial variation in the outcome of studies of sex-specific immunity, with many studies finding either no effect of sex on immunity or male-biased immunity (Kelly et al. Citation2018). In a meta-analysis by Kelly et al., there was a trend toward female-biased immunity in insects, although it was not statistically significant, and also substantial variation in sex-biased immunity based on the type of immune response measured.
Mating has the potential to harm immunity. In vertebrates, the hormones necessary to express sexually selected traits can impair male immunity (Klein Citation2000). In invertebrates, immunological costs can be mediated by hormones (Rolff & Siva-Jothy Citation2002) or the result of trade-offs between allocation of resources to reproduction versus somatic maintenance and immunity (Sheldon & Verhulst Citation1996; Lawniczak et al. Citation2007). For example, in male orthopterans that produce large courtship feedings, mating reduces the ability of males to mount an immune response; conversely, experiencing an immune challenge reduces the ability of males to reproduce (Leman et al. Citation2009; Kerr et al. Citation2010). For females, sexual conflict can make mating costly. Male–male competition for access to mating opportunities can incidentally harm females (Chapman et al. Citation2003). In Drosophila melanogaster flies and Caenorhabditis elegans nematodes, the seminal products that increase male success in sperm competition (Chapman et al. Citation2000) can reduce female lifespan (Fowler & Partridge Citation1989; Gems & Riddle Citation1996; Gordon et al. Citation2022). In species such as bed bugs and dung flies that mate via traumatic insemination, copulation can cause physical damage to females (Crudgington & Siva-Jothy Citation2000; Blanckenhorn et al. Citation2002). Female invertebrates can also experience trade-offs between reproduction and immunity. In diverse insects, an immune challenge can reduce female future reproduction (weta: Kelly Citation2011; house cricket: Bascuñán-García et al. Citation2010; mosquito: Ahmed et al. Citation2002); mating and the cost of devoting resources to provisioning offspring can decrease the ability of females to cope with future immune challenges (ground crickets: Fedorka et al. Citation2007; mealworm beetles: Rolff & Siva-Jothy Citation2002; wood ants: Castella et al. Citation2009). When mating causes harm, the relative cost of mating for males and females can differ across taxa (Schwenke et al. Citation2016) due to differences in reproductive investment (Rolff Citation2002).
Immunity is complex, even in invertebrates. Although invertebrates lack adaptive immunity (but see Sadd & Schmid-Hempel Citation2006; Cooper & Eleftherianos Citation2017), they still have the ability to defend themselves from pathogens using multiple immune responses, including reactive oxygen species, and antimicrobial peptides and phagocytosis. Any foreign body that is too large to be disabled by phagocytosis can be smothered with layers of hemocytes, forming a nodule or capsule around the pathogen (Siva-Jothy et al. Citation2005). Another powerful immunological defense mechanism is the production of melanin; when faced with an invading organism, insects can rapidly coat and immobilize it with melanin (González-Santoyo & Córdoba-Aguilar Citation2012). Coating a pathogen with melanin prevents its growth and reproduction and eventually causes its death (Nakhleh et al. Citation2017). Large pathogens like parasitoids can be subdued by encapsulating them in both layers of cells and melanin (Siva-Jothy et al. Citation2005). In our study, we used melanization and encapsulation to assay immunity of cicadas for two reasons. First, encapsulation represents a coordinated immune response of both cellular and humoral immunity. Thus, it is possible to measure the total effect of multiple arms of the immune system in a single assay. Second, cicadas have relatively little hemolymph to extract. Although cicadas can suck large quantities of plant fluids, they also rapidly excrete most of the liquid content of their food (Karban Citation2022). Our preliminary experiments demonstrated that it was not possible to extract enough hemolymph from a live cicada to assay phenoloxidase activity or bacterial cell lytic activity. Given that we had to choose a single assay of immunity per individual, we chose encapsulation because it was the most comprehensive assay.
The focus of our study is Brood X periodical cicadas. In the US, synchronous cohorts of periodical cicadas are referred to as “broods”. The geographic ranges of broods are tracked over time, and each brood has been assigned a number based on its schedule of emergence. In the northern parts of the US, periodical cicadas have a 17-year cycle, while in the southern US, they have a 13-year cycle. Brood X is the cohort of 17-year cicadas that most recently appeared in 2021 across Indiana and eastern Ohio, and Pennsylvania, Maryland and Delaware (Cooley et al. Citation2009; Kritsky Citation2021). Each brood is composed of multiple species of periodical cicada. In the southern 13-year cicadas, there can be up to four species per brood, and in the northern 17-year cicadas, there are three species: Magicicada cassini (also known as M. cassinii, see Marshall Citation2022), M. septendecim, and M. septendecula (Alexander & Moore Citation1962). At our study site, we observed (and heard) mass numbers of M. cassini and M. septendecim but observed relatively few M. septendecula. Consequently, we did not include M. septendecula in our study.
In Part 1 of our study, for each species of cicada, we examined the effect of sex, adult age and the interaction between sex and age on immunity. Many previous studies on insects have found sex differences in immunocompetence, although the direction of the difference can vary (Kelly et al. Citation2018), so we predicted a sex difference in encapsulation, but we had no a priori prediction about which sex would have superior immunity. We also predicted that standing immunity would decline as adults aged and senesced, as this is a normal component of ageing (Mackenzie et al. Citation2011; Park et al. Citation2011). In general, when reproduction is costly, immunity can suffer (Sheldon & Verhulst Citation1996; Lawniczak et al. Citation2007). Across insects, the effects of reproduction on immunity can vary. Few studies have examined immunity in cicadas, and no previous studies have measured standing immunity in 13- or 17-year periodical cicadas. So it is unclear whether reproduction is more costly for males who exert continuous effort singing, or females who produce eggs and oviposit in wood. We examined whether there was an interaction between sex and adult age to determine which sex (if any) suffered a larger drop in standing immune effort over the month of adulthood. In Part 2 of our study, for each species of cicada, we manipulated the opportunity for mating for males and females and examined the effects on encapsulation. Our a priori hypothesis is that the opportunity to mate will be immunologically costly for both sexes. However, given that male cicadas do not provide any form of courtship feeding or costly material benefit, we predict that mating will be more immunologically costly for females than for males.
METHODS
Cicada collection
All cicadas were collected by hand from a suburban housing subdivision adjacent to Highbanks Metro Park north of Columbus (40°09’42”N, 83°01’46”W). The subdivision was built in the 1980s and contains large deciduous trees that pre-date the construction of houses. In this area, individual Brood X cicadas started to appear during the week of 20 May 2021 (Week 1), and mass numbers emerged within the following week (Week 2). In our methods and results, we refer to Magicicada septendecim as “MS” and Magicicada cassini as “MC”. Cicadas emerged en masse from the ground as nymphs early in the morning, climbed plants, shrubbery and trees, then stopped climbing to molt and emerge as adults. Approximately an hour after molting, new adults were still unsclerotized, but were fully inflated and could be identified by sex and species. Cicadas collected as newly eclosed adults (Part 1 “young” adults, all Part 2 adults) were hand collected from trunks and branches of trees from 7 to 9 am on the morning that they emerged from the ground. Although the appearance of cicadas emerging from the ground was broadly synchronous, within the collection site, cicadas emerged en masse from different stands of trees on different days. Consequently, each day, we attempted to collect adults from across the site to include variation due to site and variation due to whether individuals appeared early or late in the mass emergence. Because of the patchy nature of emergence and our inability to predict where and when emergences would occur, we were unable to block for site or collect along planned transects. Each collection day, we selected individuals haphazardly until we had sufficient numbers of each sex and species. After mass emergence, mature adults are active during the day, but rarely fly at night or early morning when it is relatively cool. For our “old” adult treatment, we hand-collected adults clinging to plants, shrubbery and trees from 7 to 9 am. For both “young” and “old” adults, we avoided collecting individuals with morphological damage, but otherwise collected indiscriminately until we had sufficient numbers of each sex and species.
Encapsulation assay
To determine the encapsulation abilities of individual adult cicadas, we inserted an implant into each cicada. For implants, we used a 3-mm roughened segment of 8-lb monofilament fishing line. The time of day that implants are implanted has the potential to affect the encapsulation response (Qiu et al. Citation2023). Due to the brief time period that Brood X cicada adults emerge, we would have been unable to perform multiple replicate blocks of surgeries at the same time of day. Thus, we chose to use a 24-hr period to measure encapsulation. Most Brood X cicadas were able to heavily melanize an implant within 24 hr, so we measured both the darkness of the implant and the accretion of melanized cells to the outside of the implant.
Implants are meant to simulate a parasitoid fly larva that has penetrated the cicada’s body. We can then assay the ability of each cicada to subdue a parasitoid with melanin and encapsulate it with host cells. When cicadas are attacked by parasitoid flies, the flies leave their larvae on the dorsal side of the cicada and the larvae burrow into the cicada’s body through an intersegmental membrane (Stucky Citation2015). In our study, we used a 23-gauge needle to pierce a hole in each cicada’s dorsal side through the integument between the mesonotum and the first tergite. We then inserted the implant into the hole at an angle parallel to the cicada’s body wall, until the implant was no longer visible. Twenty-four hr after implantation, we euthanized each cicada by rapid freezing, dissected out the implant and assayed the darkness of melanin and area of accumulated cells that adhered to the implant.
After implantation, cicadas were held at 26 °C in individual 10 × 10 × 10 cm plastic containers with mesh tops and moist paper towels for substrate. Twenty-four hr after each individual’s implantation time, they were transferred into a 25 × 95 mm plastic vial and placed in a − 20 °C freezer to euthanize the cicada and stop implant melanization and cell accumulation. Several months later, each cicada was thawed for 5 min, and implants were dissected out, placed on a slide and immediately photographed to avoid any desiccation shrinkage. In several cases, dissection revealed that the implant had partially worked its way out of the cicada’s body. In those instances, only the portion of the implant that remained in the cicada’s body was measured for melanin and cell accumulation. Implants were photographed using a digital camera (Celestron 1080 HD).
To determine the melanization of each implant, we used NIH ImageJ (https://imagej.net/ij/) to outline the longest 3-mm-wide rectangle within each implant and determine its average grayscale. In ImageJ, grayscale ranges from 0 (black) to 256 (white). Because it may be visually confusing in our figures for smaller values to indicate more encapsulation, for -, we subtracted each average grayscale measurement from 125, our largest value grayscale. We refer to this variable as “implant darkness”. With this transformation, our lightest implant had an implant darkness score of 2, and our darkest implant had an implant darkness score of 125.
To determine cell accumulation around each implant, we used the ImageJ tracing tool to automatically outline the implant and calculate the area within the outline. Next, we drew the longest possible 3 mm line within each implant, representing the most conservative estimate of the size of the original implant, and calculated its area. To determine the 2-dimensional area of cell accumulation around each implant, we subtracted the area of the original implant from the total outline area. To control for unintentional variation in original implant lengths and also to allow any partially extruded implants to remain in the analysis, we divided the area of cellular accumulation (total outline area minus original implant area) by the total outline area. This gave us the proportion of the total implant area composed of cellular accumulation. We refer to this variable throughout the paper as “implant thickness”.
Body size
We measured head width (distance between eyes) as a proxy for skeletal body size. Although we had no a priori hypotheses about the effect of body size on encapsulation, we measured body size as a possible covariate explaining variation in implant darkness and implant thickness. As expected, we found that M. septendecim was larger than M. cassini (Alexander & Moore Citation1962), and females were larger than males (Koyama et al. Citation2015) in each species (Table S2 in Supplemental Data). However, when each species and sex were examined separately, we did not find a significant effect of head width on either implant darkness or implant thickness in Part 1 or Part 2 (Tables S3, S9 in Supplemental Data). Consequently, we have not included head width in subsequent analyses.
Part 1: the effect of age and sex on melanization and encapsulation
In this experiment, cicadas were collected at the beginning and end of their adult lifespans and implanted with monofilament to evaluate encapsulation ability (). The “young” treatment cicadas were collected on Week 2 of the mass emergence. Individuals were collected in two blocks on the day of their emergence: 7–9 am on 27 May and 7–9 am on 31 May. The “young” adults were held overnight outdoors in 30.5 × 30.5 × 30.5 cm wire mesh boxes to allow them to sclerotize their exoskeletons and were then given a monofilament implant to encapsulate the following morning. By the end of Week 2, mass emergence of adults from the ground had ended; the last local sighting of a larval cicada emerging from the ground was in Week 3 (4 June). The “old” treatment cicadas were collected on Week 4 at 8–11 am on 12 June. Adult Brood X cicadas were present for several additional weeks in rapidly declining numbers. In our study, the “young” cicadas were 1-day-old virgins. The “old” cicadas were 1–4 weeks post-adult emergence. The “old” cicadas had the opportunity to mate and oviposit, but the mating status of individuals was unknown. The “old” adults were implanted directly after capture as they were already fully sclerotized. We recruited between 47 and 57 individuals for each combination of species, sex and adult age. See Table S1 in Supplemental Data for exact sample sizes.
Part 2: the effect of mating opportunity on melanization and encapsulation
In this experiment, newly emerged virgin cicadas were held in enclosures containing either all conspecific individuals of the same sex or half conspecific individuals of each sex for approximately 5 days, and then each individual was given a monofilament implant to encapsulate (). Individuals can begin mating at 5 days post-eclosion, but females do not start laying eggs until 9 days post-eclosion (Karban Citation1981), so our experimental design included the effects of courtship and mating, but not oviposition. Each enclosure was a 61 × 71 cm envelope of lightweight charcoal-colored nylon window screening that were secured around a limb of a live mature Bradford pear tree. Although in our observation, these cultivated trees were too small to contain large choruses of cicadas, we observed many cicadas feeding from and ovipositing in Bradford pear trees. Tree limbs were cleared of all non-experimental cicadas when enclosures were installed. Eight enclosures were filled with (approximately) the following numbers of individuals: one containing 24 MS males, one containing 24 MS females, two containing 12 MS males and 12 MS females, one containing 24 MC males, one containing 24 MC females, two containing 12 MC males and 12 MC females. When we had collected slightly fewer than 48 individuals of each species and sex, we attempted to distribute the number of individuals evenly among treatments (see Table S7 in Supplemental Data for exact sample sizes). We performed two replicate blocks of this experiment.
For Block 1, we caught cicadas at eclosion on 27 May 2021, sorted them by species and sex and held cicadas in 30.5 × 30.5 × 30.5 cm wire mesh boxes. Although we had planned to populate enclosures on 28 May, there was unusually cold weather with heavy rain on 28–29 May, so cicadas were held in the mesh boxes in a sheltered area for their protection and put into enclosures on the morning of 30 May. Individuals were implanted on 4 June, housed in individual containers for 24 hr and frozen on 5 June. For Block 2, individuals were captured on 31 May, put into enclosures on 2 June, implanted on 7 June, and frozen on 8 June.
Statistical methods
We used the Mahalanobis distance method to locate outliers, and we dropped outlying values that were > 3 Mahalanobis distances. In Part 1, no head width values were dropped, four implant darkness values were dropped and two implant thickness values were dropped. In Part 2, no head width values were dropped, 11 implant darkness values were dropped and one implant thickness value was dropped. Individuals who became unwell or died after implantation were retained in the study to avoid having the weakest individuals self-select out of their assigned treatment group. In both Part 1 and Part 2, while head width (body size) and implant thickness were normally distributed, implant darkness was not normally distributed and could not be transformed to normality. Consequently, instead of using a single multivariate model to analyze the effects of treatment on implant darkness and implant thickness, we used non-parametric Wilcoxon/Kruskal-Wallis tests to analyze the effect of treatment on implant darkness and ANOVAs to analyze the effects of treatment on implant thickness.
Part 1 statistical methods
To detect differences between species and sexes in implant darkness and thickness, we used the MS and MC cicadas from the young adult treatment. We used non-parametric Wilcoxon/Kruskal-Wallis tests to determine the effect of species on implant darkness and the effect of sex on implant darkness. We used ANOVA to determine the effect of species, sex and the interaction between species and sex on implant thickness.
For our analysis of the effect of sex and age on implant darkness, because we could only perform nonparametric analyses, we were unable to perform a statistical test of the interaction between sex and age to determine whether males and females change in immunity at different rates as they age. For each species, we performed non-parametric Wilcoxon/Kruskal-Wallis tests to analyze the effect of sex on implant darkness, and the effect of age on implant darkness. We had two blocks of young adults, so we also tested for the effect of block on implant darkness, and found an effect of block on MS males, but no other groups (Table S4 in Supplemental Data). We performed separate Wilcoxon/Kruskal-Wallis tests for each MS male block on the effect of age on implant darkness, and found that for both blocks, old MS males have reduced implant darkness as compared to young MS males (Table S5 in Supplemental Data).
For each species, we performed an ANOVA of the effect of age, sex and the interaction between age and sex on implant thickness. In this study, we had two blocks of young adults, but only one block of old adults; consequently, we could not include block as an independent variable in the full model. We performed ANOVAs on the effect of block on each species and sex and found significant differences in implant thickness between blocks (Table S4 in Supplemental Data). For each species and block, we performed an ANOVA on the effect of sex, age and the interaction between sex and age on implant thickness to confirm that young adult blocks performed similarly to one another (Table S6 in Supplemental Data).
Part 2 statistical methods
We performed nonparametric Wilcoxon/Kruskal-Wallis tests on the effect of mating opportunity treatment (single-sex enclosure or mixed-sex enclosure) for each species and sex (Table S9 in Supplemental Data). For each species, we performed an ANOVA of the effect of sex, mating opportunity treatment and the interaction between sex and mating opportunity treatment on implant thickness (Table S10 in Supplemental Data). In this study, we used two blocks that each included all treatment combinations (males, females, mixed sex) for each species. We tested for an effect of block on implant darkness and implant thickness within each treatment. We found several block effects on implant darkness or thickness in specific treatment groups (Table S11 in Supplemental Data). For each of the treatments that showed block effects, we analyzed each block separately (Table S12 in Supplemental Data). However, including block effects did not alter our overall results. Within each block, we had two replicate mixed-sex enclosures. We looked for differences in implant darkness or thickness among enclosures within each block but did not find any differences (Table S13 in Supplemental Data).
RESULTS
Part 1: the effect of age and sex on melanization and encapsulation
We explored differences in implant darkness and thickness among species and sexes using the adults from our “young adult” treatment. We did not find differences in implant darkness among MS males, MS females, MC males and MC females (Wilcoxon/Kruskal-Wallis χ23 = 6.02, P = 0.11). There were species- and sex-specific differences in implant thickness: MS had thicker implants than MC, and females had thicker implants than males. There was not a statistically significant interaction between the effects of species and sex on implant thickness (). Using post-hoc Tukey HSD tests at a level of alpha < 0.05, we found that females had thicker implants than males, and that female implants in the two species were not significantly different (). MS males had thicker implants than MC males ().
Fig. 2. Mean (± 1 SE) differences in implant thickness among female and male M. septendecim (MS) and M. cassini (MC). Groups that are statistically significantly different according to Tukey’s HSD are shown by different letters (A–C).
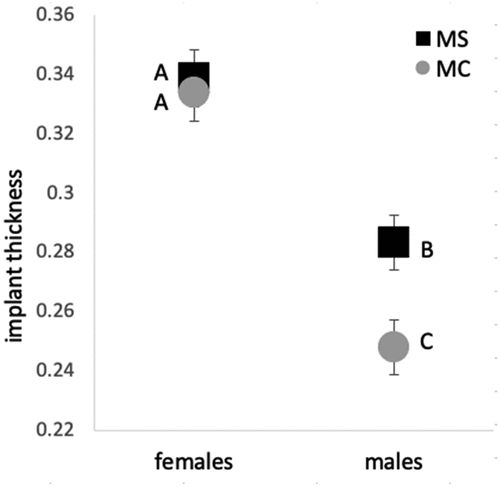
Table 1. The effect of species, sex and the interaction between species and sex on implant thickness. Values that are statistically significant at a level of P < 0.05 are in bold text. N = 47–57 individuals per species and sex. See Table S1 in Supplemental Data for full details about sample sizes.
In both MS and MC, younger males had darker implants than older males (, ). In MC, older females had darker implants than younger females. However, in MS, female implant darkness did not decline as females aged. We found the effect of block on implant darkness in young MS males, so we tested the effect of age on each block separately, and again found young MS males to have darker implants than old MS males (Table S5 in Supplemental Data).
Table 2. The effect of age on implant darkness by species and sex. Nonparametric Wilcoxon/Kruskal-Wallis χ2 tests were used. Values that are statistically significant at a level of P < 0.05 are in bold text. N = 47–57 individuals per species and sex. See Table S1 in supplemental data for full details.
Fig. 3. Mean (± 1 SE) differences in implant darkness (a) and thickness (b) among young and old adult female and male M. septendecim (MS) and mean (± 1 SE) differences in implant darkness (c) and thickness (d) among young and old adult female and male M. cassini (MC).
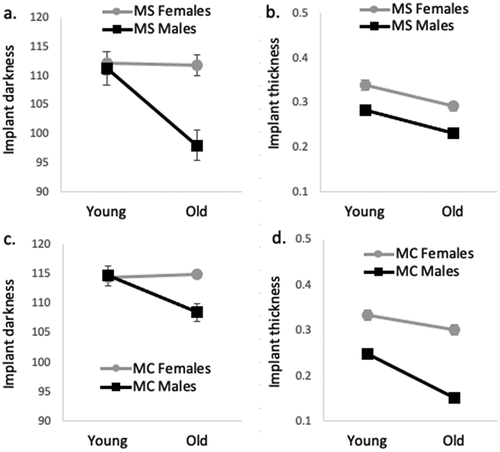
In both MS and MC, females had thicker implants than males. In both MS and MC, younger adults had thicker implants than older adults (, ). In MS, there was not an interaction between the effects of sex and age on implant thickness: male and female immunity declined at the same rate as adults age. In MC, sex and age had interacting effects on implant thickness: both male and female implant thickness declined with age, but male cellular encapsulation declined more (, ). Because we found differences between the two blocks of young adults in implant darkness and thickness (Table S4 in Supplemental Data), we also looked for differences between each block of young adults and old adults. Blocks did not differ substantially from the overall results (Table S5 in Supplemental Data).
Table 3. The effect of age and sex on implant thickness. ANOVAs were performed on each species separately. Values that are statistically significant at a level of P < 0.05 are in bold text. N = 31–46 individuals per species and sex. See Table S7 in Supplemental Data for full details about sample sizes.
Part 2: the effect of mating opportunity on melanization and encapsulation
We did not find an effect of mating opportunity on either implant darkness or implant thickness in either species, for either sex (Tables S10–S11 in Supplemental Data; ).
Fig. 4. Mean (± 1 SE) differences in implant darkness (a) and thickness (b) amongst female and male M. septendecim (MS) housed in mixed sex groups (mating) and same sex groups (no mating) and mean (± 1 SE) differences in implant darkness (c) and thickness (d) amongst female and male M. cassini (MC) housed in mixed sex groups (mating) and same sex groups (no mating).
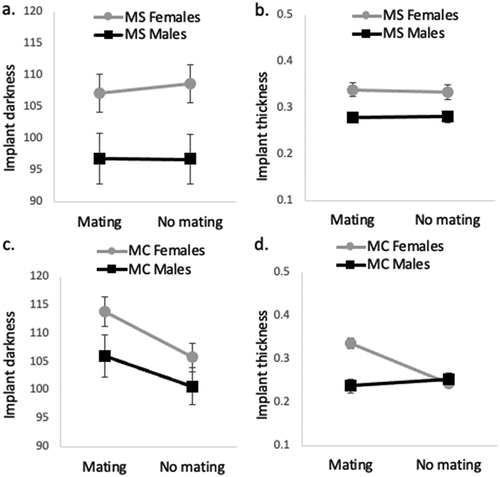
DISCUSSION
In this study, we found effects of species and sex on melanization and encapsulation of a foreign body, with females having superior immunity to males, and M. septendecim having superior immunity to M. cassini. We found that as adult periodical cicadas age, male immunity declines but female immunity remains unchanged. We did not find an effect of mating opportunity on melanization or encapsulation in either M. septendecim or M. cassini.
We found that females had superior ability to encapsulate implants in cellular material than males, across both species. Life history theory predicts that females should put more effort into maintaining immunity than males do so that females can survive long enough to oviposit before they die (Zuk & Stoehr Citation2002; Nunn et al. Citation2009). In a meta-analysis of vertebrate and invertebrate taxa by Kelly et al. (Citation2018), females had better melanization or encapsulation-based immunity than males, and our results are consistent with this. It should be noted that due to differences among taxa in sex-specific physiology, behavior and life-history traits, there is substantial variation among invertebrates in whether females or males have better immunity (Kelly et al. Citation2018). No previous studies have examined sex-specific immunity in a cicada, so our results provide a first look at how males and females may differ in their allocation of resources to different life history traits. Different components of immunity may have different trade-offs with other life history traits and also with each other (Adamo Citation2004), so in future studies, it would be valuable to assay additional components of cicada immunity.
We recorded the body size of each individual in the study. Female cicadas are larger than males (Koyama et al. Citation2015) and M. septendecim are substantially larger than M. cassini (Alexander & Moore Citation1962). Further, larger body size in male cicadas is correlated with louder song (Sanborn & Phillips Citation1995) and increased mating success (Lloyd & Dybas Citation1966b; Karban Citation1983). If individuals with larger body size were in overall better condition, having more resources to devote to both reproduction and immunity, we would expect that skeletal body size would be positively correlated with encapsulation ability. M. septendecim is substantially larger than M. cassini (Alexander & Moore Citation1962) and male M. septendecim had superior encapsulation immunity to the smaller male M. cassini. However, despite the fact that female M. septendecim is larger than female M. cassini (Koyama et al. Citation2015), females of the two species did not differ in their ability to encapsulate implants in cells. Given that body size could not consistently explain differences in encapsulation, it is likely that different species balance their resources between reproduction and immunity differently.
For males and females of both species, we found that cellular encapsulation (implant thickness) declined as adults aged. This result is consistent with many studies that found an age-based decline in immunity in short-lived seasonal insects like Drosophila flies (Mackenzie et al. Citation2011), damselflies (Rolff Citation2001), crickets (Adamo et al. Citation2001; Park et al. Citation2011), bumblebees (Doums et al. Citation2002) and cabbage white butterflies (Stoehr Citation2007). In M. septendecim, we found that male and female implant thickness declined at the same rate. However, for M. cassini, male implant thickness declined at a higher rate than female implant thickness. This suggests that the two species differ in sex-specific allocation of resources to immunity versus other life history traits, with M. cassini females continuing to devote effort to immunity when other groups had allocated effort elsewhere.
We also found differences between females and males in the melanization of implants (implant darkness). While males of both species had declining melanization as they aged, females did not. M. cassini females actually slightly increased implant melanization as they aged, while M. septendecim females did not change as they aged. There are examples in the literature of insects that are able to maintain or augment immunity as they age. In some social insects with more flexible life schedules, ageing individuals can increase investment in immunity (leaf-cutter ants: Armitage & Boomsma Citation2010) or compensate for reduced immunity by increasing anti-ageing antioxidants (Temnothorax ant queens: Negroni et al. Citation2019). One of our initial questions was whether having an extended lifespan makes periodical cicadas allocate resources differently than other insects. Specifically, whether or not the 17-year investment that Magicicada makes in prolonged somatic maintenance carries over into extended immunity and delayed immunosenescence as adults. Our results suggest that males reduce investment in immunity as they age, similar to many shorter-lived insects (Adamo et al. Citation2001). However, females (at least over the span of time that our study took place) continue to invest in immunity. To provide additional support for an association between lifespan and delayed immunosenescence, it would be necessary to collect melanization and encapsulation data on other cicadas for comparison. If delayed immunosenescence is correlated with lifespan, we predict that 13-year Magicicada periodical cicadas and other periodical cicadas (e.g. Chremistica ribhoi World Cup cicadas) would have less of a delay in immunosenescence than 17-year cicadas, while annual cicadas would have a rapid decline in adult immunity. It would also be valuable to collect melanization and encapsulation data from other 17-year cicada broods for comparison to our study system.
Variation in timing of emergence and adult lifespan could potentially add complexity to the interpretation of our results. We were unable to find published experimental documentation of the adult lifespan of males or females of either M. septendecim or M. cassini. However, if females had a slightly longer adult lifespan than males, it is possible that our finding that “old” females had better immunity than “old” males could have been due to differences between males and females in the timing of decline in immunity. If we had been able to resample females several days later, we could have potentially parsed whether older males and females differed in immunity or in timing of immunosenescence. Further, in both species, males emerge slightly (within 24 hr) ahead of females. It is likely that our “old” adult treatment males are, on average, a day older than females. If a day can make a difference in immunity, this could also contribute to differences in immunity between “old” males and females.
In our Part 2 experiment, although we found that, as in Part 1, females had superior implant darkness and thickness to males, we found no effect of mating opportunity on encapsulation in either males or females. There are several possible interpretations of this result. First, it is possible that courtship, copulation and mating do not impact immunity. There are previous studies that have examined the effect of mating on various assays of immunity and have similarly failed to find an effect (Lawniczak et al. Citation2007; Oku et al. Citation2019). A second possibility is that mating has effects on immunity, but our experimental design did not include all potential costs of mating. For example, males housed without direct access to females were not prevented from seeing females or chorusing. If singing bears an immune cost, our experimental design did not allow us to detect it. The timing of our experiment may have limited some factors relevant to females, as well. We tried to keep cicadas in captivity as briefly as possible to avoid escapes, predator attacks and weather issues, and to allow for a second replicate of the study. In our study, cicadas were mature enough for sexual activity in their final 2 days in the enclosure, but females were unlikely to begin to oviposit yet (Karban Citation1981). If oviposition results in an immune cost, this experimental design would have been unable to detect it. Another limitation of our experimental design is that we were unable to quantify the numbers of matings or ensure that each individual in the mixed sex enclosures mated. It is possible that individuals in our study were mating at a lower rate than individuals in the wild. Third, it is possible that the effects of reproductive activity cannot be separated from the effects of ageing in a biologically realistic experimental design. In Part 1, our old adults were both older than our young adults and had presumably also experienced courtship, mating and oviposition. The difference in immunity between young and old adults that we saw in Part 1 could have been due to a combination of reproductive activity and age. The adults in Part 2 had the opportunity to reproduce but were relatively young. It is likely that in the wild, ageing and reproductive activity synergistically reduce immunity. Older adults have had more mating opportunities. Further, only older adult female adults can oviposit (Karban Citation1981). So, it is not possible to separate the interacting effects of age from reproductive activity in this system.
One hypothesis for why Magicicada and other periodical cicadas have such a long periodicity is to thwart antagonistic coevolution with shorter-lived above-ground predators, parasitoids or parasites (Lloyd & Dybas Citation1966a). Although other cicadas have specialist parasitoids (Stucky Citation2015), there are no known specialist parasitoids of Magicicada. However, Magicicada are still vulnerable to both generalist pathogens and long-lived parasites like the Massospora cicadina fungus (Cooley et al. Citation2018). In our study, we found effects of sex, species and ageing on melanization and encapsulation, immune mechanisms used to subdue both parasitoids and microscopic pathogens (Siva-Jothy et al. Citation2005; González-Santoyo & Córdoba-Aguilar Citation2012; Nakhleh et al. Citation2017). One theory for why periodical cicadas emerge simultaneously in mass numbers is to overwhelm the capacity of predators to consume them. Periodical cicadas cluster in dense choruses as part of their courtship and mating behavior. However, this extreme crowding makes them vulnerable to the spread of disease. It would be valuable for future studies to examine whether this extreme crowding affects the ability of individuals to fight subsequent challenges to immunity (like exposure to Massospora cicadina fungus) or, alternatively, potentially bolsters immunocompetence via social immunity (Cremer et al. Citation2007).
ETHICAL STANDARD
This project and manuscript meet institutional and professional ethical standards.
AUTHOR CONTRIBUTION
S.N. Gershman collected the cicadas, conducted the experimental treatments and performed the implants. F. Anagbonu imaged head widths. S.N. Gershman imaged the implants. C.M. Hord and C.C. Cummings measured head width, implant darkness and implant thickness. S.N. Gershman mentored C.M. Hord and C.C. Cummings in the statistical analysis. S.N. Gershman wrote the manuscript.
DATA ACCESSIBILITY
Raw data for these experiments can be found at OSU Knowledge Bank https://kb.osu.edu/handle/1811/103734
Hord et al 2024 Supplemental data R3 highlights.docx
Download MS Word (37.7 KB)ACKNOWLEDGMENTS
We thank Oliver Pittman and Adam Robejsek for their assistance with analyzing images. We thank the Ohio State University at Marion for the use of university-owned equipment.
DISCLOSURE STATEMENT
No potential conflict of interest was reported by the authors.
SUPPLEMENTAL DATA
Supplemental Data for this article can be accessed at https://doi.org/10.1080/03949370.2024.2307028
REFERENCES
- Adamo SA. 2004. How should behavioural ecologists interpret measurements of immunity? Anim Behav. 68(6):1443–1449. doi:10.1016/j.anbehav.2004.05.005
- Adamo SA, Jensen M, Younger M. 2001. Changes in lifetime immunocompetence in male and female Gryllus texensis (formerly G. integer): trade-offs between immunity and reproduction. Anim Behav. 62(3):417–425. doi:10.1006/anbe.2001.1786
- Ahmed AM, Baggott SL, Maingon R, Hurd H. 2002. The costs of mounting an immune response are reflected in the reproductive fitness of the mosquito Anopheles gambiae. Oikos. 97(3):371–377. doi:10.1034/j.1600-0706.2002.970307.x
- Alexander RD, Moore TE. 1962. The evolutionary relationships of 17-year and 13-year cicadas, and three new species (Homoptera, Cicadidae, Magicicada). Misc Publ Mus Zool Univ Mich. 121:1–59.
- Armitage SA, Boomsma JJ. 2010. The effects of age and social interactions on innate immunity in a leaf-cutting ant. J Insect Physiol. 56(7):780–787. doi:10.1016/j.jinsphys.2010.01.009
- Bagchi B, Corbel Q, Khan I, Payne E, Banerji D, Liljestrand-Rönn J, Martinossi-Allibert I, Baur J, Sayadi A, Immonen E, Arnqvist G. 2021. Sexual conflict drives micro- and macroevolution of sexual dimorphism in immunity. BMC Biol. 19(1):114. doi:10.1186/s12915-021-01049-6
- Bascuñán-García AP, Lara C, Córdoba-Aguilar A. 2010. Immune investment impairs growth, female reproduction and survival in the house cricket, Acheta domesticus. J Insect Physiol. 56(2):204–211. doi:10.1016/j.jinsphys.2009.10.005
- Blanckenhorn WU, Hosken DJ, Martin OY, Reim C, Teuschl Y, Ward PI. 2002. The costs of copulating in the dung fly Sepsis cynipsea. Behav Ecol. 13(3):353–358. doi:10.1093/beheco/13.3.353
- Brown JH, Burger JR, Hou C, Hall CA. 2022. The pace of life: metabolic energy, biological time, and life history. Integr Comp Biol. 62(5):1479–1491. doi:10.1093/icb/icac058
- Castella G, Christe P, Chapuisat M. 2009. Mating triggers dynamic immune regulations in wood ant queens. J Evol Biol. 22(3):564–570. doi:10.1111/j.1420-9101.2008.01664.x
- Chapman T, Arnqvist G, Bangham J, Rowe L. 2003. Sexual conflict. Trends Ecol Evol. 18(1):41–47. doi:10.1016/S0169-5347(02)00004-6
- Chapman T, Neubaum DM, Wolfner MF, Partridge L. 2000. The role of male accessory gland protein Acp36DE in sperm competition in Drosophila melanogaster. Proc R Soc Lond B. 267(1448):1097–1105. doi:10.1098/rspb.2000.1114
- Cooley JR, Kritsky G, Edwards MJ, Zyla JD, Marshall DC, Hill KBR, Simon C. 2009. The distribution of periodical cicada. Am Entomol. 55(2):106–112. doi:10.1093/ae/55.2.106
- Cooley JR, Marshall DC, Hill KB. 2018. A specialized fungal parasite (Massospora cicadina) hijacks the sexual signals of periodical cicadas (Hemiptera: Cicadidae: Magicicada). Sci Rep. 8(1):1432. doi:10.1038/s41598-018-19813-0
- Cooper D, Eleftherianos I. 2017. Memory and specificity in the insect immune system: current perspectives and future challenges. Front Immunol. 8:539. doi:10.3389/fimmu.2017.00539
- Cremer S, Armitage SA, Schmid-Hempel P. 2007. Social immunity. Curr Biol. 17(16):R693–R702. doi:10.1016/j.cub.2007.06.008
- Crudgington HS, Siva-Jothy MT. 2000. Genital damage, kicking and early death. Nature. 47(6806):855–856. doi:10.1038/35038154
- Doums C, Moret Y, Benelli E, Schmid-Hempel P. 2002. Senescence of immune defence in Bombus workers. Ecol Entomol. 27(2):138–144. doi:10.1046/j.1365-2311.2002.00388.x
- Fedorka KM, Linder JE, Winterhalter W, Promislow D. 2007. Post-mating disparity between potential and realized immune response in Drosophila melanogaster. Proc Biol Sci. 274(1614):1211–1217. doi:10.1098/rspb.2006.0394
- Fowler K, Partridge L. 1989. A cost of mating in female fruitflies. Nature. 338(6218):760–761. doi:10.1038/338760a0
- Gems D, Riddle DL. 1996. Longevity in Caenorhabditis elegans reduced by mating but not gamete production. Nature. 379(6567):723–725. doi:10.1038/379723a0
- Gershman SN, Barnett CA, Pettinger AM, Weddle CB, Hunt J, Sakaluk SK. 2010. Inbred decorated crickets exhibit higher measures of macroparasitic immunity than outbred individuals. Heredity. 105(3):282–289. doi:10.1038/hdy.2010.1
- González-Santoyo I, Córdoba-Aguilar A. 2012. Phenoloxidase: a key component of the insect immune system. Entomol Exp Appl. 142(1):1–6. doi:10.1111/j.1570-7458.2011.01187.x
- Gordon KE, Wolfner MF, Lazzaro BP. 2022. A single mating is sufficient to induce persistent reduction of immune defense in mated female Drosophila melanogaster. J Insect Physiol. 140:104414. doi:10.1016/j.jinsphys.2022.104414
- Gray DA. 1998. Sex differences in susceptibility of house crickets, Acheta domesticus, to experimental infection with Serratia liquefaciens. J Invert Pathol. 71(3):288–289. doi:10.1006/jipa.1997.4742
- Jervis MA, Ferns PN. 2004. The timing of egg maturation in insects: ovigeny index and initial egg load as measures of fitness and of resource allocation. Oikos. 107(3):449–461. doi:10.1111/j.0030-1299.2004.13453.x
- Karban R. 1981. Effects of local density on fecundity and mating speed for periodical cicadas. Oecologia. 51(2):260–264. doi:10.1007/BF00540611
- Karban R. 1983. Sexual selection, body size and sex-related mortality in the cicada Magicicada cassini. Am Midl Nat. 1(2):324–330. doi:10.2307/2425413
- Karban R. 2022. Why cicadas (Hemiptera: Cicadidae) develop so slowly. Biol J Linn Soc. 135(2):291–298. doi:10.1093/biolinnean/blab152
- Kelly CD. 2011. Reproductive and physiological costs of repeated immune challenges in female Wellington tree weta (Orthoptera: Anostostomatidae). Biol J Linn Soc. 104(1):38–46. doi:10.1111/j.1095-8312.2011.01714.x
- Kelly CD, Stoehr AM, Nunn C, Smyth KN, Prokop ZM, Hosken D. 2018. Sexual dimorphism in immunity across animals: a meta‐analysis. Ecol Lett. 21(12):1885–1894. doi:10.1111/ele.13164
- Kerr AM, Gershman SN, Sakaluk SK. 2010. Experimentally induced spermatophore production and immune responses reveal a trade-off in crickets. Behav Ecol. 21(3):647–654. doi:10.1093/beheco/arq035
- Klein SL. 2000. Hormones and mating system affect sex and species differences in immune function among vertebrates. Behav Proc. 51(1–3):149–166. doi:10.1016/S0376-6357(00)00125-X
- Koyama T, Ito H, Kakishima S, Yoshimura J, Cooley JR, Simon C, Sota T. 2015. Geographic body size variation in the periodical cicadas Magicicada: implications for life cycle divergence and local adaptation. J Evol Biol. 28(6):1270–1277. doi:10.1111/jeb.12653
- Kritsky G. 2021. One for the books: the 2021 emergence of the periodical Cicada Brood X. Am Entomol. 67(4):40–46. doi:10.1093/ae/tmab059
- Lawniczak MK, Barnes AI, Linklater JR, Boone JM, Wigby S, Chapman T. 2007. Mating and immunity in invertebrates. Trends Ecol Evol. 22(1):48–55. doi:10.1016/j.tree.2006.09.012
- Leman JC, Weddle CB, Gershman SN, Kerr AM, Ower GD, St John JM, Vogel LA, Sakaluk SK. 2009. Lovesick: immunological costs of mating to male sagebrush crickets. J Evol Biol. 22(1):163–171. doi:10.1111/j.1420-9101.2008.01636.x
- Letendre C, Rios‐Villamil A, Williams A, Rapkin J, Sakaluk SK, House CM, Hunt J. 2022. Evolution of immune function in response to dietary macronutrients in male and female decorated crickets. J Evol Biol. 35(11):1465–1474. doi:10.1111/jeb.14093
- Lloyd M, Dybas HS. 1966a. The periodical cicada problem. I. Population ecology. Evolution. 20(2):133–149. doi:10.1111/j.1558-5646.1966.tb03350.x
- Lloyd M, Dybas HS. 1966b. The periodical cicada problem. II. Evolution. Evolution. 20:466–505. doi:10.2307/2406585
- Mackenzie DK, Bussière LF, Tinsley MC. 2011. Senescence of the cellular immune response in Drosophila melanogaster. Exp Gerontol. 46(11):853–859. doi:10.1016/j.exger.2011.07.004
- Marshall DC. 2022. On the spelling of the name of Cassin’s 17-year Cicada, Magicicada cassini (Fisher, 1852) (Hemiptera: Cicadidae). Zootaxa. 5125(2):241–245. doi:10.11646/zootaxa.5125.2.8
- Nakhleh J, El Moussawi L, Osta MA. 2017. The melanization response in insect immunity. Adv Insect Physiol. 52:83–109.
- Negroni MA, Foitzik S, Feldmeyer B. 2019. Long-lived Temnothorax ant queens switch from investment in immunity to antioxidant production with age. Sci Rep. 9(1):7270. doi:10.1038/s41598-019-43796-1
- Nunn CL, Lindenfors P, Pursall ER, Rolff J. 2009. On sexual dimorphism in immune function. Philos Trans R Soc B. 364(1513):61–69. doi:10.1098/rstb.2008.0148
- Oku K, Price TA, Wedell N. 2019. Does mating negatively affect female immune defences in insects? Animal Biol. 69(1):117–136. doi:10.1163/15707563-20191082
- Park Y, Kim Y, Stanley D. 2011. Cellular immunosenescence in adult male crickets, Gryllus assimilis. Arch Insect Biochem Physiol. 76(4):185–194. doi:10.1002/arch.20394
- Qiu JF, Cui WZ, Zhang Q, Dai TM, Liu K, Li JL, Wang YJ, Sima YH, Xu SQ. 2023. Temporal transcriptome reveals that circadian clock is involved in the dynamic regulation of immune response to bacterial infection in Bombyx mori. Insect Sci. 30(1):31–46. doi:10.1111/1744-7917.13043
- Rolff J. 2001. Effects of age and gender on immune function of dragonflies (Odonata, Lestidae) from a wild population. Can J Zool. 79(12):2176–2180. doi:10.1139/z01-190
- Rolff J. 2002. Bateman’s principle and immunity. Proc R Soc Lond B. 269:867–872. doi:10.1098/rspb.2002.1959
- Rolff J, Siva-Jothy MT. 2002. Copulation corrupts immunity: a mechanism for a cost of mating in insects. Proc Natl Acad Sci USA. 99(15):9916–9918. doi:10.1073/pnas.152271999
- Roved J, Westerdahl H, Hasselquist D. 2017. Sex differences in immune responses: Hormonal effects, antagonistic selection, and evolutionary consequences. Hormones Behav. 88:95–105. doi:10.1016/j.yhbeh.2016.11.017
- Sadd BM, Schmid-Hempel P. 2006. Insect immunity shows specificity in protection upon secondary pathogen exposure. Curr Biol. 16(12):1206–1210. doi:10.1016/j.cub.2006.04.047
- Sanborn AF, Phillips PK. 1995. Scaling of sound pressure level and body size in cicadas (Homoptera: Cicadidae; Tibicinidae), Ann. Entomol Soc Amer. 88(4):479–484. doi:10.1093/aesa/88.4.479
- Schwenke RA, Lazzaro BP, Wolfner MF. 2016. Reproduction–immunity trade-offs in insects. Annu Rev Entomol. 61(1):239–256. doi:10.1146/annurev-ento-010715-023924
- Sheldon BC, Verhulst S. 1996. Ecological immunology: costly parasite defences and trade-offs in evolutionary ecology. Trends Ecol Evol. 11(8):317–321. doi:10.1016/0169-5347(96)10039-2
- Simon C, Cooley JR, Karban R, Sota T. 2022. Advances in the evolution and ecology of 13- and 17-year periodical cicadas. Annu Rev Entomol. 67(1):457–482. doi:10.1146/annurev-ento-072121-061108
- Siva-Jothy MT, Moret Y, Rolff J. 2005. Insect immunity: an evolutionary ecology perspective. Adv Insect Physiol. 32:1–48. doi:10.1016/S0065-2806(05)32001-7
- Stoehr AM. 2007. Inter- and intra-sexual variation in immune defence in the cabbage white butterfly, Pieris rapae L. (Lepidoptera: Pieridae). Ecol Entomol. 32(2):188–193. doi:10.1111/j.1365-2311.2007.00855.x
- Stucky BJ. 2015. Infection behavior, life history, and host parasitism rates of Emblemasoma erro (Diptera: Sarcophagidae), an acoustically hunting parasitoid of the cicada Tibicen dorsatus (Hemiptera: Cicadidae). Zool Stud. 54(1):1–7. doi:10.1186/s40555-015-0105-z
- Williams GC. 1957. Pleiotropy, natural selection and the evolution of senescence. Evolution. 11(4):398–411. doi:10.2307/2406060
- Zuk M, Stoehr AM. 2002. Immune defense and host life history. Am Nat. 160(S4):S9–S22. doi:10.1086/342131