Abstract
Terpenoids are a large family of natural products with diversified structures and functions that are widely used in the food, pharmaceutical, cosmetic, and agricultural fields. However, the traditional methods of terpenoids production such as plant extraction and chemical synthesis are inefficient due to the complex processes, high energy consumption, and low yields. With progress in metabolic engineering and synthetic biology, microbial cell factories provide an interesting alternative for the sustainable production of terpenoids. The non-conventional yeast, Yarrowia lipolytica, is a promising host for terpenoid biosynthesis due to its inherent mevalonate pathway, high fluxes of acetyl-CoA and NADPH, and the naturally hydrophobic microenvironment. In this review, we highlight progress in the engineering of Y. lipolytica as terpenoid biomanufacturing factories, describing the different terpenoid biosynthetic pathways and summarizing various metabolic engineering strategies, including progress in genetic manipulation, dynamic regulation, organelle engineering, and terpene synthase variants.
Introduction
Terpenoids are a large group of natural products, differing in both structure and function, and are found in a variety of plants, microbes, and insects [Citation1,Citation2]. Various terpenoids are extensively used in foods, pharmaceuticals, cosmetics, and agricultural products [Citation3]. However, the extraction of terpenoids from plants is challenging because of low yields and purity, as well as the huge consumption of resources. On the other hand, due to their complex structure, the chemical synthesis of terpenoids is inherently difficult and expensive [Citation4]. The production of terpenoids by microbial fermentation using renewable resources therefore provides an interesting alternative strategy to meet the increasing demands for terpenoids.
Model microorganisms such as Escherichia coli and Saccharomyces cerevisiae have been extensively used for terpenoids biosynthesis. However, the low efficiency of the native 2-C-methyl-D-erythritol-4-phosphate (MEP) pathway for isopentenyl diphosphate (IPP) and dimethylallyl diphosphate (DMAPP) supply [Citation5] and the inability to functionally express key plant enzymes such as P450s [Citation6] has limited terpenoids biosynthesis in E. coli. Additionally, the risk of bacterial toxins and acetate inhibition also limits the use of E. coli as a biological host for industrial fermentation [Citation7]. S. cerevisiae is more suitable than E. coli for the production of value-added terpenoids because its mevalonate (MVA) pathway and redox systems offers high potential for terpenoids production and skeleton modification [Citation5,Citation8,Citation9]. However, the use of S. cerevisiae also has disadvantages, including limited secretion, relatively slow growth rates, and the Crabtree Effect, which increases the flux toward fermentation metabolism and ethanol production at the expense of metabolic flux toward acetyl-CoA [Citation10–12].
Recently, Yarrowia lipolytica, an oleaginous yeast, is being adopted as a promising chassis microbe for the production of lipid-derived compounds. In addition, Y. lipolytica is also known for its ability to express and secrete homologous or heterologous enzymes, and is therefore frequently used as a host for the production of recombinant proteins [Citation13,Citation14]. Moreover, with the development of metabolic engineering and synthetic biology tools, it has also been investigated for the biosynthesis of industrial chemicals, including organic acids [Citation15], sugar alcohols [Citation16,Citation17], lipids [Citation18], functional polyunsaturated fatty acids [Citation19], fatty acid-derived fuels, and oleochemicals [Citation20,Citation21]. In addition, Y. lipolytica possesses an active tricarboxylic acid cycle (TCA cycle) and a pentose phosphate pathway (PPP), and it supports high fluxes of acetyl-CoA, NADPH, and the ATP supply by metabolizing a variety of carbon sources [Citation22]. Meanwhile, some strains of Y. lipolytica have been approved by the FDA and obtained the generally recognized as safe (GRAS) status [Citation23], making them suitable hosts for the production of food additives, pharmaceuticals and cosmetics. These characteristics make Y. lipolytica an ideal cell factory in comparison to E. coli and S. cerevisiae for the biosynthesis of some natural products, including terpenoids and polyketides [Citation24–26].
Recent studies compared the terpenoids production in Y. lipolytica and other hosts such as S. cerevisiae [Citation27], summarized several engineering strategies used for the construction of platform strains for the overproduction of mono-, sesqui-, tri-, and tetraterpenoids [Citation28], and clarified the main challenges for terpenoid production in Y. lipolytica [Citation29]. In this review, we focus on progress within the last five years in the metabolic engineering of Y. lipolytica as a microbial cell factory for the biosynthesis of terpenoids. Specifically, the terpenoids biosynthetic pathways and the different engineering strategies for terpenoids biosynthesis are discussed in detail. This review also focuses on perspectives for further improving terpenoids bioproduction in Y. lipolytica through analyzing the achievements, challenges and cutting-edge technologies generated in metabolic engineering and synthetic biology.
Terpenoids biosynthetic pathways
All terpenoids are derived from IPP and its enzymatic tautomer DMAPP, both of which are 5-carbon building blocks. There are two biosynthetic pathways for IPP and DMAPP: the MVA and MEP pathways [Citation30]. Generally, eukaryotes (except for plants, which possess both MVA and MEP pathways), archaea and a few bacteria convert acetyl-CoAs to IPP and DMAPP through the MVA pathway, while prokaryotes produce IPP and DMAPP by the MEP pathway. In Y. lipolytica, IPP and DMAPP are synthesized through the MVA pathway (). Specifically, two acetyl-CoA molecules are condensed into acetoacetyl-CoA in a reaction catalyzed by acetyl-CoA acetyltransferase (ERG10). There are two genes encoding for acetyl-CoA acetyltransferase in Y. lipolytica, which are also termed ACOAAT1 and ACOAAT2. The acetoacetyl-CoA and acetyl-CoA are condensed to 3-hydroxy-3-methylglutaryl-CoA (HMG-CoA) under the facilitation of HMG-CoA synthase (ERG13). Then, HMG-CoA is reduced to mevalonate by NADPH dependent HMG-CoA reductase (HMGR). Subsequently, mevalonate is phosphorylated to form 5-diphosphomevalonate by mevalonate kinase (ERG12) and phosphomevalonate kinase (ERG8) with the consumption of ATPs. Subsequently, 5-diphosphomevalonate is decarboxylated by mevalonate diphosphate decarboxylase (ERG19) to form IPP, which is then isomerized by IPP isomerase (IDI) and converted into DMAPP [Citation27].
Figure 1. The biosynthetic pathways of terpenoids. (A) MVA pathway. (B) The decoration process of representative terpenes. (C) Noncanonical precursors derived from DMAPPs. Metabolites: HMG-CoA, 3-hydroxy-3-methylglutaryl-CoA; IPP, isopentenyl diphosphate; DMAPP, dimethylallyl diphosphate; NPP, neryl diphosphate; GPP, geranyl diphosphate; FPP, farnesyl diphosphate; GGPP, geranylgeranyl diphosphate; CPP, chrysanthemyl diphosphate; LPP, lavandulyl diphosphate; MPP, maconellyl diphosphate; PPP, planococcyl diphosphate. Enzymes: ERG10, acetyl-CoA acetyltransferase; ERG13, HMG-CoA synthase; HMGR, HMG-CoA reductase; ERG12, mevalonate kinase; ERG8, phosphomevalonate kinase; ERG19, mevalonate diphosphate decarboxylase; IDI, IPP isomerase; ERG20, farnesyl pyrophosphate synthetase; ERG9, squalene synthase; ERG1, squalene epoxidase; ERG7, lanosterol synthase; NDPS1, neryl diphosphate synthase; PS, pinene synthase; LS, limonene synthase; LIS, linalool synthase; FS, α-farnesene synthase; BFS, β-farnesene synthase; STS, α-santalene synthase; ADS, amorphadiene synthase; BS, bisabolene synthase; BBS, (−)-α-bisabolol synthase; VS, valencene synthase; CYP706M1, (+)-nootkatone synthase; bAS, β-amyrin synthase; CYP716A12, oleanolic acid synthase; LUS, lupeol synthase; CYP716A180, betulinic acid synthase; DS, dammarenediol-II synthase; PPDS, protopanaxadiol synthase; UGT1, UDP-glucose glucosyltransferase; CCD1, carotenoid cleavage dioxygenase; GGPPS, geranylgeranyl diphosphate synthase; CarRP, phytoene synthase/lycopene cyclase; CarB, phytoene dehydrogenase; CrtW, β-carotene ketolase; CrtZ, β-carotene hydrolase.
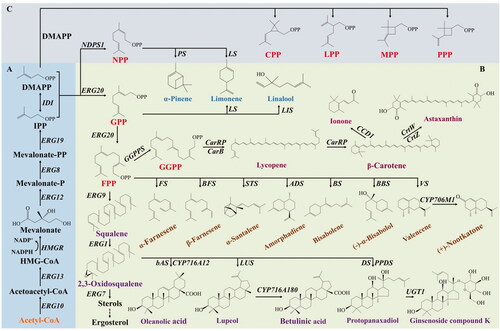
Terpenoids are classified by the number of carbons in the backbone structures and include monoterpenoids, sesquiterpenoids, diterpenoids, triterpenoids, and tetraterpenoids referring to products with 10, 15, 20, 30, and 40 carbons, respectively [Citation31]. The coupling chemistry used to link the isoprenoid precursors determines the structural diversity of natural terpenoids. For example, DMAPP and IPP complexes can generate the 10-carbon block geranyl diphosphate (GPP), and GPP can sequentially condense with other IPP molecules to generate longer carbon scaffolds such as farnesyl diphosphate (FPP), geranylgeranyl diphosphate (GGPP), and geranyl-farnesyl diphosphate (GFPP). Following this, terpene synthases with various functions including glycosyl transfer, transmethylation, or oxidation, decorates the scaffold producing structurally diverse terpenoids. The decorations of representative terpenoids are shown in .
Engineering strategies for terpenoids production
Y. lipolytica can be engineered to synthesize various valuable terpenoids by the introduction of heterologous terpene synthases. In the last few years, researchers have applied a variety of strategies to improve terpenoid(s) production in Y. lipolytica. The main products, metabolic engineering targets, fermentation conditions, and levels are summarized in . The strategies adopted in these publications are described in the following sections.
Table 1. Summary of metabolic engineering strategies for improving terpenoids biosynthesis in Y. lipolytica, and information of representative terpenes with the highest titers reported in E. coli and S. cerevisiae.
Engineering the substrate utilization
Y. lipolytica can utilize different hydrophilic and hydrophobic carbon sources, such as glucose, fructose, mannose, glycerol, fatty acids, and alkanes [Citation76–78], and some of these substrates have been adopted for terpenoids biosynthesis in Y. lipolytica [Citation33,Citation43,Citation45,Citation68]. However, Y. lipolytica does not naturally metabolize xylose, which is an economically interesting feedstock for chemical production because of its abundance in lignocellulose hydrolysates [Citation79]. Several efforts have been made to modify Y. lipolytica in order to allow utilization of xylose as a substrate. Expression of a combination of genes that encode xylose reductase, xylitol dehydrogenase, and xylulose kinase allows the engineered strains to grow efficiently on xylose [Citation80,Citation81]. Similar strategies have been applied to the production of isoprenoids in Y. lipolytica. Yao et al. [Citation35] introduced SsXR and SsXDH from Scheffersomyces stipitis to a Y. lipolytica strain with limonene synthetic modules. After overexpressing endogenous XKS, HMGR, and ERG12, the limonene production from the lignocellulosic feedstock by the recombinant strains increased significantly, reaching 20.57 mg/L after 72 h when 50% of the detoxified hydrolysate was employed. After introducing the xylose utilization pathway, Wu et al. [Citation49] used directed evolution to further improve xylose consumption and eliminate growth instability in the xylose medium. Then, with a series of metabolic engineering strategies to improve the xylose metabolism rate and enhance protopanaxadiol production, the resulting strains demonstrated highly efficient protopanaxadiol production from xylose, with a titer of 300.63 mg/L being achieved through fed-batch fermentation in a 5-L bioreactor ().
Figure 2. Overview of the engineering strategies for terpenoid production in Y. lipolytica. (A) Engineering substrate utilization to minimize production costs. HXK, hexokinase; SsXR, xylose reductase from Scheffersomyces stipitis; SsXDH, xylitol dehydrogenase from Scheffersomyces stipitis; XKS, xylulose kinase. (B) Increasing cytosolic acetyl-CoA supply by introducing heterologous pathways or redirecting native central carbon metabolism. AMPD, AMP deaminase; ICDH, isocitrate dehydrogenase; BbPK, phosphoketolase from Bifidobacterium bifidum; BsPTA, phosphotransacetylase from Bacillus subtilis; ACL, ATP citrate lyase; SeACS, acetyl-CoA synthetase from Salmonella enterica; MFE1, multifunctional β-oxidation enzyme. (C) Overexpressing the genes in MVA pathway and engineering cofactor supply. GND2, 6-phosphogluconate dehydrogenase; MnDH, mannitol dehydrogenase; MAE1, malic enzyme; UGA2, succinate semialdehyde dehydrogenase; IDP2, cytosolic NADP+-specific isocitrate dehydrogenase; AtIPK, isopentenyl phosphate kinase from Arabidopsis thaliana; ScCHK, bifunctional choline kinase/ethanolamine kinase from Saccharomyces cerevisiae. (D) Fine-tuning the expression of key enzymes: combinatorial optimization of P450s-CPRs among species and constructing self‐sufficient P450s by fusion with CPRs; protein engineering to improve the specificity and activity of enzymes; relieving side pathway competition. (E) Modulating hydrophobicity for terpenoid accumulation: increasing intracellular lipids by genetic manipulation or feeding with hydrophobic substrates and in situ trapping with organic overlay. (F) Engineering based on metabolomics analysis: modeling-guided fermentation optimization and metabolic engineering. FBA: flux balance analysis.
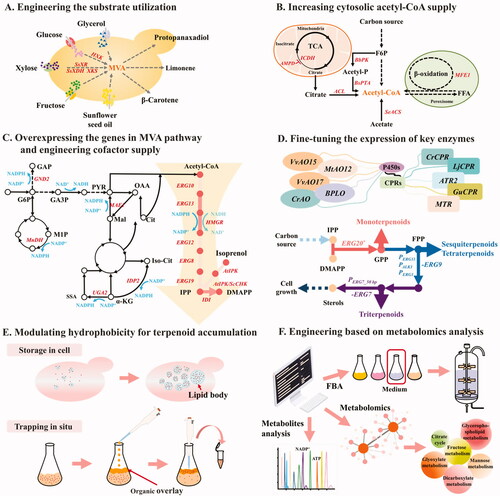
In addition to engineering Y. lipolytica for xylose utilization, researchers have also attempted to improve the glucose utilization capacities of strains. Overexpression of the hexokinase gene (HXK) in Y. lipolytica was found to improve the yield of both biomass and lipids [Citation82]. Qiang et al. [Citation68] investigated the effect of hexokinase on glucose consumption and β-carotene accumulation by overexpressing HXK. The increased hexokinase activity resulted in a higher glucose-6-phosphate content, accelerated glucose utilization rate, and improved β-carotene content by 98%, to 4.63 mg/g DCW ().
Increasing cytosolic acetyl-CoA supply
Adequate acetyl‐CoA supply is crucial for terpenoids production. Y. lipolytica possesses only one acetyl-CoA synthase that is similar to the acetyl-CoA synthetase (ScACS2) of S. cerevisiae, and most of the cytosolic acetyl-CoA is produced by the action of ATP citrate lyase (ACL). The ACL cleaves citrate derived from the mitochondria to oxaloacetate and acetyl-CoA under conditions of nutrient limitation [Citation83]. Different approaches have been used to improve the supply of cytosolic acetyl‐CoA, including introducing heterologous pathways, as well as redirecting the native central carbon metabolism ().
Lu et al. [Citation73] introduced the phosphoketolase-phosphotransacetylase (PK-PTA) pathway to enhance the cytosolic acetyl-CoA supply and re-channel carbon into the target products. After the introduction of the BbPK-BsPTA pathway and modification of the endogenous pathway, the titer of β-ionone was 0.98 g/L in the 3-L fed batch bioreactor, a staggering 280-fold improvement compared with the starting strain. To enhance the carbon flux from pyruvate toward the production of acetyl-CoA, Huang et al. [Citation55] overexpressed the heterogenous acetyl-CoA synthetase gene from Salmonella enterica (SeACS) and the native ATP citrate lyase gene (ACL1) in an HMGR-overexpressing strain. The squalene production of the resulting strain was improved 16.4-fold compared to the control strain. The squalene production ultimately reached 10 mg/g DCW with 10 mM citrate supplemented in shake flasks fermentation.
In Y. lipolytica, continuous efflux of citrate from the mitochondria is required to maintain the level of cytosolic acetyl-CoA. The citrate efflux is the result of the downregulation of isocitrate dehydrogenase, which is localized in mitochondria and catalyzes citrate to isocitrate in an AMP-dependent manner. Zhang et al. [Citation62] overexpressed an AMP deaminase-encoding gene (AMPD) in Y. lipolytica to inhibit the catalytic activity of isocitrate dehydrogenase and enhance the citrate efflux to the cytosol. The lycopene content in the engineered strains was significantly improved, achieving 46-60 mg/g DCW.
As an oleaginous microorganism, Y. lipolytica is capable of accumulating abundant intracellular lipids from acetyl-CoA using a pathway that competes with terpenoids biosynthesis. To direct the carbon flux toward terpenoid production, Jin et al. [Citation54] engineered the acetyl-CoA generation by reinforcing the fatty acid β-oxidation pathway. Combined with other modules such as heterogenous CYP/CPR and a reinforced MVA pathway, overexpression of the multifunctional fatty acid β-oxidation gene (MFE1) further increased squalene accumulation 1.72-fold, ultimately achieving a total titer of 204.89 mg/L for triterpenoids in shake flask fermentation.
Overexpressing MVA pathway genes
The MVA pathway, which converts acetyl-CoA into IPP and its isomer DMAPP represents the core, upstream part of the terpenoid biosynthetic pathway, while the downstream pathway involves decoration, including the conversion of IPP and DMAPP into diverse functional terpenoids [Citation27].
In many organisms, HMG-CoA reductase (HMGR) is the major rate-limiting enzyme of the MVA pathway. Several metabolic engineering strategies are applied to overcome this “bottleneck” and improve the biosynthesis of terpenoids (). HMGR is an endoplasmic reticulum (ER)-resident protein that contains an N-terminal membrane anchor and a C-terminal catalytic domain [Citation84]. Overexpression of intact or N-terminal-truncated HMGR enhances the production of diverse terpenoids [Citation28]. In earlier studies, an N-terminal-truncated HMGR was thought more effective, because the N-terminal truncation can prevent self-degradation of HMGR and make it more stable in the cytoplasm. However, Kildegaard et al. [Citation69] compared the performance between the full-length and truncated HMGR variants and found that the native HMGR is superior for β-carotene production in Y. lipolytica. Overexpression of the full-length HMGR gene in a limonene-producing strain increased the yield of D-limonene and L-limonene by nearly 10-fold, reaching 0.256 mg/L and 0.316 mg/L, respectively [Citation34].
IPP isomerase (IDI), which is responsible for the conversion between IPP and DMAPP and plays a crucial role in the flux distribution of GPP and FPP, is commonly overexpressed to enhance the biosynthesis of terpenoids (). Yang et al. [Citation43] reported that IDI overexpression increased the titer of α-farnesene to 57.08 mg/L, nearly 2.67-fold higher than that produced by the wild-type strain. In addition to overexpressing PvIDI from Pseudescherichia vulneris, Luo et al. [Citation63] introduced a two-step isoprenol utilization pathway (AtIPK-ScCHK) in Y. lipolytica to enhance the supply of IPP and DMAPP. This chimeric AtIPK-ScCHK pathway elevated the total levels of IPP and DMAPP by 15.7-fold compared to the MVA pathway alone, which further improved the lycopene content to approximately 120 mg/g DCW.
In addition to HMGR and IDI, overexpression of other genes in the MVA pathway has also been found to be helpful in directing the MVA flux toward terpenoid production. Zhao et al. [Citation45] found that overexpression of ERG10 (ACOAAT1or ACOAAT2), ERG13, ERG12, ERG8, and ERG19 individually could improve the bisabolene production compared to controls expressing only the bisabolene synthases. Although the effect of ERG13 seems little on boosting bisabolene production, it became more significant for β-carotene production in the context of HMGR being co-overexpressed. Qiang et al. [Citation68] found that overexpressing ERG13 elevated the content of β-carotene by 181% compared to the parent strain which harboring overexpressed tHMGR, and the recombinant strain with two copies of ERG13 performed better, which was 259% higher than parental strain. Therefore, the genes involved in the MVA pathway are usually considered as engineering targets for enhancing the production of terpenoids ().
Fine-tuning the expression of key enzymes
In contrast to the upstream MVA pathway, the downstream terpenoid synthesis pathway is more complex, because it involves heterogenous terpene synthases, various precursors, and different modification steps. In order to improve synthesis efficiency and the yield of products, some strategies have been applied to fine-tune the expression of key enzymes ().
Most natural plant terpene synthases are members of the cytochrome P450 superfamily (P450s or CYPs), that couples with suitable NADPH-cytochrome P450 reductases (CPRs) in order to maintain their catalytic activities. However, there is no P450s-CPRs system associated with terpenoids in Y. lipolytica. Consequently, it is necessary to co-express heterologous P450s and their redox partners for terpenoid production in Y. lipolytica. Although individual expression of P450s and CPRs showed better effects than fused expression of these two proteins in Jin’s studies [Citation54], under certain circumstances, co-expression of individual P450s and CPRs may have little effect on the engineering because of the poor coupling between them [Citation53]. By contrast, constructing self‐sufficient P450s by fuzing P450s and their redox partner CPRs proved a more effective solution. The fusion of P450s and CPRs accelerated the effective electron transfer from NADPH to the P450s and improved the turnover rate of the enzymes [Citation85]. This strategy has been widely used to improve terpenoids production in Y. lipolytica, including protopanaxadiol, valencene, ginsenoside compound K, and oleanolic acid [Citation40,Citation49–51].
In some cases, introduction of an artificial chimeric pathway from different species works more effectively than an intact pathway from the single heterologous species. Therefore, combinatorial optimization by screening alternative steps or isoenzymes among species plays an important role in enhancing the productivity of exogenous pathways [Citation86]. In order to augment the yield of betulinic acid, 25 combinations of P450s and CPRs were screened, and the results showed that the combination of P450s from Betula platyphylla and CPRs from Medicago truncatula contributed the best catalytic effects on the production of betulinic acid (32.33 ± 4.34 mg/L) [Citation54].
In addition, rational protein engineering is also an effective strategy to improve the specificity and activity of enzymes. Some enzymes, such as ERG20, are promiscuous and can catalyze the production of a wide range of natural and non-natural substrates. The enzyme ERG20 functions as both a GPP synthase (GPPS) and FPP synthase (FPPS), which causes difficulty in separating the two synthases during monoterpenoids biosynthesis. To increase the monoterpenoids titer, a double mutant ERG2088W-N119W was employed to improve the GPP pool in Y. lipolytica, resulting in higher linalool or limonene titers significantly compared to the original strain [Citation28,Citation36]. Meanwhile, protein fusion is a convenient strategy for enhancing the local intermediates concentration and enzyme catalytic efficiency, which is helpful to efficiently redirect the metabolic flux to the product [Citation87]. For example, FPP is the immediate precursor in the synthesis of α-farnesene. Co-expression of ERG20 and α-farnesene synthase with a linker is beneficial to improve the catalytic efficiency of α-farnesene synthase on FPP, thereby preventing FPP from entering branched metabolic pathways, such as the biosynthesis of farnesol and squalene [Citation44].
Deleting the key genes in the ergosterol (a component of the cell membrane) biosynthetic pathway leads to serious growth defects [Citation88]. To maintain cell growth and improve the production of target terpenoids, it is feasible to fine-tune the expression of critical genes by promoter engineering. Squalene is a native terpenoid in Y. lipolytica and is the first committed product after FPP in the ergosterol biosynthetic pathway. Squalene synthase is responsible for the synthesis of squalene, encoded by ERG9, which is the key node for competition with FPP for target terpenoid synthesis. To increase the supply of FPP for the biosynthesis of the terpenoids of interest, Kildegaard et al. [Citation69] truncated the native promoter of ERG9 or replaced it with PERG1 or PERG11, which are repressed by ergosterol, and the resulting strains showed a 2-2.5-fold increase in the β-carotene titer compared to the strain with the native ERG9 promoter. Meanwhile, Gao et al. [Citation65] substituted the ERG9 promoter with PALK1, which is repressed by glucose and glycerol [Citation89], and the squalene content decreased by 57.2% in the recombinant strain, resulting in increased lycopene accumulation. Similar tactics have been applied to downregulate the expression of ERG7 (encoding lanosterol synthase) when triterpenoids are regarded as targets such as squalene or 2,3-oxidosqualene [Citation28].
Engineering the cofactor supply
The levels of intracellular cofactors determine the metabolic flux, because cofactors are involved in enzymatic reactions and regulate the chemical equilibrium state. Therefore, the metabolic flux can be directed toward the target biosynthesis by regulating the cellular cofactor levels.
Cellular NADPH, the essential reducing factor for protecting cells from oxidative stress, has been reported as the major rate-limiting factor for fatty acids synthesis in Y. lipolytica [Citation90]. As mentioned above, NADPH dependent HMGR is considered a rate-limiting enzyme and plays an important role in terpenoid biosynthesis. The heterogenous CYP/CPR system for terpenoid biosynthesis also is NADPH dependent. In Y. lipolytica, it has been demonstrated that increasing the supply of NADPH is more effective for the production of betulinic acid than increasing the supply of NADH [Citation54]. Recently, to augment the NADPH pool, a collection of endogenous auxiliary cytosolic NADPH pathways including 6-phosphogluconate dehydrogenase (GND2), mannitol dehydrogenase (MnDH1 or MnDH2), malic enzyme (MAE1), cytosolic NADP+-specific isocitrate dehydrogenase (IDP2), and succinate semialdehyde dehydrogenase (UGA2) has been tested and investigated (). The results showed that mannitol dehydrogenase (MnDH2) demonstrated the best improvement in squalene production [Citation56,Citation91].
In Y. lipolytica, excess NADH will accumulate in cells when oxygen is limited, which leads to the formation of mannitol, and the amount of mannitol can be promoted by fed-batch fermentation [Citation92,Citation93]. Thus, the cofactor NADH may be more abundant than NADPH under certain conditions in Y. lipolytica. Liu et al. [Citation44] have constructed libraries of strains by integrating a chimeric NADH-dependent MVA pathway including EcatoB, ERG13 and NADH dependent BpHMGR into chromosome randomly via non-homologous end-joining (NHEJ) to obtain a mevalonate overproduction strain. In this strain, the α-farnesene synthesis pathway was enhanced by fuzing FS with ERG20 as well as overexpressing ERG12, IDI, ERG8, ERG19 and GPPS, and a titer of 25.55 g/L α-farnesene was achieved in fed-batch fermentation under the conditions of pH 5.5 with a 1.5 vvm air flux.
Modulating hydrophobicity for terpenoid accumulation
Most long-chain terpenoids, such as carotenoids, are fat-soluble due to their inherent hydrophobic characteristics and they are difficult to dissolve in the hydrophilic cytoplasmic environment. Therefore, excess isoprenoids are usually stored within intracellular hydrophobic compartments, such as membranes or lipid bodies [Citation94]. It is well known that Y. lipolytica shows a strong ability to synthesize and accumulate lipids, and its intracellular lipid content can be further increased through metabolic modulation, which provides an ideal environment for terpenoid storage [Citation65,Citation90,Citation95]. It has been previously demonstrated that lycopene accumulation is related with increased lipid content, and that nitrogen limitation is conducive to the accumulation of both lipids and carotenoids [Citation96] (). Based on these studies, Larroude et al. [Citation66] remodeled a lipid-overproducing strain (DGA2, GPD1, Δpox1-6, and Δtgl4) with the carotenoid expression cassette, and the generated strain accumulated higher amounts of β-carotene, 1.93 times more than a wild type strain with the same carotenoid expression cassette.
In Y. lipolytica, intracellular lipids can be synthesized in two ways: the de novo and ex novo pathways. In the de novo synthesis pathway, hydrophilic substrates are required to produce acetyl-CoA, and the acetyl-CoAs are condensed to synthesize acyl-CoA and lipids, while the ex novo synthesis pathway requires the hydrolysis of hydrophobic substrates to produce either fatty acids or glycerol, which are then transported into cells for reassembly as lipids [Citation18]. Lipid synthesis through an ex novo pathway can be activated by feeding Y. lipolytica with exogenous fatty acids. Luo et al. [Citation63] enhanced lipid synthesis separately by overexpressing both ACC1 and DGA1 or supplementing palmitic acid and compared the effects of these two tactics on the yield of lycopene. The addition of palmitic acid to strains resulted in a lycopene triter of 594.6 mg/L, much higher compared with the controls (less than 400 mg/L). The titer of lycopene in ACC1-DGA1-overexpressing strain was 905.9 mg/L, which was elevated more than 2 folds. These results illustrated the importance of providing an intercellular hydrophobic microenviroment for the sequestration and accumulation of long-chain terpenoids.
Unlike carotenoids, some terpenoids are not suitable for intracellular accumulation due to their characteristics, such as cytotoxicity, evaporability, or secretion. To enhance terpenoid production, it is helpful to artificially provide an extracellular hydrophobic environment for product enrichment and storage. A liquid two-phase solvent extraction system that had previously been proved helpful in improving cell viability [Citation97], has been widely used to enrich products and avoid loss from vaporization during fermentation, especially for volatile terpenoids, such as limonene, linalool, and β-ionone (). Cao et al. [Citation32] found that the limonene yield was associated with the amount of dodecane when pyruvic acid was used as the auxiliary carbon source. Meanwhile, it has been shown that an excess of organic overlay could not indefinitely improve the trapping efficiency for products, and the optimum volume of the overlay should be determined according to the actual fermentation process [Citation34]. Recently, Zhang et al. [Citation52] developed a strategy to enhance the product efflux from the cell to the lipophilic solvent by modifying the membrane components. The resultant strain showed an increased unsaturated fatty acid content and an improved production of lupeol with a 33.2-fold increase compared with the original strain.
Engineering based on metabolomics analysis
Genome-scale metabolic models embody significant advantages of systems biology because such approaches can predict globally the biosynthesis of interesting metabolites, facilitating an understanding of the metabolic networks and guiding rational metabolic engineering (). Nambou et al. [Citation59] combined flux balance analysis (FBA) and Plackett-Burman design to screen compounds suitable for lycopene production in Y. lipolytica. An efficient medium was designed based on the variables screened from the factors predicted by FBA and employed for fed-batch cultivation in a 1.5-L fermenter. As a result, a lycopene titer of 242 mg/L was achieved, increased by 92% compared to the FBA-independent media. Zhao et al. [Citation98] investigated the metabolome of Y. lipolytica with expression of the heterologous lycopene biosynthesis cassette PaCrtE-PaCrtB-PaCrtI. The comprehensive analysis showed that lycopene biosynthesis in Y. lipolytica has a significant impact on carbon metabolism, including the metabolism of fructose, mannose, glyoxylate, dicarboxylate, and glycerophospholipid, as well as on the citrate acid cycle. Meanwhile, lycopene production also affected the biosynthesis and metabolism of hydrophobic amino acids such as valine, leucine, and isoleucine.
In later work, researchers [Citation99] analyzed the role of metabolites such as nucleotides and coenzymes in lycopene-producing and control strains using optimized capillary electrophoresis. The concentrations of ATP and NADP+ changed remarkably between the strains, indicating that these two metabolites may be closely linked with lycopene production. Czajka et al. [Citation71] quantified the physiological responses and temporal changes in the metabolism of an engineered Y. lipolytica strain with the production of α-ionone during fermentation. The results of dynamic labeling showed a measurable ATP shortage during the phase of α-ionone production, suggesting that electron transport and oxidative phosphorylation may be bottlenecks in energy supply and strain performance. In conclusion, these findings offer insights into the metabolic dynamics of terpenoid biosynthesis in Y. lipolytica and provides guidance for rational metabolic engineering.
Conclusion and future perspective
Great progress has been made in the metabolic engineering of Y. lipolytica, and several products synthesized in this host have been successfully implemented in commercial production. However, reported terpenoid titers in Y. lipolytica are often in the mg·L−1 range, and gram-scale production has only been reported for a few intensively engineered strains. Meanwhile, the titers of some representative terpenoids, such as limonene, farnesene and squalene, are still much lower in Y. lipolytica than in E. coli or S. cerevisiae (), and also remain well below the concentrations of endogenous molecules such as lipids, citric acid, α-ketoglutarate, succinic acid, and erythritol. This suggests that the potential of Y. lipolytica as a cell factory for terpenoids production has not yet been fully exploited, and there are still challenges that limit the terpenoid biosynthesis in Y. lipolytica. Some of the main reasons may include insufficient genetic tools, lack of basic knowledge and dynamic regulatory elements, as well as underdeveloped compartmentalization engineering in Y. lipolytica.
Firstly, the lack of molecular tools for genetic manipulation limits harnessing the full potential for chemical biosynthesis in Y. lipolytica compared to E. coli and S. cerevisiae. In Y. lipolytica, non-homologous end-joining (NHEJ) is the predominant route for repairing DNA double strand breaks (DSB), while homology-mediated DNA repair is not effective, thereby limiting the application of many synthetic biology tools that depend on homologous recombination (HR) [Citation100]. It has also been shown that disruption of the NHEJ genes, such as KU70, is helpful to increase the HR rate. However, strains with disrupted KU70 show undesirable growth phenotypes and are more sensitive to UV light, which are unfavorable characteristics for the construction of robust host strains [Citation101]. Recently, this problem has been mitigated by the CRISPR/Cas9-based gene editing systems, but more tools are still needed to allow rapid, multiplexed strain engineering in Y. lipolytica [Citation102].
Secondly, the poor basic knowledge of Y. lipolytica metabolism and physiology also limits the rational engineering of strains. For example, the production and regulation of cytosolic acetyl-CoA has not yet been unraveled in detail. This is especially the case for the regulatory mechanisms underlying the multiple acetyl-CoA generation and consumption pathways, as well as for the mechanisms governing acetyl-CoA translocation between subcellular compartments [Citation29]. Although different engineering strategies have been applied to direct the flux of cytosolic acetyl-CoA toward terpenoid biosynthesis, this has not yet been wholly successful. Markham et al. [Citation26] have investigated and demonstrated the utility of the pyruvate bypass pathway for improving the formation of acetyl-CoA and malonyl-CoA, the key precursors of polyketide triacetic acid lactone. However, the strategy has not yet been reported to help increase terpenoid production in Y. lipolytica. Meanwhile, several promising alternative routes for improved cytosolic acetyl-CoA formation in S. cerevisiae have not yet been tested in Y. lipolytica; including the pyruvate oxidase-phosphotransacetylase (PO-PTA) pathway [Citation12], pyruvate decarboxylase-acetylating acetaldehyde dehydrogenase (PDC-A-ALD) pathway and the pyruvate-formate lyase-formate dehydrogenase (PFL-FDH) pathway [Citation103]. Therefore, more specific and detailed knowledge is needed to guide rational metabolic engineering with Y. lipolytica, particularly regarding the ensembling of metabolic fluxes and their regulation.
Thirdly, engineering terpenoids biosynthesis requires a variety of heterologous enzymes. The introduction of these heterologous enzymes can impose a metabolic burden, and will result in flux imbalance, toxic intermediates accumulation, and genetic instability, which in turn limits yield. Dynamic metabolic engineering (DME) is a rapidly developing field that has been applied widely in both E. coli and S. cerevisiae. It allows the cell to autonomously adjust its flux in response to its external and internal metabolic state through the design of genetically encoded metabolic control systems [Citation104]. DME is helpful in improving pathway production and can be further modified and utilized to prevent degenerated and abortive production phenotypes during long-term cultivation and scale-up [Citation105]. However, a dynamic system relies on precise regulation of its elements, such as metabolite-responsive transcription factors, responsive promoters, and riboswitches, which are particularly rare and interesting. Exploring such elements and applying them in Y. lipolytica will provide exciting clues for improving terpenoids production.
Fourthly, compartmentalization into different subcellular organelles can limit interference between different cellular processes and provide suitable environments and sufficient precursors or enzymes for complex biosynthetic pathways. Peroxisomes are the main sites where β-oxidation of fatty acid takes place, creating a pool of acetyl-CoA that can be utilized by heterologous pathways. Recent studies demonstrated the positive effects of peroxisome compartmentalization on monoterpenoid yields in S. cerevisiae, suggesting that the peroxisome is a promising subcellular factory for terpenoid production [Citation39]. Theoretically, peroxisome compartmentalization in Y. lipolytica should offer potential for terpenoids production, as the strains can synthesize and accumulate large amounts of lipids and provide more acetyl-CoA through β-oxidation. However, these findings still need to be verified in Y. lipolytica.
Finally, terpenoids decoration is completed mainly by terpene synthases, which are a large group of related enzymes involved in primary and secondary metabolism [Citation106]. Although many terpene synthases have been identified and characterized, the full repertoire of these enzymes is not yet completely known [Citation2,Citation107]. The range of terpene synthases that are currently used for the construction of biosynthetic pathways in Y. lipolytica is limited to 21 common candidates that lead to poor product variety compared with the reported 80 000 different terpenoids [Citation2], and a low titer in products (). Thus, further mining and directed evolution of terpene synthases from other genomes suitable for use in Y. lipolytica may result in more efficient and diverse biosynthetic pathways. Alternatively, as a result of an irregular coupling reaction, such as cyclobutylation, branching, or cyclopropanation, DMAPP can be converted to neryl diphosphate (NPP) [Citation108], DMAPP and IPP molecules can be fused to produce different carbon skeletons, such as chrysanthemyl diphosphate (CPP), lavandulyl diphosphate (LPP), maconellyl diphosphate (MPP), or planococcyl diphosphate (PPP), resulting in further diversification of the terpene structure [Citation2] (). Therefore identifying specific enzymes that catalyze conversion of these uncommon substrates may also help to further diversify the family of terpenoids.
In conclusion, more background knowledge, genetic tools, engineering strategies and efficient enzymes are needed to exploit the full potential of Y. lipolytica as a platform strain for terpenoids biosynthesis. We envision that the insights from physiological studies of Y. lipolytica, tools developed by synthetic biology and systems biology will provide exciting novel avenues for research into the biosynthesis of terpenoids in Y. lipolytica.
Disclosure statement
No potential conflict of interest was reported by the author(s).
Additional information
Funding
References
- Gershenzon J, Dudareva N. The function of terpene natural products in the natural world. Nat Chem Biol. 2007;3(7):408–414.
- Christianson DW. Structural and chemical biology of terpenoid cyclases. Chem Rev. 2017;117(17):11570–11648.
- Pichersky E, Raguso RA. Why do plants produce so many terpenoid compounds? New Phytol. 2018;220(3):692–702.
- Martin VJJ, Pitera DJ, Withers ST, et al. Engineering a mevalonate pathway in Escherichia coli for production of terpenoids. Nat Biotechnol. 2003;21(7):796–802.
- Wang CL, Liwei M, Park JB, et al. Microbial platform for terpenoid production: Escherichia coli and yeast. Front Microbiol. 2018;9:2460.
- Chang MCY, Eachus RA, Trieu W, et al. Engineering Escherichia coli for production of functionalized terpenoids using plant P450s. Nat Chem Biol. 2007;3(5):274–277.
- De Mey M, De Maeseneire S, Soetaert W, et al. Minimizing acetate formation in E. coli fermentations. J Ind Microbiol Biotechnol. 2007;34(11):689–700.
- Immethun CM, Hoynes-O'Connor AG, Balassy A, et al. Microbial production of isoprenoids enabled by synthetic biology. Front Microbiol. 2013;4:75.
- Moser S, Pichler H. Identifying and engineering the ideal microbial terpenoid production host. Appl Microbiol Biotechnol. 2019;103(14):5501–5516.
- Nielsen J. Synthetic biology for engineering acetyl coenzyme A metabolism in yeast. mBio. 2014;5(6):e02153.
- Palmer CM, Alper HS. Expanding the chemical palette of industrial microbes: metabolic engineering for type III PKS-derived polyketides. Biotechnol J. 2019;14(1):e1700463.
- Dai ZJ, Huang M, Chen Y, et al. Global rewiring of cellular metabolism renders Saccharomyces cerevisiae Crabtree negative. Nat Commun. 2018;9(1):3059.
- Madzak C. Yarrowia lipolytica: recent achievements in heterologous protein expression and pathway engineering. Appl Microbiol Biotechnol. 2015;99(11):4559–4577.
- Vandermies M, Fickers P. Bioreactor-scale strategies for the production of recombinant protein in the yeast Yarrowia lipolytica. Microorganisms. 2019;7(2):40.
- Ma JB, Gu Y, Marsafari M, et al. Synthetic biology, systems biology, and metabolic engineering of Yarrowia lipolytica toward a sustainable biorefinery platform. J Ind Microbiol Biotechnol. 2020;47(9-10):845–862.
- Fickers P, Cheng HR, Sze Ki Lin C. Sugar alcohols and organic acids synthesis in Yarrowia lipolytica: where are we? Microorganisms. 2020;8(4):574.
- Xu YR, Chi P, Bilal M, et al. Biosynthetic strategies to produce xylitol: an economical venture. Appl Microbiol Biotechnol. 2019;103(13):5143–5160.
- Wang JP, Ledesma-Amaro R, Wei YJ, et al. Metabolic engineering for increased lipid accumulation in Yarrowia lipolytica - A Review. Bioresour Technol. 2020;313:123707.
- Kothri M, Mavrommati M, Elazzazy AM, et al. Microbial sources of polyunsaturated fatty acids (PUFAs) and the prospect of organic residues and wastes as growth media for PUFA-producing microorganisms. FEMS Microbiol Lett. 2020;367(5):fnaa028.
- Yan Q, Pfleger BF. Revisiting metabolic engineering strategies for microbial synthesis of oleochemicals. Metab Eng. 2020;58:35–46.
- Spagnuolo M, Yaguchi A, Blenner M. Oleaginous yeast for biofuel and oleochemical production. Curr Opin Biotechnol. 2019;57:73–81.
- Muhammad A, Feng XD, Rasool A, et al. Production of plant natural products through engineered Yarrowia lipolytica. Biotechnol Adv. 2020;43:107555.
- Groenewald M, Boekhout T, Neuveglise C, et al. Yarrowia lipolytica: safety assessment of an oleaginous yeast with a great industrial potential. Crit Rev Microbiol. 2014;40(3):187–206.
- Liu H, Marsafari M, Wang F, et al. Engineering acetyl-CoA metabolic shortcut for eco-friendly production of polyketides triacetic acid lactone in Yarrowia lipolytica. Metab Eng. 2019;56:60–68.
- Lv YK, Marsafari M, Koffas M, et al. Optimizing oleaginous yeast cell factories for flavonoids and hydroxylated flavonoids biosynthesis. ACS Synth Biol. 2019;8(11):2514–2523.
- Markham KA, Palmer CM, Chwatko M, et al. Rewiring Yarrowia lipolytica toward triacetic acid lactone for materials generation. Proc Natl Acad Sci U S A. 2018;115(9):2096–2101.
- Ma YR, Wang KF, Wang WJ, et al. Advances in the metabolic engineering of Yarrowia lipolytica for the production of terpenoids. Bioresour Technol. 2019;281:449–456.
- Arnesen JA, Kildegaard KR, Pastor MC, et al. Yarrowia lipolytica strains engineered for the production of terpenoids. Front Bioeng Biotechnol. 2020;8:945.
- Worland AM, Czajka JJ, Li Y, et al. Biosynthesis of terpene compounds using the non-model yeast Yarrowia lipolytica: grand challenges and a few perspectives. Curr Opin Biotechnol. 2020;64:134–140.
- Vranova E, Coman D, Gruissem W. Network analysis of the MVA and MEP pathways for isoprenoid synthesis. Annu Rev Plant Biol. 2013;64(1):665–700.
- Kirby J, Keasling JD. Metabolic engineering of microorganisms for isoprenoid production. Nat Prod Rep. 2008;25(4):656–661.
- Cao X, Lv YB, Chen J, et al. Metabolic engineering of oleaginous yeast Yarrowia lipolytica for limonene overproduction. Biotechnol Biofuels. 2016;9:214.
- Cheng BQ, Wei LJ, Lv YB, et al. Elevating limonene production in oleaginous yeast Yarrowia lipolytica via genetic engineering of limonene biosynthesis pathway and optimization of medium composition. Biotechnol Bioproc E. 2019;24(3):500–506.
- Pang YR, Zhao YK, Li SL, et al. Engineering the oleaginous yeast Yarrowia lipolytica to produce limonene from waste cooking oil. Biotechnol Biofuels. 2019;12:241.
- Yao F, Liu SC, Wang DN, et al. Engineering oleaginous yeast Yarrowia lipolytica for enhanced limonene production from xylose and lignocellulosic hydrolysate. FEMS Yeast Res. 2020;20(6):foaa046.
- Cao X, Wei LJ, Lin JY, et al. Enhancing linalool production by engineering oleaginous yeast Yarrowia lipolytica. Bioresour Technol. 2017;245(Pt B):1641–1644.
- Wei LJ, Zhong YT, Nie MY, et al. Biosynthesis of α-pinene by genetically engineered Yarrowia lipolytica from low-cost renewable feedstocks. J Agric Food Chem. 2021;69(1):275–285.
- Rolf J, Julsing MK, Rosenthal K, et al. A gram-scale limonene production process with engineered Escherichia coli. Molecules. 2020;25(8):1881.
- Dusseaux S, Wajn WT, Liu YX, et al. Transforming yeast peroxisomes into microfactories for the efficient production of high-value isoprenoids. Proc Natl Acad Sci U S A. 2020;117(50):31789–31799.
- Guo XY, Sun J, Li DS, et al. Heterologous biosynthesis of (+)-nootkatone in unconventional yeast Yarrowia lipolytica. Biochem Eng J. 2018;137:125–131.
- Jia D, Xu S, Sun J, et al. Yarrowia lipolytica construction for heterologous synthesis of α-santalene and fermentation optimization. Appl Microbiol Biotechnol. 2019;103(8):3511–3520.
- Marsafari M, Xu P, Debottlenecking mevalonate pathway for antimalarial drug precursor amorphadiene biosynthesis in Yarrowia lipolytica. Metab Eng Commun. 2020;10:e00121.
- Yang X, Nambou K, Wei LJ, et al. Heterologous production of α-farnesene in metabolically engineered strains of Yarrowia lipolytica. Bioresour Technol. 2016;216:1040–1048.
- Liu YH, Jiang X, Cui ZY, et al. Engineering the oleaginous yeast Yarrowia lipolytica for production of α-farnesene. Biotechnol Biofuels. 2019;12:296.
- Zhao YK, Zhu K, Li J, et al. High-efficiency production of bisabolene from waste cooking oil by metabolically engineered Yarrowia lipolytica. Microb Biotechnol. 2021:1–17.
- Ma YR, Li WJ, Mai J, et al. Engineering Yarrowia lipolytica for sustainable production of the chamomile sesquiterpene (−)-α-bisabolol. Green Chem. 2021;23(2):780–787.
- You SP, Yin QD, Zhang JY, et al. Utilization of biodiesel by-product as substrate for high-production of β-farnesene via relatively balanced mevalonate pathway in Escherichia coli. Bioresour Technol. 2017;243:228–236.
- Meadows AL, Hawkins KM, Tsegaye Y, et al. Rewriting yeast central carbon metabolism for industrial isoprenoid production. Nature. 2016;537(7622):694–697.
- Wu YF, Xu S, Gao X, et al. Enhanced protopanaxadiol production from xylose by engineered Yarrowia lipolytica. Microb Cell Fact. 2019;18(1):83.
- Li DS, Wu YF, Zhang CB, et al. Production of triterpene ginsenoside compound K in the non-conventional yeast Yarrowia lipolytica. J Agric Food Chem. 2019;67(9):2581–2588.
- Li DS, Wu YF, Wei PP, et al. Metabolic engineering of Yarrowia lipolytica for heterologous oleanolic acid production. Cheml Eng Sci. 2020;218:115529.
- Zhang JL, Bai QY, Peng YZ, et al. High production of triterpenoids in Yarrowia lipolytica through manipulation of lipid components. Biotechnol Biofuels. 2020;13:133.
- Sun J, Zhang CB, Nan WH, et al. Glycerol improves heterologous biosynthesis of betulinic acid in engineered Yarrowia lipolytica. Chem Eng Sci. 2019;196:82–90.
- Jin CC, Zhang JL, Song H, et al. Boosting the biosynthesis of betulinic acid and related triterpenoids in Yarrowia lipolytica via multimodular metabolic engineering. Microb Cell Fact. 2019;18(1):77.
- Huang YY, Jian XX, Lv YB, et al. Enhanced squalene biosynthesis in Yarrowia lipolytica based on metabolically engineered acetyl-CoA metabolism. J Biotechnol. 2018;281:106–114.
- Liu H, Wang F, Deng L, et al. Genetic and bioprocess engineering to improve squalene production in Yarrowia lipolytica. Bioresour Technol. 2020;317:123991.
- Meng YH, Shao XX, Wang Y, et al. Extension of cell membrane boosting squalene production in the engineered Escherichia coli. Biotechnol Bioeng. 2020;117(11):3499–3507.
- Han JY, Seo SH, Song JM, et al. High-level recombinant production of squalene using selected Saccharomyces cerevisiae strains. J Ind Microbiol Biotechnol. 2018;45(4):239–251.
- Nambou K, Jian XX, Zhang XK, et al. Flux balance analysis inspired bioprocess upgrading for lycopene production by a metabolically engineered strain of Yarrowia lipolytica. Metabolites. 2015;5(4):794–813.
- Schwartz C, Frogue K, Misa J, et al. Host and pathway engineering for enhanced lycopene biosynthesis in Yarrowia lipolytica. Front Microbiol. 2017;8:2233.
- Liu D, Liu H, Qi H, et al. Constructing yeast chimeric pathways to boost lipophilic terpene synthesis. ACS Synth Biol. 2019;8(4):724–733.
- Zhang XK, Nie MY, Chen J, et al. Multicopy integrants of crt genes and co-expression of AMP deaminase improve lycopene production in Yarrowia lipolytica. J Biotechnol. 2019;289:46–54.
- Luo ZS, Liu N, Lazar Z, et al. Enhancing isoprenoid synthesis in Yarrowia lipolytica by expressing the isopentenol utilization pathway and modulating intracellular hydrophobicity. Metab Eng. 2020;61:344–351.
- Gao SL, Tong YY, Zhu L, et al. Production of β-carotene by expressing a heterologous multifunctional carotene synthase in Yarrowia lipolytica. Biotechnol Lett. 2017;39(6):921–927.
- Gao SL, Tong YY, Zhu L, et al. Iterative integration of multiple-copy pathway genes in Yarrowia lipolytica for heterologous β-carotene production. Metab Eng. 2017;41:192–201.
- Larroude M, Celinska E, Back A, et al. A synthetic biology approach to transform Yarrowia lipolytica into a competitive biotechnological producer of β-carotene. Biotechnol Bioeng. 2018;115(2):464–472.
- Zhang XK, Wang DN, Chen J, et al. Metabolic engineering of β-carotene biosynthesis in Yarrowia lipolytica. Biotechnol Lett. 2020;42(6):945–956.
- Qiang S, Wang J, Xiong XC, et al. Promoting the synthesis of precursor substances by overexpressing hexokinase (Hxk) and hydroxymethylglutaryl-CoA synthase (Erg13) to elevate β-carotene production in engineered Yarrowia lipolytica. Front Microbiol. 2020;11:1346.
- Kildegaard KR, Adiego-Perez B, Belda DD, et al. Engineering of Yarrowia lipolytica for production of astaxanthin. Synth Syst Biotechnol. 2017;2(4):287–294.
- Tramontin LRR, Kildegaard KR, Sudarsan S, et al. Enhancement of astaxanthin biosynthesis in oleaginous yeast Yarrowia lipolytica via microalgal pathway. Microorganisms. 2019;7(10):472.
- Czajka JJ, Kambhampati S, Tang YJ, et al. Application of stable isotope tracing to elucidate metabolic dynamics during Yarrowia lipolytica α-ionone fermentation. iScience. 2020;23(2):100854.
- Czajka JJ, Nathenson JA, Benites VT, et al. Engineering the oleaginous yeast Yarrowia lipolytica to produce the aroma compound β-ionone. Microb Cell Fact. 2018;17(1):136.
- Lu YP, Yang QY, Lin ZL, et al. A modular pathway engineering strategy for the high-level production of β-ionone in Yarrowia lipolytica. Microb Cell Fact. 2020;19(1):49.
- Yang JM, Guo LZ, Biosynthesis of β-carotene in engineered E. coli using the MEP and MVA pathways. Microb Cell Fact. 2014;13:160.
- Sun L, Atkinson CA, Lee YG, et al. High-level β-carotene production from xylose by engineered Saccharomyces cerevisiae without overexpression of a truncated HMG1 (tHMG1). Biotechnol Bioeng. 2020;117(11):3522–3532.
- Spagnuolo M, Hussain MS, Gambill L, et al. Alternative substrate metabolism in Yarrowia lipolytica. Front Microbiol. 2018;9:1077.
- Iida T, Sumita T, Ohta A, et al. The cytochrome P450ALK multigene family of an n-alkane-assimilating yeast, Yarrowia lipolytica: cloning and characterization of genes coding for new CYP52 family members. Yeast. 2000;16(12):1077–1087.
- Mirończuk AM, Rzechonek DA, Biegalska A, et al. A novel strain of Yarrowia lipolytica as a platform for value-added product synthesis from glycerol. Biotechnol Biofuels. 2016;9(1):180.
- Jeffries TW. Emerging technology for fermenting D-xylose. Trends Biotechnol. 1985;3(8):208–212.
- Ledesma-Amaro R, Lazar Z, Rakicka M, et al. Metabolic engineering of Yarrowia lipolytica to produce chemicals and fuels from xylose. Metab Eng. 2016;38:115–124.
- Li HB, Alper HS. Enabling xylose utilization in Yarrowia lipolytica for lipid production. Biotechnol J. 2016;11(9):1230–1240.
- Lazar Z, Dulermo T, Neuveglise C, et al. Hexokinase-a limiting factor in lipid production from fructose in Yarrowia lipolytica. Metab Eng. 2014;26:89–99.
- Dulermo T, Lazar Z, Dulermo R, et al. Analysis of ATP-citrate lyase and malic enzyme mutants of Yarrowia lipolytica points out the importance of mannitol metabolism in fatty acid synthesis. Biochim Biophys Acta. 2015;1851(9):1107–1117.
- Burg JS, Espenshade PJ. Regulation of HMG-CoA reductase in mammals and yeast. Prog Lipid Res. 2011;50(4):403–410.
- Zhao FL, Bai P, Liu T, et al. Optimization of a cytochrome P450 oxidation system for enhancing protopanaxadiol production in Saccharomyces cerevisiae. Biotechnol Bioeng. 2016;113(8):1787–1795.
- Zhang Y, Wang Y, Yao MD, et al. Improved campesterol production in engineered Yarrowia lipolytica strains. Biotechnol Lett. 2017;39(7):1033–1039.
- Zhou YJ, Gao W, Rong QX, et al. Modular pathway engineering of diterpenoid synthases and the mevalonic acid pathway for miltiradiene production. J Am Chem Soc. 2012;134(6):3234–3241.
- Tanaka S, Tani M. Mannosylinositol phosphorylceramides and ergosterol coodinately maintain cell wall integrity in the yeast Saccharomyces cerevisiae. Febs J. 2018;285(13):2405–2427.
- Mori K, Iwama R, Kobayashi S, et al. Transcriptional repression by glycerol of genes involved in the assimilation of n-alkanes and fatty acids in yeast Yarrowia lipolytica. FEMS Yeast Res. 2013;13(2):233–240.
- Qiao KJ, Wasylenko TM, Zhou K, et al. Lipid production in Yarrowia lipolytica is maximized by engineering cytosolic redox metabolism. Nat Biotechnol. 2017;35(2):173–177.
- Liu H, Marsafari M, Deng L, et al. Understanding lipogenesis by dynamically profiling transcriptional activity of lipogenic promoters in Yarrowia lipolytica. Appl Microbiol Biotechnol. 2019;103(7):3167–3179.
- Workman M, Holt P, Thykaer J. Comparing cellular performance of Yarrowia lipolytica during growth on glucose and glycerol in submerged cultivations. AMB Express. 2013;3(1):58.
- Zhang M, Gu L, Cheng C, et al. Recent advances in microbial production of mannitol: utilization of low-cost substrates, strain development and regulation strategies. World J Microbiol Biotechnol. 2018;34(3):41.
- Wei LJ, Kwak S, Liu JJ, et al. Improved squalene production through increasing lipid contents in Saccharomyces cerevisiae. Biotechnol Bioeng. 2018;115(7):1793–1800.
- Xu P, Qiao KJ, Ahn WS, et al. Engineering Yarrowia lipolytica as a platform for synthesis of drop-in transportation fuels and oleochemicals. Proc Natl Acad Sci U S A. 2016;113(39):10848–10853.
- Matthaus F, Ketelhot M, Gatter M, et al. Production of lycopene in the non-carotenoid-producing yeast Yarrowia lipolytica. Appl Environ Microbiol. 2014;80(5):1660–1669.
- Brennan TCR, Turner CD, Kromer JO, et al. Alleviating monoterpene toxicity using a two-phase extractive fermentation for the bioproduction of jet fuel mixtures in Saccharomyces cerevisiae. Biotechnol Bioeng. 2012;109(10):2513–2522.
- Zhao C, Gao Q, Chen J, et al. Metabolomic changes and metabolic responses to expression of heterologous biosynthetic genes for lycopene production in Yarrowia lipolytica. J Biotechnol. 2017;251:174–185.
- Zhao C, Yang Y, Wei LF, et al. Simultaneous determination of intracellular nucleotides and coenzymes in Yarrowia lipolytica producing lipid and lycopene by capillary zone electrophoresis. J Chromatogr A. 2017;1514:120–126.
- Le Guen T, Ragu S, Guirouilh-Barbat J, et al. Role of the double-strand break repair pathway in the maintenance of genomic stability. Mol Cell Oncol. 2015;2(1):e968020.
- Kretzschmar A, Otto C, Holz M, et al. Increased homologous integration frequency in Yarrowia lipolytica strains defective in non-homologous end-joining. Curr Genet. 2013;59(1-2):63–72.
- Abdel-Mawgoud AM, Stephanopoulos G. Improving CRISPR/Cas9-mediated genome editing efficiency in Yarrowia lipolytica using direct tRNA-sgRNA fusions. Metab Eng. 2020;62:106–115.
- van Rossum HM, Kozak BU, Pronk JT, et al. Engineering cytosolic acetyl-coenzyme A supply in Saccharomyces cerevisiae: pathway stoichiometry, free-energy conservation and redox-cofactor balancing. Metab Eng. 2016;36:99–115.
- Hartline CJ, Schmitz AC, Han Y, et al. Dynamic control in metabolic engineering: theories, tools, and applications. Metab Eng. 2021;63:126–140.
- Lv YK, Gu Y, Xu JL, et al. Coupling metabolic addiction with negative autoregulation to improve strain stability and pathway yield. Metab Eng. 2020;61:79–88.
- Keeling CI, Bohlmann J, Genes, enzymes and chemicals of terpenoid diversity in the constitutive and induced defence of conifers against insects and pathogens. New Phytol. 2006;170(4):657–675.
- Sun WT, Qin L, Xue HJ, et al. Novel trends for producing plant triterpenoids in yeast. Crit Rev Biotechnol. 2019;39(5):618–632.
- Zhou F, Pichersky E, More is better: the diversity of terpene metabolism in plants. Curr Opin Plant Biol. 2020;55:1–10.