Abstract
The circular economy is anticipated to bring a disruptive transformation in manufacturing technologies. Robust and industrial scalable microbial strains that can simultaneously assimilate and valorize multiple carbon substrates are highly desirable, as waste bioresources contain substantial amounts of renewable and fermentable carbon, which is diverse. Lignocellulosic biomass (LCB) is identified as an inexhaustible and alternative resource to reduce global dependence on oil. Glucose, xylose, and arabinose are the major monomeric sugars in LCB. However, primary research has focused on the use of glucose. On the other hand, the valorization of pentose sugars, xylose, and arabinose, has been mainly overlooked, despite possible assimilation by vast microbial communities. The present review highlights the research efforts that have explicitly proven the suitability of arabinose as the starting feedstock for producing various chemical building blocks via biological routes. It begins by analyzing the availability of various arabinose-rich biorenewable sources that can serve as potential feedstocks for biorefineries. The subsequent section outlines the current understanding of arabinose metabolism, biochemical routes prevalent in prokaryotic and eukaryotic systems, and possible products that can be derived from this sugar. Further, currently, exemplar products from arabinose, including arabitol, 2,3-butanediol, 1,2,3-butanetriol, ethanol, lactic acid, and xylitol are discussed, which have been produced by native and non-native microbial strains using metabolic engineering and genome editing tools. The final section deals with the challenges and obstacles associated with arabinose-based production, followed by concluding remarks and prospects.
Graphical Abstract
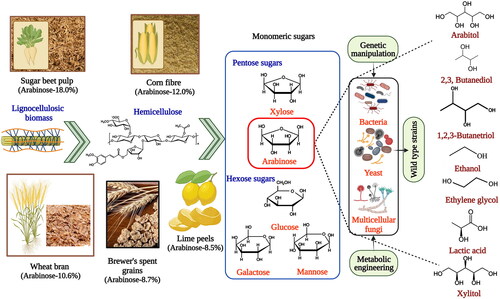
Introduction
We are witnessing an era where sustainable green chemistry is striving to overtake fossil-based synthetic routes for manufacturing widely used chemicals, fuels, and materials. We are still largely dependent on the petrochemical sector to produce industrial chemicals (90%) and energy (80%). The continuous depletion of nonrenewable fossil resources and their adverse environmental impact has necessitated an urgent search for exploiting renewable carbon-rich resources [Citation1]. Biomass is the most promising resource as it enables an inexhaustible storage of organic carbon, which can be transformed into an array of value-added energy and non-energy products depending on the technology platforms used [Citation2,Citation3]. The last two decades have witnessed significant investment and activity in this area. In 2004, the US Department of Energy (DoE) released a list of chemicals obtainable from biomass, which was further revised in 2009 by dropping and adding some chemicals. Various international players (e.g., BASF, DuPont, and Dow) are actively pursuing the development of bio-based production platforms for selected chemicals [Citation4–6]. Presently, the concept of the biorefinery has evolved from merely biomass processing for the production of several value-added marketable products to establishing a circular economy, which requires the development of innovative, energy-efficient, and sustainable decarbonization technologies favoring complete valorization of waste biological resources, with minimal waste generation and greenhouse gas (GHG) capture. This inclusivity is seen in first-generation biorefineries (1G), which use edible feedstocks as primary carbonaceous resources and have already attained prodigious industrial success. Successful examples include the production of ethanol and biodiesel from corn/sugarcane juice, starchy or sugary and vegetable oils, respectively [Citation2,Citation7]. Likewise, several other commercial products are manufactured by 1G biorefineries. For instance, ∼90% of lactic acid (LA) is generated from the fermentation of starchy and sugary feedstocks [Citation8]. The market of bio-based products is continuously growing and is forecasted to reach 97.2 billion USD by 2023 [Citation9]. However, it will be challenging to fulfill this demand using only edible feedstocks, as it poses a threat to feeding the exponentially growing human population. As such, attention has been diverted in the last few decades toward using non-edible biomass, primarily wastes, so as not to compete with food production.
Second-generation (2G) biorefineries mainly use plentiful lignocellulosic biomass (LCB), not part of the human food chain. Despite many efforts, LCB-based production of fuels and chemicals is still at the R&D stage. The sustainability and techno-commercial viability of LCB-based biorefineries could be significantly improved if most of its fraction is valorized cost-effectively and efficiently. The typical composition of LCB is as follows: cellulose (35–50%), hemicellulose (23–32%), and lignin (15–30%) [Citation2,Citation10]. Cellulose is a homopolymer of glucose, while the significant sugars in the hemicellulosic fraction are: xylose, arabinose, galactose, and mannose. Therefore, efficient utilization of different sugars present in LCB is crucial for the commercial success of LCB-based biorefineries. Although glucose, xylose, and arabinose are the major sugars in LCB, most research efforts have been focused on exploiting only the glucose platform.
In contrast, LCB pentose sugars, xylose, and arabinose, have been overlooked as precursor “molecules” and less attention has been given to their valorization [Citation11]. Although there has been an upsurge in research leveraging xylose valorization in recent years, the commercial interest in arabinose is yet to be fully explored. For profitable LCB-based biorefineries, it is imperative to use pentose sugars, which comprise a large fraction of LCB [Citation2]. To the author’s knowledge, this is the first review that exclusively unveils the updated record of research efforts that envisaged arabinose as a potential starting feedstock for producing chemical building blocks via the microbial route. The starting point of this review is a description of the readily available arabinose-rich renewable sources that can play a pivotal role in biorefinery development. Later, the review depicts arabinose metabolism with biochemical routes prevalent in prokaryotic and eukaryotic systems and their possible products. Then, high-value products (arabitol; 2,3-butanediol (BDO); 1,2,3-butanetriol (BTO); ethanol; LA; xylitol) synthesized using arabinose by native and non-native strains with the aid of metabolic engineering and genome editing tools are considered. The review concludes by discussing the challenges and obstacles associated with arabinose-based production and its prospects.
Arabinose and its occurrence
Arabinose (C5H10O5) is an aldopentose sugar and structural isomer to xylose. Unlike most of the monosaccharides that are present in their d-configuration in nature, arabinose is a rare exception as it exists in the l-form. After d-xylose, l-arabinose is the second most widespread pentose sugar in the biosphere. It is a natural component of LCB, the most abundant material on earth (∼200 billion metric tonnes per annum). LCB is an incredible and inexpensive renewable carbonaceous bioresource rich in fermentable sugars, where arabinose is the third most abundant sugar after glucose and xylose. The efficient utilization of LCB-based waste streams is essential for creating improved revenue streams and reducing the waste management burden [Citation10]. The expansion of technologies to utilize arabinose will enable the effective use of LCB, pushing the economics of the bioproduction of chemicals toward favorable outcomes. Arabinose primarily exists as a polymeric component of dietary fibers in several plants, where it is associated with the sugars, xylose, and galactose, as well as other components, such as proteins and uronic acids, to form arabinoxylans and arabinogalactans. l-Arabinose is mainly present in the side chains of hemicellulose and pectin biopolymers as arabinogalactan–protein complexes [Citation12,Citation13]. Arabinose is used in a myriad of applications, including artificial sweeteners, anti-oxidative and blood sugar-reducing agents, precursors for synthesizing amino acids, drug and therapeutic molecules, and raw materials for surfactant production. The abundance of l-arabinose is in the range of 5–10% in most LCB-based feedstocks. A few examples where arabinose is present in significant amounts as a structural carbohydrate include corn fiber (12.0%), wheat bran (10.6%), sugar beet pulp (SBP) (18.0%), brewer’s spent grains (8.7%), and lime peels (8.5%). Further, plant gums such as flaxseed gums of Omega, Foster, Norman, and Arabic also contain 9%, 11%, 14%, and 24% of l-arabinose, respectively [Citation11,Citation14,Citation15].
Sugar beet pulp, a major byproduct from sugar beet-based sugar industries, is one of the richest and inexpensive sources of l-arabinose. Globally, 17 million tonnes of SBP (on a dry weight basis) is generated [Citation14,Citation16]. Alone in the UK, 8 million tonnes of sugar beet is produced annually, and fermentable sugar obtained from SBP is 170 000 tonnes/year [Citation17]. Currently, bioethanol-producing industries pelletize SBP and mix it with molasses, which is sold as animal feed. This is a low-grade application where the potential of SBP is not fully harnessed.
Moreover, high labor costs are associated with SBP-sourced waste management in developed countries. There are brighter prospects with SBP (as well as other arabinose-rich substrates) utilization than the present industrial applications. As a general biorefinery feedstock, the scope of utilizing SBP is potentially enormous. The succeeding section gives a detailed account of arabinose transport and its metabolism in microbial systems, which are the stepping stones for its biotechnological valorization.
Arabinose transport and metabolism
Prokaryotes and eukaryotes use different assimilatory pathways for metabolizing pentose sugars, such as arabinose and xylose, where the former relies on an isomerase pathway and the latter on an oxidoreductive route (). Due to common reaction steps and metabolic intermediates, arabinose and xylose metabolism is well connected, and hence many microorganisms can metabolize both sugars. In fungi, pentose sugar assimilation begins with their uptake through large and poorly characterized sugar transporters [Citation13]. The eukaryotic pathway (fungal) consists of four oxidoreductive steps where two oxidations and two reduction reactions are coupled to NAD(P)+ and NAD(P)H consumption, respectively, and a final phosphorylation step, which makes it distinct from bacterial pathways for the catabolism of l-arabinose. Most genes and their corresponding gene products participating in the l-arabinose catabolic pathway have been characterized in two fungal strains: Aspergillus niger and Trichoderma reesei [Citation12]. The pathway starts with the reduction of l-arabinose to l-arabitol or l-arabinitol by NAD(P)H. This biochemical reaction is catalyzed by l-arabinose reductase/l-arabinitol reductase (AR/LarA) in A. niger and d-xylose reductase (XYL1/XR) in T. reesei. Both enzymes are NADPH specific, and XYL1/XR possesses reductase activity for both d-xylose and l-arabinose. In the next step, l-arabitol is dehydrogenated to l-xylulose, and the transfer of electrons to NAD+ is accomplished by arabitol-4-dehydrogenase (ARDH/LAD1/LadA). This is followed by the isomerization of l-xylulose to d-xylulose via xylitol formation in the next two steps, catalyzed by l-xylulose reductase (LXR3/LxrA) (l-xylulose + NAD(P)H → xylitol + NAD(P)+) and xylitol dehydrogenase (XDH1/XdhA) (xylitol + NAD+ → d-xylulose + NADH). The d-xylulose formed is readily phosphorylated to d-xylulose-5-phosphate using ATP as the phosphate donor and mediated through d-xylulose kinase/d-xylulokinase (LyxK/XK/XKI1/XkiA/XKI1/XKS/XYL3). The d-xylulose-5-phosphate enters the central carbon metabolism (CCM) via the non-oxidative branch of the pentose phosphate pathway (PPP). Xylitol is the first common intermediate of d-xylose and l-arabinose assimilatory pathways, and the last two steps of the fungal l-arabinose pathway (xylitol to d-xylulose-5-phosphate) are shared with the catabolic pathway for d-xylose [Citation12]. In some fungi such as Pichia stipitis, there is another route for l-arabinose entry into the nonoxidative PPP, where d-xylulose is reduced to d-arabinitol, followed by oxidation to d-ribulose and then phosphorylation to ribulose-5-phosphate () [Citation18]. The metabolism of pentose sugars, d-xylose and l-arabinose, takes place by an overall redox neutral oxidoreductive pathway, where dissimilarity of redox factors is a major bottleneck for the efficient uptake of pentose sugars [Citation13,Citation19]. This differential cofactor preference of the enzymes creates redox imbalance and results in a poor arabinose flux [Citation20].
Figure 1. Metabolic pathways for arabinose degradation in prokaryotic and eukaryotic systems [Citation12,Citation13,Citation26,Citation51,Citation76].
![Figure 1. Metabolic pathways for arabinose degradation in prokaryotic and eukaryotic systems [Citation12,Citation13,Citation26,Citation51,Citation76].](/cms/asset/9bc261ef-ea7e-4e30-bb4b-627ec6660508/ibty_a_2270702_f0001_c.jpg)
The bacterial uptake of pentose sugars begins with assimilation via active transporters. The catabolism of arabinose in bacteria is mediated through the following key enzymes (): l-arabinose isomerase (AraA), l-ribulokinase (AraB), and l-ribulose-5-phosphate-4-epimerase (AraD). As soon as l-arabinose enters the cell, it is isomerized into l-ribulose by l-arabinose isomerase. This step is followed by phosphorylation and epimerization to l-ribulose-5-phosphate and d-xylulose-5-phosphate catalyzed by l-ribulokinase and l-ribulose-5-phosphate-4-epimerase, respectively. The bacterial route for arabinose metabolism is redox neutral and circumvents the intrinsic problems of redox imbalances associated with the fungal pathway. The non-oxidative PPP canonically metabolizes the d-xylulose-5-phosphate in both pathways [Citation15,Citation21]. In the case of bacteria and non-arabinose metabolizing yeast strains with XR activity, arabitol production by XR creates a dead end in metabolism, as arabitol is a potent inhibitor of arabinose isomerase [Citation22].
A third pathway is present in archaebacteria where pentose sugars (arabinose and xylose) are metabolized by non-phosphorylating pathways known as Weimberg and Dahms pathways (). The Weimberg pathway is an oxidative route with five consecutive enzymatic steps. It starts with the oxidation of l-arabinose into l-arabino-γ-lactone with NAD(P)+ as a cofactor (step I), followed by hydration into l-arabinonate/L-arabonate (step II). Next, l-arabinonate is transformed into α-ketoglutaric semialdehyde (α-KGSA) with l-2-keto-3-deoxy-arabonate/pentanoate (l-KDA/l-KDP) as intermediate via two consecutive dehydration steps (steps III and IV). The last step (V) of the pathway involves the oxidation of α-ketoglutaric semialdehyde (α-KGSA) to α-ketoglutarate (α-KGA), an intermediate of the TCA cycle. It allows the entry of l-arabinose in the CCM. The pathway steps I to V are catalyzed by: l-arabinose dehydrogenase, l-arabinolactonase, l-arabonate dehydratase, l-KDA dehydratase, and α-KGSA dehydrogenase (KGSADH), respectively. The overall pathway reaction is C5H10O5 (arabinose) + 2NAD+ → C5H6O5 (KGA) + 2NADH + 2H+. The Dahms pathway is an alternative to the Weimberg pathway, where 2-keto-3-deoxy-l-pentanoate (KDP) KDP is split into pyruvate and glycolaldehyde by KDP aldolase (). Pyruvate is part of the CCM, while glycolaldehyde enters the CCM after being reduced to glyoxylate via glycolic acid. The overall equation of the Dahms pathway is C5H10O5 (l-arabinose) + H2O + 3 NAD(P)+ → C2H2O3 (glyoxylate) + C3H4O3 (pyruvate) + 3 NAD(P)H + 3H+. The orthogonality of these non-phosphorylating pathways enables them to function alone or in connection with the CCM to support cellular growth and product formation. The intermediates of these two pathways can be transformed into a range of high-value products via three potential downstream pathways. For example, glycolaldehyde can be reduced to ethylene glycol (EG) while its oxidation yields glycolic acid ().
Figure 2. Non-phosphorylating, Dahms and Weimberg routes for arabinose metabolism [Citation13,Citation58].
![Figure 2. Non-phosphorylating, Dahms and Weimberg routes for arabinose metabolism [Citation13,Citation58].](/cms/asset/8324cb0a-5b76-4270-aa4d-400dbdc9447c/ibty_a_2270702_f0002_c.jpg)
Similarly, l-KDA (2-keto-3-deoxy-l-arabonate) from the Weimberg pathway, after dehydration and decarboxylation, can be converted to 1,4-butanedial, which upon reduction generates 1,4-butanediol (). On the other hand, direct decarboxylation of l-KDA gives 3,4-dihydroxy butyraldehyde, whose reduction results in BTO (). However, most of the research on biotechnological production of these chemicals has been attempted with d-xylose. Supplementary Table 1 summarizes gene encoding for various enzymes involved in arabinose metabolism and products synthesized from it.
Figure 3. Metabolic pathway for production of 1,2,4-butanetriol from arabinose [Citation13,Citation48].
![Figure 3. Metabolic pathway for production of 1,2,4-butanetriol from arabinose [Citation13,Citation48].](/cms/asset/9f176109-5406-4591-8a11-d2d22db613e2/ibty_a_2270702_f0003_c.jpg)
Despite being an omnipresent sugar, the literature on use of arabinose as a feedstock for microbial production of chemical building blocks in biorefineries is scarce. This scarcity is attributed to many factors, including a lack of knowledge on appropriate microbial biochemical pathways for arabinose metabolism. Further, the classical metabolic network harbored by most microbial systems is not adequately designed for arabinose utilization, as pentose metabolism suffers from several limitations, such as the occurrence of carbon catabolite repression (CCR) in the presence of glucose, kinetic efficiencies of biochemical pathways for arabinose metabolism, absence of suitable transporters, etc. [Citation2,Citation13,Citation23]. However, recently Havukainen et al. carried out a detailed characterization of an arabinose transporter in Trichoderma reesei, namely Trire2_104072 [Citation24]. This transporter displayed high affinity for l-arabinose with a Km value of 0.207 ± 0.079 mM and 0.102 ± 0.020 mM at pH 5.5 and –50 mV, when its gene was expressed in oocytes and yeast, respectively. Likewise, an arabinose-proton symporter “PcAraT” was identified in Penicillium chrysogenum, which did not transport d-xylose and d-glucose, showing a K m of 0.13 mM for l-arabinose when expressed in Saccharomyces cerevisiae [Citation25]. Hence, the development of industrially tractable microbial strains capable of using arabinose as the sole carbon source to produce fuels and chemicals has received much attention recently.
Arabinose-based bioproducts
The current state reveals that similar to glucose and d-xylose, l-arabinose can be used as a substrate for the fermentative production of many high-value chemicals. However, research efforts on arabinose-based bioproduction are significantly less. Also, many microbial strains cannot naturally metabolize arabinose, necessitating the requirement to engineer heterologous arabinose metabolic pathways to enable growth and metabolite production [Citation26]. The microbial synthesis of some chemical building blocks from pure and crude arabinose is described in the following section and summarized in . Supplementary Table 2 provides the maximum theoretical yields of different products on arabinose, calculated on the basis of the degree of reduction [Citation27,Citation28].
Table 1. Summary of arabinose-based production of biochemicals.
Arabitol or arabinitol
Arabitol (C5H12O6) is a sugar alcohol and a member of the pentitol family together with ribitol and xylitol. It exists in nature in two forms: d-arabitol and l-arabitol. Due to its low calorific value, arabitol finds major applications in food and pharmaceutical industries as a sweetener like other polyols. d-Arabitol is used for producing chirally pure chemicals having medical properties with applications as immuno-suppressive glycolipids and herbicides [Citation29]. It is also a starting material for synthesizing: propylene, EG, xylitol, arabinoic, and xylonic acids. d-Arabitol is found in low amounts in nature, and its demand is met through chemical and biological production routes. The current industrial d-arabitol manufacturing process involves a two-step hydrogenation of lactones into arabinoic and lyxonic acids using the expensive Raney-Nickel catalyst [Citation30,Citation31]. Microbial d-arabitol production is still in its infancy due to the dearth of knowledge about routes for its biosynthesis and regulatory mechanisms in microbial hosts. The production of l-arabitol from l-arabinose is achieved by a single-step reduction reaction, catalyzed by l-arabinose/arabinitol reductase or xylose reductase (), and l-arabitol is secreted into the growth media under oxygen-limited conditions. Despite this simplified l-arabitol synthesis pathway, l-arabinose remains an underutilized carbon source, and most of the reported work is based on glucose and glycerol-based l-arabitol production involving multi-step complex pathways. Notably, glucose and glycerol have been employed as substrates for the biosynthesis of d-arabitol using osmotolerant yeast strains [Citation31].
Many yeast strains consume l-arabinose under aerobic and fermentative conditions and produce l-arabitol with a small amount of ethanol as the byproduct. Gong et al. examined various yeast strains for the utilization and conversion of: d-xylose, d-xylulose, l-arabinose, and xylitol [Citation32]. Besides l-arabinose, a small amount of l-arabitol was also detected in the culture medium while cultivating yeasts on d-xylose and d-xylulose. With arabinose as substrate, they identified Pachysolen tannophilus and Candida tropicalis as the best l-arabitol producers. These strains accumulated a maximum of 18 and 27 g/L of l-arabitol under aerobic conditions, with yields being 0.59 and 0.64 g/g, respectively. In another report, Dien et al. screened 116 yeast strains for their ability to ferment l-arabinose [Citation33]. They identified Candida auringiensis and Candida succiphila as the most potent l-arabitol accumulators, producing 73 and 81 g/L of l-arabitol using all of the supplied l-arabinose under moderate aeration conditions. In the same year, Saha and Bothast screened 49 arabinose utilizing yeasts and identified that both Candida entomaea and Pichia guilliermondii produced high arabitol titers [Citation34]. After optimizing the pH of the medium and incubation temperature, both the strains produced ≥35 g/L of arabitol from arabinose with a yield of 0.70 g/g. Further, upon subjecting to acid hydrolysate of corn fiber rich in xylose (6.2–6.5 g/L) and arabinose (4.9–5 g/L), C. entomaea and P. guilliermondii produced 2.7 and 2.6 g/L of arabitol, respectively, consuming the latter sugar. This is one of the earliest reports where the real-time arabinose-rich lignocellulosic hydrolysate was tested for arabitol fermentation [Citation34].
Debaryomyces nepalensis, a halotolerant yeast isolated from rotten apples, was reported to metabolize both C6 and C5 sugars [Citation29]. The strain was cultured on glucose, fructose, sucrose, l-arabinose, and glycerol to examine for cell growth and metabolite production. The fungus grew on all these carbon sources except glycerol, with concentrations as high as >80 g/L. Among the different carbon sources used, the maximum cell growth was achieved on glycerol (33 g/L) and l-arabinose (31 g/L), which was much higher than on glucose, sucrose, and fructose (10 g/L). l-Arabitol and ethanol were obtained as major fermentation products. The highest ethanol production (9–10 g/L) was observed on sucrose, glucose, and fructose, while the maximum amount of l-arabitol was accumulated on l-arabinose (22.7 g/L), followed by glucose (7.3 g/L), sucrose (3.7 g/L), and fructose (0.7 g/L) [Citation29].
Bera et al. engineered S. cerevisiae to enable arabinose metabolism by further engineering the d-xylose metabolizing the S. cerevisiae strain 424A(LNH-ST), developed in their previous work [Citation35]. The recombinant 424A(LNH-ST) strain, already harboring xylose reductase and aldol reductase, could reduce l-arabinose to l-arabitol and the missing link to connect arabitol to CCM was completed by the over-expression of two additional enzymes (l-arabitol-4-dehydrogenase and l-xylulose reductase), transforming l-arabitol to d-xylitol via l-xylulose (). The excess unused l-arabitol was transported to the extracellular medium. Both NADH and NADPH-dependent forms of l-xylulose reductase were over-expressed to create S. cerevisiae 424A(LNH-ST)/pLXRNAD-LAD and 424A(LNH-ST)/pLXRNADP-LAD strains. The 424A(LNH-ST)/pLXRNAD-LAD strain, bearing plasmid pLXRNAD-LAD, produced l-arabitol and ethanol with a yield of 33.7 and 42.6%, respectively, during the l-arabinose fermentation. Though both strains accumulated 15 g/L of l-arabitol, l-arabinose assimilation was slower in 424A(LNH-ST)/pLXRNADP-LAD than in 424A(LNH-ST)/pLXRNAD-LAD. The low ethanol production was attributed to the imbalance of redox cofactors. The enzyme activity quantification also showed that the specific activity of the NADPH-dependent l-xylulose reductase was four-fold lower than the NADH-dependent form.
Kordowska-Wiater et al. developed a fermentation process for the biotransformation of arabinose to arabitol using a karyoductant named SP-K7 [Citation36]. This fusant was one of the several karyoductants obtained by fusing the protoplast of S. cerevisiae V30 with the nucleus of P. stipitis CCY 39501 [Citation37]. The work involved optimizing culture conditions using the statistical response surface methodology (RSM) such as the Plackett–Burman and central composite designs. The application of RSM helped identify optimal values for rotation speed, arabinose concentration and temperature, which were 150 rpm, 32.5 g/L, and 28 °C, respectively. The fusant accumulated 16.8 g/L l-arabitol with a yield of 0.52 g/g after 48 h of cultivation, using the optimal parameters.
2,3-Butanediol
BDO (C4H10O2) is a C4 diol with enormous applications in several sectors, including food, chemical, and pharmaceutical industries. BDO is the gateway molecule to many industrially important chemical derivatives, and the total market demand for BDO and allied chemicals is ∼32 million tonnes per annum, worth 43 billion USD [Citation38]. In the last two decades, immense work has been conducted on bio-based BDO production, primarily from glucose. But few research efforts are reported involving pentose sugar-based BDO production. The metabolic pathway for BDO production using pyruvate as the starting material obtained from sugar metabolism is shown in . The pathway starts with the self-condensation of pyruvate to α-acetolactic acid catalyzed by α-acetolactate synthase, followed by either its direct conversion or via diacetyl formation to acetoin by α-acetolactate decarboxylase or diacetyl reductase. Then, acetoin is reduced to BDO by acetoin or BDO reductase in the last step. We found only three reports where arabinose has been used as a carbon source for BDO production. Saha and Bothast isolated a strain of Enterobacter cloacae NRRL B-23289 with the capability to synthesize BDO from arabinose [Citation39]. The work examined the impact of initial l-arabinose concentration, temperature, pH, agitation, various monosaccharides, and multiple sugar mixtures. They reported a BDO titer of 21.7 g/L with a yield of 0.43 g/g from 50 g/L of l-arabinose, and the results were better than that from glucose (18.6 g/L; 0.37 g/g) and xylose (18.9 g/L; 0.38 g/g). The highest BDO concentration of 34.4 g/L was achieved at 100 g/L of l-arabinose; however, the fermentation period was prolonged. In another study, BDO production by five Enterobacter strains from several sugars, including l-arabinose, was studied [Citation40]. The BDO titer of 6.9, 7.3, 7.4, 6.9, and 7.6 g/L was obtained with corresponding yields of 0.31, 0.33, 0.34, 0.38, and 0.36 g/g on l-arabinose by: Enterobacter ludwigii FMCC 204, Enterobacter aerogenes FMCC 9, E. aerogenes FMCC 10, Enterobacter sp. FMCC 208, and Citrobacter freundii FMCC 207, respectively. In a recent study, pure l-arabinose and l-arabinose-rich hydrolysate from SBP pellets were employed for BDO production using E. ludwigii [Citation7]. The l-arabinose-rich hydrolysate from SBP pellets was obtained via dilute acid pretreatment, optimized for solid and acid loadings. The best results were obtained during the fed-batch cultivation, where BDO concentration of 42.9 and 35.5 g/L from pure l-arabinose and SBP hydrolysate was achieved with conversion yields of 0.31 and 0.29 g/g, respectively. The accumulated BDO was recovered using an aqueous two-phase extraction system, and the recovery yield in both cases was ∼97%.
Figure 4. Biochemical route for production of 2,3-butanediol [Citation7].
![Figure 4. Biochemical route for production of 2,3-butanediol [Citation7].](/cms/asset/688bba72-86aa-45cd-9b4b-452c115f273e/ibty_a_2270702_f0004_c.jpg)
1,2,3-Butanetriol
BTO (C4H10O3), a straight-chain C4 triol, is a non-natural molecule with a multitude of applications. BTO and its derivatives are building blocks for manufacturing several pharmaceutical drugs, propellants, and energetic plasticizers. Much work has been done regarding the biotechnological valorization of xylose to BTO [Citation41–47]. However, arabinose has been overlooked so far for BTO synthesis. In a recent isolated report, Escherichia coli was metabolically engineered to generate d-BTO from d-arabinose [Citation48]. The BTO biosynthetic pathway from d- or l-arabinose involves dehydrogenation, dehydration, decarboxylation, and reduction (). To this end, two d-arabinose dehydrogenases (ADG/AraDH), two d-arabinonate dehydratases (AraD/ADT), four 2-keto acid decarboxylases (KivD/KdcA/MdlC/Aro10), and three aldehyde reductases (AdhP/BdhA/ADH2) were evaluated to identify the optimal BTO synthesis pathway. The d-KDA obtained from the Weimberg pathway was decarboxylated to d-3,4-dihydroxybutyraldehyde, followed by its reduction to d-BTO. The best BTO production titer (0.86 g/L) was observed with the recombinant E. coli BT5 strain overexpressing aDG, araD, kivD, and adhP genes sourced from Burkholderia sp., Sulfolobus solfataricus, Lactococcus lactis, and E. coli, respectively [Citation48]. Further, yiaE/ycdW and yjhH/yagE genes encoding glyoxylate reductase and d-KDA aldolase were knocked out to prevent the consumption of carbon flux via the formation of pathway intermediates such as 2-dehydro-3-deoxy-d-pentanoic acid, pyruvate, and glycolaldehyde. The resultant engineered strain BT5ΔyiaEΔycdWΔyagE accumulated 1.13 g/L of BTO after 48 h of cultivation. The application of optimal process parameters (induction temperature: 20 °C; induction OD: 2.0 at 600 nm; IPTG concentration: 2.0 mM; temperature: 37 °C; pH: 7.0; initial l-arabinose concentration: 20 g/L) with the engineered strain resulted in 2.24 g/L of BTO with a yield of 0.11 g/g after 48 h.
Ethanol
Ethanol (C2H6O) is an important biofuel and a platform chemical, being a part of the revised list by US-DoE [Citation4]. It is used as a transportation fuel in pure form or as an additive with gasoline. Its biofuel market is exponentially increasing; presently, 68% of ethanol manufactured is used to make gasoline–ethanol blends. Ethanol also acts as a precursor to manufacture several important commodity chemicals, including ethylene and 1,3-butadiene. Global ethanol production was 100.2 billion liters in 2016 and peaked at 115 billion liters in 2019 [Citation49,Citation50]. It is anticipated that the demand for ethanol will continuously increase because ethanol can play a vital role in the decarbonization of the transport sector, which is crucial to achieving net zero carbon emissions targets. Ethanol is mainly produced by fermentation of hexose sugars using S. cerevisiae as the commercial chassis. However, this yeast cannot metabolize pentose sugars, including l-arabinose and d-xylose. A substantial amount of work has been carried out on xylose-based ethanol production; however, only a handful of studies have reported l-arabinose-based ethanol production [Citation15].
The current state reveals that many yeast and fungal strains metabolize l-arabinose, but its fermentation to ethanol is non-existent. Some d-xylose fermenting engineered S. cerevisiae are available in the market; however, only a few yeast strains can ferment arabinose to ethanol [Citation51]. The first report on ethanol production from arabinose was demonstrated by Richard et al. [Citation52]. They introduced a fungal pathway () into S. cerevisiae, and the recombinant strain carrying all pathway genes (XYL1 encoding xylose reductase, LAD1 encoding arabitol dehydrogenase, LxrA encoding xylulose reductase, XYL2 encoding xylitol dehydrogenase, and XKS encoding xylulokinase) grew very slowly on l-arabinose, generating very little ethanol (0.1 g/L) after 70 h of cultivation. This low performance was attributed to the redox imbalance created in the fungal pathway. The redox neutrality of the pathway was disrupted owing to the consumption of NADPH for the reductive reactions and NADH generation in the oxidation reactions. In an alternative approach, Wisselink et al. metabolically engineered S. cerevisiae by over-expressing the bacterial l-arabinose pathway for efficient ethanol production under anaerobic conditions [Citation20]. The recombinant strain (IMS0001) contained genes for l-arabinose (araA (l-arabinose isomerase), araB (l-ribulokinase), and araD (l-ribulose-5-phosphate 4-epimerase) sourced from Lactobacillus plantarum) and d-xylose pathways (xylA (d-xylose isomerase) and XKS1 (xylulokinase) from Piromyces sp.), as well as for the nonoxidative PPP (TAL1 (transaldolase), TKL1 (transketolase), RPE1 (d-ribulose-5-phosphate 3-epimerase), and RKI1 (ribose-5-phosphate ketol-isomerase)). The pentose sugars (l-arabinose and d-xylose) were assimilated via the PPP. Enhancing the expression of the pathway genes was beneficial for the engineered yeast strain to grow on pentose sugars. The strain was further subjected to extensive evolutionary engineering involving long-term selection in synthetic media containing l-arabinose as the sole carbon source. This strategy yielded an efficient l-arabinose fermenting strain designated as IMS0002, with an enhanced expression of araA, araB, araD, xylA, and XKS1 by several-fold. The anaerobic batch cultivation of the IMS0002 strain on l-arabinose (20 g/L) resulted in an ethanol titer of 8.9 g/L with a conversion yield of 0.43 g/g. The co-fermentation with glucose (20 g/L) and l-arabinose (20 g/L) yielded 17 g/L of ethanol with a similar yield (0.42 g/g). The sugars were wholly consumed in both cases; however, the growth rate of the strain was slower on l-arabinose. Though the reported yields are not very far from the theoretical yield of 0.51 g/g, the titer and production rate need significant improvement for the commercial viability of the process.
The productivity of the bacterial l-arabinose pathway is limited due to the unfavorable thermodynamic properties of l-arabinose isomerase under ambient conditions. The sugar transporters also play an essential role in the l-arabinose-based bioproduction processes. It has been reported that among several native transporters, GAL2 (galactose permease) from S. cerevisiae has the highest affinity for l-arabinose (57 mM) and can help improve the host’s growth rate by transporting l-arabinose. Also, there are other reports on ethanol production (6.0–9.0 g/L) from l-arabinose, where araBAD operon was simultaneously over-expressed with GAL2 (l-arabinose-transporting yeast galactose permease) in S. cerevisiae [Citation53,Citation54]. However, no heterologous sugar transporter has been reported to improve l-arabinose uptake or allow simultaneous consumption of glucose and l-arabinose [Citation15]. Natural l-arabinose assimilators such as Klebsiella oxytoca [Citation55] and E. coli [Citation56] have been engineered for ethanol production. To enable ethanol biosynthesis, pyruvate decarboxylase and alcohol dehydrogenase from Zymomonas mobilis were over-expressed in both organisms. The E. coli strain was further engineered with the deletion of pfl (pyruvate formate lyase) and ldh (lactate dehydrogenase) genes to block pyruvate flux toward acetyl-CoA and lactate formation, respectively, thereby directing more carbon flux toward the product formation. The engineered K. oxytoca and E. coli strains accumulated 27.2 and 44.4 g/L of ethanol with conversion yields of 0.34 and 0.46 g/g, respectively. These results were comparable to that achieved with glucose (46.6 g/L; 0.47 g/g) and xylose (44.8 g/L; 0.46 g/g) using the same strains. The presence of native l-arabinose metabolic pathways in both bacterial strains gave rise to higher ethanol titers than the engineered yeast strains mentioned earlier.
Ethylene glycol
EG (C2H6O2) is a C2 chemical with a hydroxyl group on each carbon atom. EG is a commodity chemical having many applications in our daily life, including: as an industrial solvent and antifreeze agent, as a monomer of polyethylene terephthalate with applications in food and packaging industries, as well as being used in manufacturing paints and resins in the chemical industry. The market size of EG in 2018 was 26 billion USD, and the estimated global production will be 65 million tonnes by 2024 with a compound annual growth rate (CAGR) of 5% [Citation13,Citation57]. Although EG is mainly sourced from fossil fuel-based petrochemical processes, a small fraction of biobased EG is produced as a byproduct of ethanol production from hexose sugars. However, research efforts on pentose sugars such as l-arabinose and d-xylose-based EG manufacture are scarce. Pereira et al. [Citation58] designed recombinant E. coli K12 strains for EG production from pentose sugars. The notable feature of the work is that both l- and d-arabinose were utilized for EG production using the engineered E. coli K12 strains (). For d-arabinose-based EG production, the pathway involves the isomerization of d-arabinose into d-ribulose by FucI (l-fucose isomerase), followed by phosphorylation to d-ribulose-1-phosphate by FucK (l-fuculose kinase). d-Ribulose-1-phosphate is then cleaved into glycolaldehyde and dihydroxyacetone phosphate (DHAP) catalyzed by l-fuculose-1-phosphate aldolase (FucA). DHAP enters the CCM while glycolaldehyde can either be reduced to EG by FucO (l-1,2-propanediol oxidoreductase) or oxidized to glycolic acid by AldA (glycolaldehyde dehydrogenase) (). The recombinant E. coli K-12 MG1655 (DE3) ΔrecAΔendA strain synthesized 1.8 g/L of EG from d-arabinose along with glycolate, confirming the presence of the pathway. Like EG, glycolic acid is a commercial product with diverse applications in the textile and cosmetic industries, and the market is forecasted to reach 415 million USD by 2024 [Citation59].
Figure 5. Biosynthetic pathways for production of ethylene glycol and glycolic acid from d- and l-arabinose [Citation13,Citation58].
![Figure 5. Biosynthetic pathways for production of ethylene glycol and glycolic acid from d- and l-arabinose [Citation13,Citation58].](/cms/asset/0dd2bc18-0f01-4e64-876a-875589c30f47/ibty_a_2270702_f0005_c.jpg)
The titer and yield of EG were further improved (3.4 g/L, 0.35 g/g) with the deletion of AldA to prevent glycolic acid formation and divert more carbon flux toward EG formation at the glycolaldehyde node (). In the l-arabinose-based EG production pathway, the first two steps include the isomerization of l-arabinose to l-xylulose via l-ribulose, catalyzed by l-arabinose isomerase (AraA) and d-tagatose-3-epimerase (DTE). Then, l-xylulose is transformed to d-ribulose-1-phosphate by l-rhamnulose kinase (RhaB), followed by splitting into glycolaldehyde and DHAP by rhamnulose-1-phosphate aldolase (RhaD). The genes fucI, fucK, fucA, and fucO belong to fuc-regulon used for utilization of l-fucose [Citation60], while rhaB and rhaD are part of the rhamnose regulon, involved in metabolizing l-rhamnose, a methylpentose [Citation61]. To enable EG production from l-arabinose, AraB (l-ribulokinase) was deleted to avoid the degradation of l-ribulose via the PPP, along with the introduction of plasmids overexpressing DTE, RhaB, RhaD, and FucO. During the batch cultivation, the recombinant strain exhibited a significant lag phase, followed by an active growth phase resulting in an EG titer of 20 g/L with a conversion yield of 0.38 g/g. This study successfully demonstrated the conversion of both l- and d-arabinose into EG, but the EG production captures only 40% of the total carbon supplied from pentose sugars. Therefore, future work should be directed toward valorizing the remaining three carbon atoms into products derived from the CCM.
Lactic acid
LA (C3H6O3) is an optically active compound that exists in both d and l stereoisomers. The presence of a hydroxyl and carboxylic acid group makes it a highly versatile molecule. It can be transformed into a variety of other high-value products, including propylene glycol, lactate esters, acrylic acid, propylene oxide, propanoic acid, acetaldehyde, 2,3-pentanedione, dilactide, and poly-LA [Citation3,Citation62]. LA is also one of the most important biomass-derived platform chemicals, according to the list prepared by the US DoE [Citation4]. LA has significant applications in the production of food, chemical, cosmetic, and pharmaceutical industries. The worldwide demand for LA is continuously growing, and its market value is expected to increase from 2.6 billion USD in 2018 to 8.7 billion USD in 2025, with a CAGR of 18.7% [Citation63]. The current state reveals several reports on LA bioproduction from edible and non-edible feedstocks. In fact, manufacturing via the fermentative route meets a large amount of current LA demand (∼90%). Despite these advances, the research work on l-arabinose-based LA synthesis is sparse. Most of the LA-producing organisms generate the l-isomer naturally. The earliest evidence of arabinose transformation to d-LA was reported by Okano and colleagues, who engineered Lactobacillus plantarum to produce d-LA via homolactic acid fermentation [Citation64]. The l-lactate dehydrogenase (ldhL1) was deleted in the wild-type L. plantarum, and the resultant mutant strain L. plantarum ΔldhL1 generated only d-LA (8.7 g/L) with acetic acid (6.3 g/L) as a byproduct from l-arabinose (19.6 g/L). Both products were generated from the phosphoketolase (PK) pathway: l-arabinose + 2ADP + 2Pi → LA + acetic acid + 2ATP + 2H2O. Notably, the PPP can produce LA as the only product from a pentose with a maximum theoretical yield of 1.67 mol LA/mol pentose. Thus, to divert the carbon flux from PK to PPP for shifting hetero- to homo-LA fermentation, the endogenous PK gene (xpk1) in L. plantarum was replaced with the heterologous transketolase gene (tkt) from Lactococcus lactis IL 1403 strain. The recombinant strain L. plantarum ΔldhL1-xpk1::tkt accumulated 38.6 g/L of d-LA after 27 h of fermentation with a small amount of acetic acid (0.4 g/L). The yield, purity, and productivity of d-LA were 0.82 g/g, 99.9%, and 1.43 g/L h, respectively. Thus, the diversion of carbon flux from PK to PPP significantly enhanced cell growth and titer, yield and productivity (TYP) of d-LA. In recent work, a recombinant Aspergillus brasiliensis was designed with the heterologous overexpression of the ldhA (l-lactate dehydrogenase) gene from Rhizopus oryzae [Citation65]. The recombinant strain synthesized l-LA from glucose, d-xylose, and l-arabinose. The strain synthesized l-LA very slowly and accumulated 9.0 g/L after 264 h of cultivation on l-arabinose.
Xylitol
Like ethanol and LA, xylitol (C5H12O5) is a top platform chemical. It has been enlisted in the old and revised lists of platform chemicals obtainable from biomass prepared by the US DoE [Citation4]. Xylitol has a similar sweetness to table sugar but has a lower calorific value (1/3rd of table sugar) and acts as an alternative sweetener. Xylitol has several health benefits and finds applications in the food, pharmaceutical, and chemical industries. It can be transformed into numerous high-value products, such as xylaric acid, glycerol, LA, EG, propylene glycol, and hydroxyfuran derivatives, being a platform chemical. In 2016, the estimated global market for xylitol was 921 million USD, predicted to reach 1.37 billion USD by 2025 [Citation66,Citation67]. Xylitol can be obtained via chemical and biochemical routes, but both routes use biomass as the source of xylose, a native substrate for xylitol. Xylitol is a reduced compound obtained by reducing the aldehyde group in xylose. The chemical method uses H2 to reduce xylose in the presence of the Raney Ni catalyst, while electrons for this reduction reaction are provided by NAD(P)H mediated via xylose reductase [Citation2]. Besides xylose, xylitol can also be produced from other sugars, such as l-arabinose, as it is one of the intermediates of arabinose metabolism ().
Sakakibara et al. designed an E. coli strain for xylitol production from l-arabinose [Citation68]. The synthetic ATX pathway involved the isomerization of l-arabinose to l-ribulose by l-arabinose isomerase (AraA), followed by further conversion of l-ribulose to l-xylulose by d-psicose 3-epimerase (DPE sourced from Rhizobium radiobacter) and l-xylulose to xylitol by xylulose reductase (Lxr sourced from Ambrosio monospora) with NADH as oxidant. The genes encoding these three enzymes were cloned behind the araBAD promoter of the pBAD18-Kan vector in tandem, and the resulting plasmid was designated as pATX210. The l-xylulose in the ATX pathway has an advantage over d-xylulose, which is directly phosphorylated to d-xylulose-5-phoshate and funneled into PPP. The plasmid carrying the ATX pathway was introduced into wild-type E. coli AB707, and the transformant AB707 (pATX210) consumed all the supplied l-arabinose without accumulation of xylitol. No xylitol formation was observed as the transformant contained the araBAD operon encoding l-arabinose isomerase (AraA), l-ribulokinase (AraB), and l-ribulose 5-phosphate 4-epimerase (AraD). It consumed l-ribulose produced from l-arabinose, thereby reducing arabinose carbon flux toward xylitol. Moreover, the presence of l-xylulokinase (LxyK) further diverted l-xylulose away from being reduced to xylitol through phosphorylation. These bottlenecks were circumvented by deleting the araBAD operon and LyxK gene from the transformant to create the recombinant E. coli ZUC99 (pATX210) strain. This strain assimilated 4.2 g/L from 10.5 g/L of l-arabinose and produced 2.6 g/L xylitol in 36 h, yielding 0.62 g/g. Approximately, 38% of the consumed l-arabinose was not converted to xylitol, indicating the presence of other endogenous kinases and epimerases causing the degradation of l-arabinose. Notably, a continuous supply of NADH is required for smooth xylitol production, and glycerol, being a reduced carbon source, can generate more reducing equivalents like NADH than pentose and hexose sugars. Therefore, the co-fermentation of l-arabinose (10.5 g/L) and glycerol (11.8 g/L) with the engineered strain in shake flasks, resulted in the xylitol titer and yield of 9.7 g/L and 0.92 g/g after 36 h with complete consumption of l-arabinose. Further, in the co-fermentation (20.1 g/L l-arabinose + 10.3 g/L glycerol) in bioreactors, 15.2 g/L of l-arabinose was consumed with an accumulation of 14.5 g/L xylitol in 30 h [Citation68].
A similar approach was taken by Dhar et al. for xylitol production from d-xylose and l-arabinose by Corynebacterium glutamicum [Citation69]. They overexpressed AraA (from E. coli), DPE (from Agrobacterium tumefaciens), Lxr (from Mycobacterium smegmatis), and XR (from Rhodotorula mucilaginosa) enzymes in C. glutamicum to achieve xylitol production from l-arabinose and d-xylose, respectively. The expression of XR and the synthetic ara A-dpe-lxr operon were controlled under the IPTG-inducible Ptac promoter of plasmid pVWEx1 in C. glutamicum. The recombinant C. glutamicum (Cg-ax3) strain accumulated 31.0 g/L of xylitol in 12 h during the fed-batch cultivation using a minimal medium containing glucose, d-xylose, and l-arabinose, each at 10 g/L. However, the same strain on the SAPL2 medium based on acid-pretreated liquor of sorghum containing 10 g/L of glucose, d-xylose, and l-arabinose each during fed-batch culture produced 27.0 g/L xylitol.
Other bio-based products
Besides these extensively studied bio-based products, butanol and isopropanol production has been demonstrated from arabinose using the wild Clostridium beijerinckii strain BGS1. The strain accumulated 7.8 g/L and 1.6 g/L of butanol and isopropanol, respectively, when fed with 60 g/L arabinose [Citation70]. Using the PPP, the genus Clostridium is long known to valorize arabinose to acetone–butanol–ethanol (ABE). In 1983, Clostridium acetobutylicum ATCC 824 was evaluated for solvent (ABE) production, which assimilated 57 g/L arabinose and attained a concentration of 10.5, 4.5, and 1.5 g/L for butanol, acetone, and ethanol, respectively [Citation71]. Qureshi et al. reported the production of 15.2 g/L ABE by Clostridium acetobutylicum P260 within 72 h consuming 37 g/L arabinose when corn fiber xylan was used as the substrate during fermentation [Citation72]. Later, in 2010, Liu et al. attempted to valorize wheat bran hydrolysate containing 21.3, 17.4, and 10.6 g/L glucose, xylose, and arabinose, respectively, using Clostridium beijerinckii ATCC 55025, which simultaneously consumed both hexose and pentose sugar effectively [Citation73]. Within 72 h, the strain accumulated 11.8 g/L ABE, with yield and productivity being 0.32 g/g and 0.16 g/L h, respectively. Likewise, when a batch study was conducted with Clostridium saccharoperbutylacetonicum N1-4, using arabinose (20 g/L) with LA (5 g/L) as co-substrate, 8.3 g/L solvents were produced with >85% product being butanol [Citation74]. Later, the robustness of the same strain was evaluated for ABE fermentation with arabinose as the sole substrate. It was found that 24.8 g/L ABE was formed with acetone, butanol and ethanol titers being 8.3, 15.6, and 0.9 g/L, respectively [Citation75].
In this section, the three isolated reports where arabinose biovalorization led to attaining appreciable titers of >40 g/L for products like BDO, arabitol, and ethanol () indicate that arabinose is an equally good feedstock for biorefinery development. In this context, rigorous efforts need to be expedited to realize the full potential of arabinose. The relevance of these endeavors becomes more important when disruptive transformation is anticipated from lignocellulosic feedstocks toward the commercial production of bio-based fuels and chemicals, of which arabinose is also an integral part.
Challenges associated with biovalorization of arabinose
Almost two decades of research have been dedicated to developing microbial strains capable of efficient pentose sugar fermentation. However, only a handful of chemicals have been produced from l-arabinose, and the product spectrum needs to be broadened [Citation25]. Despite being a promising carbon source, in most cases, the fermentation rate and TYP metrics of l-arabinose-based fermentation processes are still lower than glucose-based bioprocesses. Approaches, where arabinose metabolism has been extensively addressed, have failed to provide clear solutions. Thus, premeditating the future of l-arabinose-based bioproduction processes is challenging, as genetic and metabolic engineering efforts have been limited. Several roadblocks must be addressed to improve the performance of l-arabinose metabolizing cell factories to make them industrially appealing for commercial applications or for creating arabinose-based biorefineries. For instance, no pretreatment strategy currently can selectively fractionate arabinose from other sugars in the LCB. As a result, though arabinose-rich hydrolysates can be obtained, they may contain other sugars like xylose, glucose, mannose, or galactose. Preferential uptake of arabinose is often restricted owing to the CCR phenomenon. Furthermore, l-arabinose assimilating microbes are sparse, and an intensive search is required for microbial systems having an active and fast l-arabinose metabolism. Hence, the application of l-arabinose as a feedstock of choice for the fermentation industry is jeopardized owing to the absence of metabolic routes connecting arabinose to CCM, the reservoir for precursors to various high-value commodity chemicals.
In industrial fermentation, the complexity of substrate metabolism plays a crucial role in the overall performance of the bioprocesses, which is governed by metabolic regulation and the availability of efficient transporters. Sugar transport across the cell membrane is the first step in metabolism and is a strong driving force for overall bioprocess productivity. The information on l-arabinose transporters is very limited, and in fact, there is no reported transporter for enhancing l-arabinose uptake or simultaneous assimilation of l-arabinose and glucose [Citation15]. l-Arabinose uptake is inefficient even in natural l-arabinose metabolizing strains [Citation32]. The assimilation of non-natively metabolizable substrates is far more complicated than producing target end products. Since the metabolic modules, i.e., reactions and enzymes involved in glucose, d-xylose, and l-arabinose, are different, the introduction of an l-arabinose-metabolizing pathway into a host designed for only glucose metabolism might not always give the desired outcomes [Citation22]. It has been hypothesized that in the case of non-native sugar metabolism, routine metabolic engineering strategies such as the constitutive expression and/or deletion of metabolic genes are insufficient because the inherent regulatory and transcriptional limitations of the host are surfaced once metabolic limitations are eliminated, leading to carbon and amino acid starvation signals. Therefore, activating certain regulatory, metabolic, and signaling genes further downstream from the CCM is essential to achieve enhanced growth rates of the engineered chassis [Citation25].
Another challenge in l-arabinose pathway engineering is to balance the use of redox cofactors, especially with the fungal pathway owing to different enzyme preferences for NAD(P)H and NAD(P)+ [Citation25]. However, instead of a bottleneck, this redox cofactor imbalance can be a driving force for enhanced production of target metabolites whose synthesis complements the deficiency of the redox factor. Despite various efforts to optimize strains and bioprocesses using l-arabinose, this underperformance indicates some other reasons that need to be decoded. For instance, the kinetic and thermodynamic properties of different enzymes involved in l-arabinose-based biomanufacturing need a detailed investigation, as kinetic inefficiencies of enzymes and unfavorable thermodynamics of pathway reactions may obstruct the effective functioning of the l-arabinose metabolic pathway. These challenges necessitate rewiring the classical cellular metabolic networks of hosts for optimal functioning of the l-arabinose metabolism.
Further, it requires designing and constructing bespoke metabolic networks by introducing heterologous pathways in the hosts from other organisms and/or creating novel synthetic routes by applying current computational tools. The new pathways should be orthogonal to the CCM to ensure cellular homeostasis. For example, plugging in non-phosphorylating pathways orthogonal to the hosts’ natural metabolic networks can make them imperceptive to endogenous genetic and metabolic regulations. This pathway orthogonality will allow the partitioning of the carbon flux toward biomass and product formation with a tunable dynamic control to the desired goal.
Certainly, no single solution addresses all the challenges encountered in the l-arabinose-based fermentation processes. The advanced fields of modern biotechnology, including high-throughput “omics” technologies, such as comparative and functional genomics, transcriptomics, proteomics, metabolomics, systems, and synthetic biology, including metabolic, enzyme, and evolutionary engineering, can be employed to elicit more definitive results for fixing the challenges associated with l-arabinose metabolism. The use of these modern techniques, individually or in combination, can lead to the construction of optimized engineered strains capable of efficiently metabolizing l-arabinose with powerful native/synthetic control elements for fine-tuning of the expression level of multiple genes and strongly regulated metabolic networks. Robust cell factories that can efficiently assimilate arabinose in a mixed sugar hydrolysate can also be developed using traditional, rational, and inverse engineering approaches, such as adaptive laboratory evolution and directed evolution. Finally, extensive research on bioprocess optimization and intensification for l-arabinose-based cell factories should be carried out to sustainably develop commercially viable fermentation processes for the bioproduction of value-added commodity chemicals. However, it is equally essential that while harnessing the potential of arabinose from renewable resources like LCB, major cost-contributing steps and environmental hotspots of the process are identified. Advanced tools like techno-economic analysis (TEA) and life-cycle assessment (LCA) can easily address their impacts and guide the researchers to make subsequent process improvements, which critically affect the commercial and environmental sustainability during scale-up operations. Under the present scenario wherein the "low carbon economy" is one of the thrust areas, the role of TEA and LCA cannot be ignored.
Concluding remarks and prospects
The demand for bio-based products is anticipated to increase due to concerns related to global warming, economic stability and emphasis on clean production, green and environmentally friendly manufacturing, and decarbonization of the chemical industry. For the economical production of chemical building blocks from LCB, converting other abundant sugars such as l-arabinose besides glucose is a pre-requisite. Despite its abundance, l-arabinose has not been considered a critical bioresource. Thus, a pressing need has arisen to utilize arabinose from the LCB-based production of chemical building blocks through genome editing, metabolic pathways engineering, and evolutionary engineering in many other strains to fulfill the current global demand at a competitive price. The microbial strains lacking the ability to efficiently metabolize hexose and pentose sugars simultaneously is the major bottleneck for the cost-effective production of biochemicals from 2G feedstocks; thus, expanding the substrate range is key to creating commercially viable 2G biorefineries. Research on the use of pentoses, specifically l-arabinose, in the fermentation industry is still in its infancy. Also, inadequate research efforts on designing efficient l-arabinose metabolizing cell factories for industrial biotechnology applications could be a considerable cause for the inefficient production of metabolites from l-arabinose compared to glucose and xylose. The literature is full of reports often committed to addressing isolated problems of l-arabinose metabolism, and a combined approach to focus on applications of l-arabinose for industrial manufacturing is missing. Thus, further intensive efforts need to be made in this direction, which might cater to a scalable yield. The authors hope this article can give an impetus to accelerate essential research on developing sustainable biotechnology targeting using l-arabinose-rich LCB feedstock.
Author contributions
VK: conceptualization, writing-original draft, reviewing and editing, and project management; DA: conceptualization, writing-original draft, reviewing, and editing; RRB, MAI, SJ, VB, and VS: writing-original draft, reviewing, and editing; VKT and NSS: reviewing and editing.
Supplemental Material
Download MS Word (23.9 KB)Disclosure statement
No potential conflict of interest was reported by the authors.
Additional information
Funding
References
- Takkellapati S, Li T, Gonzalez MA. An overview of biorefinery-derived platform chemicals from a cellulose and hemicellulose biorefinery. Clean Technol Environ Policy. 2018;20:1615–1630. doi: 10.1007/s10098-018-1568-5.
- Narisetty V, Cox R, Bommareddy R, et al. Valorisation of xylose to renewable fuels and chemicals, an essential step in augmenting the commercial viability of lignocellulosic biorefineries. Sustain Energy Fuels. 2021;6:29–65. doi: 10.1039/d1se00927c.
- Agrawal D, Kumar V. Recent progress on sugarcane-bagasse based lactic acid production: technical advancements, potential and limitations. Ind Crops Prod. 2023;193:116132. doi: 10.1016/j.indcrop.2022.116132.
- Bozell JJ, Petersen GR. Technology development for the production of biobased products from biorefinery carbohydrates—the US Department of Energy’s "Top 10" revisited. Green Chem. 2010;12:539–554. doi: 10.1039/b922014c.
- Maity SK. Opportunities, recent trends and challenges of integrated biorefinery: part I. Renew Sustain Energy Rev. 2015;43:1427–1445. doi: 10.1016/j.rser.2014.11.092.
- Maity SK. Opportunities, recent trends and challenges of integrated biorefinery: part II. Renew Sustain Energy Rev. 2015;43:1446–1466. doi: 10.1016/j.rser.2014.08.075.
- Narisetty V, Narisetty S, Jacob S, et al. Biological production and recovery of 2,3-butanediol using arabinose from sugar beet pulp by Enterobacter ludwigii. Renew Energy. 2022;191:394–404. doi: 10.1016/j.renene.2022.04.024.
- Alexandri M, Schneider R, Mehlmann K, et al. Recent advances in d-lactic acid production from renewable resources: case studies on agro-industrial waste streams. Food Technol Biotechnol. 2019;57:293–304. doi: 10.17113/ftb.57.03.19.6023.
- Ko YS, Kim JW, Lee JA, et al. Tools and strategies of systems metabolic engineering for the development of microbial cell factories for chemical production. Chem Soc Rev. 2020;49:4615–4636. doi: 10.1039/d0cs00155d.
- Liu Y, Tang Y, Gao H, et al. Challenges and future perspectives of promising biotechnologies for lignocellulosic biorefinery. Molecules. 2021;26:5411. doi: 10.3390/molecules26175411.
- Fehér C. Novel approaches for biotechnological production and application of l-arabinose. J Carbohydr Chem. 2018;37:251–284. doi: 10.1080/07328303.2018.1491049.
- Metz B, Mojzita D, Herold S, et al. A novel l-xylulose reductase essential for l-arabinose catabolism in Trichoderma reesei. Biochemistry. 2013;52:2453–2460. doi: 10.1021/bi301583u.
- Francois JM, Alkim C, Morin N. Engineering microbial pathways for production of bio-based chemicals from lignocellulosic sugars: current status and perspectives. Biotechnol Biofuels. 2020;13:118. doi: 10.1186/s13068-020-01744-6.
- Joanna B, Michal B, Piotr D, et al. Sugar beet pulp as a source of valuable biotechnological products. In: Holban AM, Grumezescu AM, editors. Advances in biotechnology for food industry. Academic Press; 2018. p. 359–392. doi: 10.1016/B978-0-12-811443-8.00013-X.
- Ye S, Kim JW, Kim SR. Metabolic engineering for improved fermentation of l-arabinose. J Microbiol Biotechnol. 2019;29:339–346. doi: 10.4014/jmb.1812.12015.
- Finkenstadt VL. A review on the complete utilization of the sugarbeet. Sugar Tech. 2014;16:339–346. doi: 10.1007/s12355-013-0285-y.
- Ioannidou SM, Pateraki C, Ladakis D, et al. Sustainable production of bio-based chemicals and polymers via integrated biomass refining and bioprocessing in a circular bioeconomy context. Bioresour Technol. 2020;307:123093. doi: 10.1016/j.biortech.2020.123093.
- Jin YS, Cruz J, Jeffries TW. Xylitol production by a Pichia stipitis d-xylulokinase mutant. Appl Microbiol Biotechnol. 2005;68:42–45. doi: 10.1007/s00253-004-1854-5.
- Young E, Lee SM, Alper H. Optimizing pentose utilization in yeast: the need for novel tools and approaches. Biotechnol Biofuels. 2010;3:24 doi: 10.1186/1754-6834-3-24.
- Van Maris AJ, Abbott DA, Bellissimi E, et al. Alcoholic fermentation of carbon sources in biomass hydrolysates by Saccharomyces cerevisiae: current status. Anton Van Leeuwenhoek. 2006;90:391–418. doi: 10.1007/s10482-006-9085-7.
- Wisselink HW, Toirkens MJ, del Rosario Franco Berriel M, et al. Engineering of Saccharomyces cerevisiae for efficient anaerobic alcoholic fermentation of l-arabinose. Appl Environ Microbiol. 2007;73:4881–4891. doi: 10.1128/AEM.00177-07.
- Zhang Z, Su B, Wu M, et al. Strategies for eliminating l-arabinitol in the bioconversion of xylitol. Process Biochem. 2016;51:1964–1972. doi: 10.1016/j.procbio.2016.08.027.
- Kwak S, Jo JH, Yun EJ, et al. Production of biofuels and chemicals from xylose using native and engineered yeast strains. Biotechnol Adv. 2019;37:271–283. doi: 10.1016/j.biotechadv.2018.12.003.
- Havukainen S, Pujol-Giménez J, Valkonen M, et al. Functional characterization of a highly specific l-arabinose transporter from Trichoderma reesei. Microb Cell Fact. 2021;20:1–20. doi: 10.1186/s12934-021-01666-4.
- Bracher JM, Verhoeven MD, Wisselink HW, et al. The Penicillium chrysogenum transporter PcAraT enables high-affinity, glucose-insensitive l-arabinose transport in Saccharomyces cerevisiae. Biotechnol Biofuels. 2018;11:63. doi: 10.1186/s13068-018-1047-6.
- Endalur Gopinarayanan V, Nair NU. Pentose metabolism in Saccharomyces cerevisiae: the need to engineer global regulatory systems. Biotechnol J. 2019;14:e1800364. doi: 10.1002/biot.201800364.
- Dugar D, Stephanopoulos G. Relative potential of biosynthetic pathways for biofuels and bio-based products. Nat Biotechnol. 2011;29:1074–1078. doi: 10.1038/nbt.2055.
- Kumar V, Park S. Potential and limitations of Klebsiella pneumoniae as a microbial cell factory utilizing glycerol as the carbon source. Biotechnol Adv. 2018;36:150–167. doi: 10.1016/j.biotechadv.2017.10.004.
- Kumdam H, Narayana Murthy S, Gummadi SN. Production of ethanol and arabitol by Debaryomyces nepalensis: influence of process parameters. AMB Express. 2013;3:23. doi: 10.1186/2191-0855-3-23.
- Kordowska-Wiater M. Production of arabitol by yeasts: current status and future prospects. J Appl Microbiol. 2015;119:303–314. doi: 10.1111/jam.12807.
- Ravikumar Y, Razack SA, Ponpandian LN, et al. Microbial hosts for production of d-arabitol: current state-of-art and future prospects. Trends Food Sci Technol. 2022;120:100–110. doi: 10.1016/j.tifs.2021.12.029.
- Gong CS, Claypool TA, McCracken LD, et al. Conversion of pentoses by yeasts. Biotechnol Bioeng. 1983;25:85–102. doi: 10.1002/bit.260250108.
- Dien BS, Kurtzman CP, Saha BC, et al. Screening for l-arabinose fermenting yeasts. Appl Biochem Biotechnol. 1996;57–58:233–242. doi: 10.1007/BF02941704.
- Saha BC, Bothast RJ. Production of l-arabitol from l-arabinose by Candida entomaea and Pichia guilliermondii. Appl Microbiol Biotechnol. 1996;45:299–306. doi: 10.1007/s002530050687.
- Bera AK, Sedlak M, Khan A, et al. Establishment of l-arabinose fermentation in glucose/xylose co-fermenting recombinant Saccharomyces cerevisiae 424A (LNH-ST) by genetic engineering. Appl Microbiol Biotechnol. 2010;87:1803–1811. doi: 10.1007/s00253-010-2609-0.
- Kordowska-Wiater M, Kubik-Komar A, Targoński Z. Optimization of arabitol production by karyoductant SP-K 7 of Saccharomyces cerevisiae V 30 and Pichia stipitis CCY 39501 using response surface methodology. Pol J Microbiol. 2012;61:291–297. doi: 10.33073/pjm-2012-039.
- Kordowska-Wiater M, Targoński Z. Application of Saccharomyces cerevisiae and Pichia stipitis karyoductants to the production of ethanol from xylose. Acta Microbiol Pol. 2004;50:291–299.
- Amraoui Y, Prabhu AA, Narisetty V, et al. Enhanced 2,3-butanediol production by mutant Enterobacter ludwigii using Brewers’ spent grain hydrolysate: process optimization for a pragmatic biorefinery loom. Chem Eng J. 2022;427:130851. doi: 10.1016/j.cej.2021.130851.
- Saha BC, Bothast RJ. Production of 2,3-butanediol by newly isolated Enterobacter cloacae. Appl Microbiol Biotechnol. 1999;52:321–326. doi: 10.1007/s002530051526.
- Liakou V, Pateraki C, Palaiogeorgou AM, et al. Valorisation of fruit and vegetable waste from open markets for the production of 2,3-butanediol. Food Bioprod Process. 2018;108:27–36. doi: 10.1016/j.fbp.2017.10.004.
- San K-Y, Bennett GN, Berríos-Rivera SJ, et al. Metabolic engineering through cofactor manipulation and its effects on metabolic flux redistribution in Escherichia coli. Metab Eng. 2002;4:182–192. doi: 10.1006/mben.2001.0220.
- Niu W, Molefe MN, Frost JW. Microbial synthesis of the energetic material precursor 1,2,4-butanetriol. J Am Chem Soc. 2003;125:12998–12999. doi: 10.1021/ja036391+.
- Abdel-Ghany SE, Day I, Heuberger AL, et al. Metabolic engineering of Arabidopsis for butanetriol production using bacterial genes. Metab Eng. 2013;20:109–120. doi: 10.1016/j.ymben.2013.10.003.
- Zhang N, Wang J, Zhang Y, et al. Metabolic pathway optimization for biosynthesis of 1,2,4-butanetriol from xylose by engineered Escherichia coli. Enzyme Microb Technol. 2016;93–94:51–58. doi: 10.1016/j.enzmictec.2016.07.007.
- Sun L, Yang F, Sun H, et al. Synthetic pathway optimization for improved 1,2,4-butanetriol production. J Ind Microbiol Biotechnol. 2016;43:67–78. doi: 10.1007/s10295-015-1693-7.
- Jing P, Cao X, Lu X, et al. Modification of an engineered Escherichia coli by a combined strategy of deleting branch pathway, fine-tuning xylose isomerase expression, and substituting decarboxylase to improve 1,2,4-butanetriol production. J Biosci Bioeng. 2018;126:547–552. doi: 10.1016/j.jbiosc.2018.05.019.
- Bamba T, Yukawa T, Guirimand G, et al. Production of 1,2,4-butanetriol from xylose by Saccharomyces cerevisiae through Fe metabolic engineering. Metab Eng. 2019;56:17–27. doi: 10.1016/j.ymben.2019.08.012.
- Wang J, Chen Q, Wang X, et al. The biosynthesis of d-1,2,4-butanetriol from d-arabinose with an engineered Escherichia coli. Front Bioeng Biotechnol. 2022;10:844517. doi: 10.3389/fbioe.2022.844517.
- Bušić A, Marđetko N, Kundas S, et al. Bioethanol production from renewable raw materials and its separation and purification: a review. Food Technol Biotechnol. 2018;56:289–311. doi: 10.17113/ftb.56.03.18.5546.
- Mendiburu AZ, Lauermann CH, Hayashi TC, et al. Ethanol as a renewable biofuel: combustion characteristics and application in engines. Energy. 2022;257:124688. doi: 10.1016/j.energy.2022.124688.
- Caballero A, Ramos JL. Enhancing ethanol yields through d-xylose and l-arabinose co-fermentation after construction of a novel high efficient l-arabinose-fermenting Saccharomyces cerevisiae strain. Microbiology. 2017;163:442–452. doi: 10.1099/mic.0.000437.
- Richard P, Verho R, Putkonen M, et al. Production of ethanol from l-arabinose by Saccharomyces cerevisiae containing a fungal l-arabinose pathway. FEMS Yeast Res. 2003;3:185–189. doi: 10.1016/S1567-1356(02)00184-8.
- Becker J, Boles E. A modified Saccharomyces cerevisiae strain that consumes l-arabinose and produces ethanol. Appl Environ Microbiol. 2003;69:4144–4150. doi: 10.1128/AEM.69.7.4144-4150.2003.
- Wiedemann B, Boles E. Codon-optimized bacterial genes improve l-arabinose fermentation in recombinant Saccharomyces cerevisiae. Appl Environ Microbiol. 2008;74:2043–2050. doi: 10.1128/AEM.02395-07.
- Bothast RJ, Saha BC, Flosenzier AV, et al. Fermentation of l-arabinose, d-xylose and d-glucose by ethanologenic recombinant Klebsiella oxytoca strain P2. Biotechnol Lett. 1994;16:401–406. doi: 10.1007/BF00245060.
- Dien BS, Hespell RB, Wyckoff HA, et al. Fermentation of hexose and pentose sugars using a novel ethanologenic Escherichia coli strain. Enzyme Microb Technol. 1998;23:366–371. doi: 10.1016/S0141-0229(98)00064-7.
- Bourgade B, Humphreys CM, Millard J, et al. Design, analysis, and implementation of a novel biochemical pathway for ethylene glycol production in Clostridium autoethanogenum. ACS Synth Biol. 2022;11:1790–1800. doi: 10.1021/acssynbio.1c00624.
- Pereira B, Li ZJ, De Mey M, et al. Efficient utilization of pentoses for bioproduction of the renewable two-carbon compounds ethylene glycol and glycolate. Metab Eng. 2016;34:80–87. doi: 10.1016/j.ymben.2015.12.004.
- Grand View Research. Glycolic acid market size worth $415.0 million by 2024; 2016 [cited 2023 Mar 6]. Available from: https://www.grandviewresearch.com/press-release/global-glycolic-acid-market
- Chen YM, Zhu Y, Lin ECC. The organization of the fuc regulon specifying l-fucose dissimilation in Escherichia coli K12 as determined by gene cloning. Mol Gen Genet. 1987;210:331–337. doi: 10.1007/BF00325702.
- Moralejo P, Egan SM, Hidalgo E, et al. Sequencing and characterization of a gene cluster encoding the enzymes for l-rhamnose metabolism in Escherichia coli. J Bacteriol. 1993;175:5585–5594. doi: 10.1128/jb.175.17.5585-5594.1993.
- Abedi E, Hashemi SMB. Lactic acid production – producing microorganisms and substrates sources—state of art. Heliyon. 2020;6:e04974. doi: 10.1016/j.heliyon.2020.e04974.
- Augustiniene E, Valanciene E, Matulis P, et al. Bioproduction of l- and d-lactic acids: advances and trends in microbial strain application and engineering. Crit Rev Biotechnol. 2022;42:342–360. doi: 10.1080/07388551.2021.1940088.
- Okano K, Yoshida S, Tanaka T, et al. Homo-d-lactic acid fermentation from arabinose by redirection of the phosphoketolase pathway to the pentose phosphate pathway in l-lactate dehydrogenase gene-deficient Lactobacillus plantarum. Appl Environ Microbiol. 2009;75:5175–5178. doi: 10.1128/AEM.00573-09.
- Liaud N, Rosso MN, Fabre N, et al. l-Lactic acid production by Aspergillus brasiliensis overexpressing the heterologous ldha gene from Rhizopus oryzae. Microb Cell Fact. 2015;14:1–9. doi: 10.1186/s12934-015-0249-x.
- Arcaño YD, García ODV, Mandelli D, et al. Xylitol: a review on the progress and challenges of its production by chemical route. Catal Today. 2020;344:2–14. doi: 10.1016/j.cattod.2018.07.060.
- Umai D, Kayalvizhi R, Kumar V, et al. Xylitol: bioproduction and applications—a review. Front Sustain. 2022;3:826190. doi: 10.3389/frsus.2022.826190.
- Sakakibara Y, Saha BC, Taylor P. Microbial production of xylitol from l-arabinose by metabolically engineered Escherichia coli. J Biosci Bioeng. 2009;107:506–511. doi: 10.1016/j.jbiosc.2008.12.017.
- Dhar KS, Wendisch VF, Nampoothiri KM. Engineering of Corynebacterium glutamicum for xylitol production from lignocellulosic pentose sugars. J Biotechnol. 2016;230:63–71. doi: 10.1016/j.jbiotec.2016.05.011.
- Zhang C, Li T, He J. Characterization and genome analysis of a butanol–isopropanol-producing Clostridium beijerinckii strain BGS1. Biotechnol Biofuels. 2018;11:280. doi: 10.1186/s13068-018-1274-x.
- Ounine K, Petitdemange H, Raval G, et al. Acetone-butanol production from pentoses by Clostridium acetobutylicum. Biotechnol Lett. 1983;5:605–610. doi: 10.1007/BF00130841.
- Qureshi N, Li XL, Hughes S, et al. Butanol production from corn fiber xylan using Clostridium acetobutylicum. Biotechnol Prog. 2006;22:673–680. doi: 10.1021/bp050360w.
- Liu Z, Ying Y, Li F, et al. Butanol production by Clostridium beijerinckii ATCC 55025 from wheat bran. J Ind Microbiol Biotechnol. 2010;37:495–501. doi: 10.1007/s10295-010-0695-8.
- Yoshida T, Tashiro Y, Sonomoto K. Novel high butanol production from lactic acid and pentose by Clostridium saccharoperbutylacetonicum. J Biosci Bioeng. 2012;114:526–530. doi: 10.1016/j.jbiosc.2012.06.001.
- Yao D, Dong S, Wang P, et al. Robustness of Clostridium saccharoperbutylacetonicum for acetone–butanol–ethanol production: effects of lignocellulosic sugars and inhibitors. Fuel. 2017;208:549–557. doi: 10.1016/j.fuel.2017.07.004.
- Spagnuolo M, Shabbir Hussain M, Gambill L, et al. Alternative substrate metabolism in Yarrowia lipolytica. Front Microbiol. 2018;9:1077. doi: 10.3389/fmicb.2018.01077.