ABSTRACT
Plasma triglyceride (TG) levels show a clear daily rhythm, however, thus far it is still unknown whether this rhythm results from a daily rhythm in TG production, TG uptake or both. Previous studies have shown that feeding activity affects plasma TG concentrations, but it is not clear how the daily rhythm in feeding activity affects plasma TG concentrations. In the present study, we measured plasma TG concentrations and TG secretion rates in rats at 6 Zeitgeber times to investigate whether plasma TG concentrations and TG secretion show a daily rhythm. We found that plasma TG concentrations and TG secretion show a significant day/night rhythm. Next, we removed the daily rhythm in feeding behavior by introducing a 6-meals-a-day (6M) feeding schedule to investigate whether the daily rhythm in feeding behavior is necessary to maintain the daily rhythm in TG secretion. We found that the day/night rhythm in TG secretion was abolished under 6M feeding conditions. Hepatic apolipoprotein B (ApoB) and microsomal TG transfer protein (Mttp), which are both involved in TG secretion, also lost their daily rhythmicity under 6M feeding conditions. Together, these results indicate that: (1) the daily rhythm in TG secretion contributes to the formation of a day/night rhythm in plasma TG levels and (2) a daily feeding rhythm is essential for maintaining the daily rhythm in TG secretion.
Introduction
Hypertriglyceridemia is an independent risk factor for type 2 diabetes, metabolic syndrome and cardiovascular disease (Grundy Citation2004; Miller et al. Citation2011; Pramono and Harbuwono Citation2015). Plasma triglyceride (TG) concentrations are mainly determined by the balance between TG secretion and TG clearance. Disruption of the balance between TG secretion and TG clearance may result in hypertriglyceridemia (Reiner Citation2017). In order to understand the pathophysiology of hypertriglyceridemia, it is necessary to investigate the molecular and biological mechanisms of plasma TG metabolism – namely, the regulation of TG secretion and clearance.
It is well known that plasma TG concentrations exhibit daily rhythmicity in both humans and laboratory rodents (Mondola et al. Citation1995; Pan and Hussain Citation2007; Rudic et al. Citation2004; Van Oostrom et al. Citation2000; Yasumoto et al. Citation2016). Recently, Moran-Ramos et al. (Citation2017) showed that (part of) this rhythmicity might be caused by a day/night rhythm in TG clearance, however, until now, it remains unknown whether also a daily rhythm in TG secretion exists. Very low density lipoprotein (VLDL) is a lipoprotein mainly secreted by the liver, it transports hepatic lipids, mainly TGs, to plasma for use in peripheral tissues. As VLDL’s mostly carry TGs in plasma, the rate of VLDL secretion is a major determinant of the TG secretion rate. Lipin 1 (Chen et al. Citation2015), stearoyl-CoA desaturase 1(Scd1) (Lam et al. Citation2007), apolipoprotein B (ApoB) (Olofsson and Boren Citation2005; Olofsson et al. Citation2000), microsomal TG transfer protein (MTTP) (Jamil et al. Citation1995) and ADP-ribosylation factor 1 (ARF-1) (Asp et al. Citation2000) are other important enzymes involved in VLDL-TG assembly and secretion. A number of studies have shown that these genes exhibit a clear daily rhythm in expression (Gorne et al. Citation2015; Liu et al. Citation2013; Pan and Hussain Citation2007; Pan and Hussain Citation2009; Pan et al. Citation2013; Pan et al. Citation2010). Thus, we hypothesized that also TG secretion would show a daily rhythm.
Shift work in the long term leads to an increased risk for obesity and the development of other features of the metabolic syndrome (Itani et al. Citation2017; Schernhammer and Thompson Citation2011; Zhao et al. Citation2012). Previous studies (Itani et al. Citation2017; Lu et al. Citation2017; Romon et al. Citation1992) have shown that plasma TG levels are increased in shift workers. Feeding during the usual period of inactivity and sleep is thought to be one of the important contributors to the metabolic disorders of shift workers (Guerrero-Vargas et al. Citation2018; Mukherji et al. Citation2015; Salgado-Delgado et al. Citation2010). Indeed, some studies (Al-Naimi et al. Citation2004; Sopowski et al. Citation2001) found that postprandial plasma TG levels increased to a larger extent when eating in the inactive period. Thus, eating at the wrong time of day may be a possible contributing factor to the higher plasma TG levels in shift workers. Other studies (Lanza-Jacoby et al. Citation1986; Pan and Hussain Citation2007; Pan et al. Citation2013; Shamsi et al. Citation2014; Yasumoto et al. Citation2016) have shown that indeed changes in the daily rhythm of feeding behavior shifted or abolished the daily rhythm of plasma TG. Therefore, the daily feeding rhythm is considered to be an important Zeitgeber for the maintenance of the daily rhythm of plasma TG levels – namely, for maintaining the daily rhythm of TG production and/or clearance.
In the present study, we measured the TG secretion rate by the Tyloxapol method (Bruinstroop et al. Citation2012) at different time points during the light/dark cycle, to investigate whether TG secretion shows a daily rhythm. Next, we abolished the daily feeding rhythm by introducing a 6-meals-a-day feeding schedule (Kalsbeek and Strubbe Citation1998) to investigate whether a daily rhythm in feeding behavior is necessary to maintain the daily rhythm in plasma TG levels and TG secretion.
Materials and methods
Animals
Experiments were performed with 10 week-old adult male Wistar rats (Charles River, Germany). Animals were maintained under a12h/12h light/dark cycle (lights on at 07:00) and constant conditions of temperature (21 ± 2°C) and humidity (60 ± 5%). Food and water was available ad libitum (AL), unless stated otherwise. All experiments were approved by the animal care committee of the Royal Netherlands Academy of Arts and Science.
Surgery procedures
After 7 days of habituation, rats were anesthetized with a 0.14 ml/100g body weight (BW) i.m. Ketamine (4.57%)–Xylazine (0.23%)–Atropin (0.0014%) mixture (Ketamine: 45.7mg/ml (Eurovet Animal Health, Bladel, The Netherlands); Xylazine: 2.3 mg/ml (Bayer Health Care, Mijdrecht, The Netherlands); Atropin: 0.014 mg/ml (Pharmachemine B.V., Haarlem, The Netherlands). Post-operative care was provided via a subcutaneous injection of Buprecare (AUV, Cuijk, The Netherland; 0.005 mg/100 g BW) after the operation.
A permanent catheter was placed into the right jugular vein for blood sampling. The catheter was tunneled subcutaneously and exited the skin at the top of the head. It was filled with a mixture of polyvinylpyrrolidone (PVP; Sigma-Aldrich Corp., St. Louis, MO), heparin (LEO Pharma, Ballerup, DK) and amoxicillin (Centrafarm, Etten-Leur, NL) and closed with a silicon cap. The catheter was fixed on the top of the head of animals using a bended piece of needle, four stainless steel screws and dental cement. Experiments were only performed after recovery to pre-surgery body weight (minimal 7 days).
Experimental procedures
Sixteen to 18 hours before the TG secretion measurement experiment, animals were connected to a metal collar for overnight adaptation. The metal collar served to guide the blood sampling cannula during blood sampling and was kept out of reach from the rats by way of a counterbalanced arm. Food was removed 4h before the experiment (i.e. 4 h before t = 0). At the same time animals were also connected to the blood sampling line.
To measure TG secretion, we used the Tyloxapol technique (Bruinstroop et al. Citation2012; Stafford et al. Citation2008). Tyloxapol is a drug that prevents TG uptake in tissue by blocking lipolytic activity and thus the breakdown of TG-rich lipoproteins and consequently results in hyperlipidemia (Baldissera et al. Citation2017; Schurr et al. Citation1972). The effects of Tyloxapol on TG uptake have been shown to last at least 6 h (Rasouli et al. Citation2016). TG uptake was blocked by an intravenous dose of 300 mg/kg Tyloxapol (Sigma-Aldrich, Germany) (Otway and Robinson Citation1967a, Citationb). After the intravenous injection of Tyloxapol, we took blood samples at regular intervals. The rate of increase in plasma TG concentration after the injection of Tyloxapol provides a measure for the rate of TG secretion. In separate experiments, we determined the rate of TG secretion at ZT3, ZT7, ZT11, ZT15, ZT19 and ZT23 (with ZT0 being the time of lights on).
Experiment 1: the daily rhythm of TG secretion
Animals (n = 48) were divided into six ZT point groups. Forty minutes before ZT2 (08:20), we injected Tyloxapol intravenously, immediately after taking a baseline blood sample (t = −40 min). The TG level at t = −40 was used to analyze the daily rhythm of basal plasma TG concentrations. Subsequently, blood samples (0.2 ml) were taken every 20 min until 160 min (ZT4) after the Tyloxapol injection. We determined the TG production rate between ZT2 and ZT4 by calculating the slope of the plasma TG rise from t = 0 (ZT2) to t = 120 (ZT4) by linear regression analysis. For the other time points along the L/D-cycle (ZT6, ZT10, ZT14, ZT18 and ZT22) the experimental procedure was the same, i.e. Tyloxapol was injected 40 min before the destined ZT and regular blood samples were taken every 20 min thereafter for 160 min.
Experiment 2: effect of a 6-meals-a-day feeding schedule on the daily rhythms in TG secretion, locomotor activity and body temperature
Animals were housed in separate cages, and divided into two groups. For one group of animals (n = 12), food was available AL group. The other group of animals (n = 16) was entrained to a 6-meals-a-day feeding schedule (with 1 meal every 4 h (6M group))(Kalsbeek and Strubbe Citation1998). Animals had access to food during three 12-min-bins equally spaced over the light period (i.e. ZT2, ZT6 and ZT10) and three 11-min-bins equally spaced over the dark period (i.e. ZT14, ZT18 and ZT22). Rats required approximately 2 weeks to adapt to this feeding schedule. Adaptation was considered to be complete when the rats consumed the same amount of food during the 12-h light and 12-h dark period. Animals received jugular vein surgery after adaptation to the 6M feeding schedule. TG secretion measurements were performed at ZT11 and ZT23 in both groups after animals had recovered to their pre-surgery body weight.
To measure activity, the rat cages were placed on a baseplate which in turn was placed on four parallel connected piezo-electronic sensors (Murata, 27 mm round disks). The voltage output of the sensors is proportional to relative changes in pressure, i.e. the activity of the rat. The signals of the Piezo-electric stabilimeter (activity) were transmitted to a PC-based analog computer interface (CED1404 Cambridge Electronic Design LTD), and transformed into absolute values, i.e. activity bouts. They were added up over a period of 5 min and stored into text files for later analysis.
A temperature sensitive data logger (DST nano-T) (Star-Oddi, Gardabaer, Iceland) was implanted subcutaneously in the dorsal region caudally to the brown adipose tissue when animals received their jugular vein surgery. Body temperature changes were measured by the subcutaneous temperature sensitive data loggers every 15 min and analyzed after sacrifice.
Experiment 3: the effect of a 6-meals-a-day feeding schedule on daily gene expression rhythms in the liver
Animals (n = 64) were divided into AL and 6M groups. After having been on the AL or 6M feeding schedule for 28 days, animals were anesthetized with 72% CO2 and sacrificed by decapitation at ZT2, ZT8, ZT14 and ZT20. After decapitation, liver tissue was collected and stored at −80°C.
TG measurement
Plasma TG was assayed using a kit from Roche (Mannheim, Germany). TG secretion rate at the different ZT points was determined by calculating the slope of the plasma TG rise over time by linear regression analysis.
RNA extraction and CDNA synthesis
Liver tissue was homogenized with a T10 basic UL TRA-TURRAX® homogenizer (IKA, Germany) with TRIzol (QIAGEN) and total RNA was isolated using the Trizol protocol. Total RNA was purified by NucleoSpin® RNAII Kit (Macherey-Nagel) and included a DNase step, according to the manufacturer’s instructions.
RNA was reverse transcribed using Transcriptor First cDNA Synthesis Kit (Roche) with oligo-dT primers (30 min at 55°C, 5 min at 85°C). Additional reverse transcriptase minus (-RT) controls were run to check for genomic DNA contamination.
Reverse transcription polymerase chain reaction (RT-PCR)
Gene expression was measured by quantitative RT-PCR (qRT-PCR) using the following reaction system: 2 μl cDNA was incubated in a final volume of 10 μl reaction containing 1×SensiFAST SYBR NO-ROX Mix and 25 ng of each primer (forward and reverse). qRT-PCR was performed in a Lightcycler®480 (Roche); primer information for each gene is shown in . The relative quantity of each gene was normalized against the geometric mean of Glyceraldehyde 3-phosphate dehydrogenase (Gapdh) and TATA box binding protein (Tbp).
Table 1. Information on gene primers.
Statistical analysis
Data are presented as mean±SEM (standard error of the mean). Statistical analysis was performed using SPSS version 20. In Experiment-1, one-way ANOVA was performed to detect the effect of Time (6 levels: ZT1, ZT5, ZT9, ZT13, ZT17 and ZT21 or ZT3, ZT7, ZT11, ZT15, ZT19 and ZT23) on basal plasma TG and TG secretion rate. In Experiments 2 and 3, two-way ANOVA was performed to detect the effects of Time (ZT11 versus ZT23 and ZT2 versus ZT8 versus ZT14 versus ZT20), Treatment (AL versus 6M) and Interaction for TG secretion and relative gene expression. ANOVA with repeated measures was performed to detect the effects of Time (day versus night), Treatment (AL versus 6M) and Interaction for locomotor activity, body temperature and food intake. If the effect for Time, Treatment or Interaction was significant, we performed a post-hoc analysis. For Experiment-2, we used independent T test as post-hoc analysis to analyze the effect of Time (ZT11 versus ZT 23) on TG secretion. We used paired T test as post-hoc analysis to analyze the effect of Time (day versus night) on locomotor activity, body temperature and food intake. For experiment-3, we used one-way ANOVA as post-hoc analysis to analyze the effect of Time (ZT2 versus ZT8 versus ZT14 versus ZT20) on gene expression. In addition, data were analyzed using the Circwave 1.4 software to test the daily rhythmicity of TG secretion, relative gene expression, locomotor activity and body temperature. P values reported are the result of the F-test. A 24 h rhythm was confirmed if p < 0.05.
Results
Experiment 1: the daily rhythm of TG secretion
We measured the basal level of plasma TG at ZT1, ZT5, ZT9, ZT13, ZT17 and ZT21 (). ANOVA did not reveal a significant effect of ZT Time for plasma TG levels (F = 1.861, p = 0.124), however, Circwave analysis showed a significant daily rhythm in basal plasma TG levels, with an acrophase at ZT6.
Figure 1. The daily rhythm of plasma TG (a) and TG secretion (b). Data are presented as mean±SEM. Gray area represents the dark period.
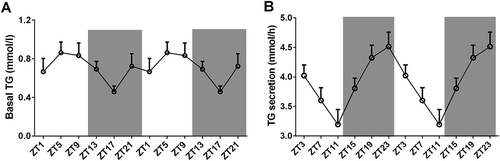
We calculated the TG secretion rate at ZT3, ZT7, ZT11, ZT15, ZT19 and ZT23 (). ANOVA showed a significant effect of ZT Time on TG secretion (F = 4.691, p = 0.002). TG secretion reached its lowest rate at ZT11 and its highest rate at ZT23. TG secretion rate at ZT10 was significantly lower than the rate at ZT3, ZT19 and ZT23 (p = 0.015, p = 0.001 and p < 0.001). TG secretion rate at ZT19 was significantly higher than the rates at ZT7 and ZT11 (p = 0.015 and p = 0.001). TG secretion rate at ZT23 was significantly higher than the rates at ZT7, ZT11 and ZT15 (p = 0.004, p < 0.001 and p = 0.029). Circwave confirmed the significant daily rhythm in TG secretion, with an acrophase of TG secretion at ZT22.
Experiment 2: effect of a 6-meals-a-day feeding schedule on the daily rhythms in TG secretion, locomotor activity and body temperature
We checked the effect of 6M on TG secretion at ZT11 and ZT23, i.e. according to the results of Experiment-1, the lowest and highest points of the daily rhythm in TG secretion. Basal plasma TG values did not differ between Treatment (F = 0.167, p = 0.687) or ZT times (F = 1.038, p = 0.320) (). We found that Time had a significant effect (F = 8.1666, p = 0.009) on TG secretion, with higher levels in the dark period, as in Experiment 1. The 6M Treatment tended to increase TG secretion during the light period (F = 3.844, p = 0.063), but this effect did not reach significance. Interaction did not show a significant effect (F = 0.828, p = 0.373). AL fed animals showed a significant day/night difference (p = 0.050), but in 6M animals, this day/night rhythm in TG secretion was lost (ZT11 and ZT23 did not significantly differ (p = 0.126)) ().
Figure 2. Effects of a 6-meals-a-day feeding (6M) schedule on basal plasma TG levels (a) and the daily TG secretion rhythm (b). Data are presented as mean±SEM. White bars – Ad libitum (AL) animals, Black bars – 6M animals. * indicates a significant difference between ZT11 and ZT23 for the AL animals, p ≤ 0.05.
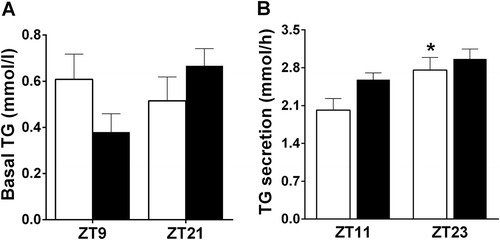
General activity and body temperature of 6M and AL rats showed a significant day/night rhythm (a–d). Circwave confirmed the significant daily rhythm in locomotor activity in 6M and AL rats, with an acrophase of locomotor activity at ZT18 in both groups. The amplitude of the daily locomotor activity rhythm in 6M animals was decreased to 89% of that in AL animals. Body temperature also showed a significant daily rhythm in the 6M and AL groups according to the Circwave analysis. The acrophase of body temperature was at ZT17 in the AL group and at ZT16 in the 6M group. The amplitude of the daily body temperature rhythm in 6M animals was 62% of that in AL animals.
Figure 3. Effects of a 6-meals-a-day feeding schedule (6M) on the 24 h profile of body temperature and locomotor activity. (a) The 24 h profile of body temperature; (b) the day/night rhythm of body temperature; (c) the 24 h profile of locomotor activity (values are percentages of 24 h-mean); (d) the day/night rhythm of locomotor activity (values are percentages of 24h-mean) and (e) the day/night rhythm of food intake. Data are presented as mean±SEM. White bars and Grey line – AL animals, Black bars and Black line – 6M animals. * indicates a significant difference between night and day, p < 0.05. # indicates a significant difference between 6M and AL animals, p < 0.05.
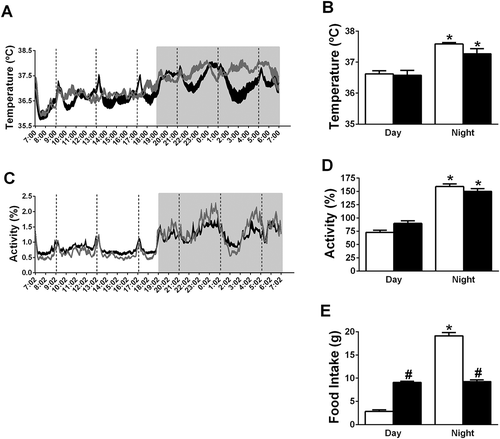
The 6M animals ate more than the AL animals during the light period, but ate less during the dark period (). The AL animals showed a significant day/night rhythm in food intake, but no such day/night rhythm was present in the 6M animals.
Experiment 3: the effect of a 6-meals-a-day feeding schedule on daily gene expression rhythms in liver
We measured the daily expression rhythm of five hepatic genes that are involved in TG synthesis and secretion (). Two-way ANOVA showed a significant Treatment (F = 4.381, p = 0.042) effect for the Scd1 expression level. One-way ANOVA showed that Time had a significant effect on the expression levels of Mttp and Scd1 in control animals, and Lipin1 expression in 6M animals ().
Table 2. Effects of the 6-meals-a-day feeding schedule on daily gene expression rhythms in liver.
Figure 4. Effects of a 6-meals-a-day (6M) feeding schedule on daily gene expression rhythms in liver. *p < 0.05. Open circles – data points of AL group. Solid line – Circwave calculated curve for AL group. Solid circles – data points of 6M group. Dashed line – circwave calculated curve for experimental 6M group.
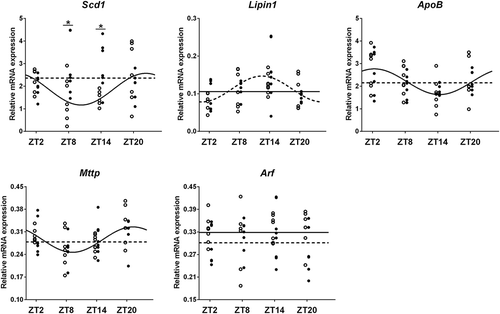
Circwave 1.4 analysis showed similar results (). Mttp, Scd1 and ApoB expression displayed a daily rhythm in control animals, but lost their daily rhythmicity under 6M feeding conditions. The expression of Lipin1 showed a clear daily rhythm in 6M rats, but not in control rats.
Table 3. Circwave V1.4 analysis of effects of a 6-meals-a-day feeding schedule on daily TG secretion related gene expression rhythms in liver.
Discussion
In the present study, we found that both plasma TG concentrations and TG secretion rates show a clear day/night rhythm. Day/night rhythms in plasma TG levels have been reported before, but this is the first time that a significant daily rhythm in TG secretion rate has been reported. TG secretion rates were lowest at the end of the light period and highest at the end of the night, i.e. approx. 6 h before the peak in plasma TG. It is well known that changes in feeding behavior affect the daily rhythm of plasma TG (Lanza-Jacoby et al. Citation1986; Pan and Hussain Citation2007; Pan et al. Citation2013; Shamsi et al. Citation2014; Yasumoto et al. Citation2016). In the present study, we showed that the day/night rhythm in TG secretion rate was lost when no clear day/night rhythm in feeding behavior was present anymore. On the other hand, we previously showed that the daily rhythm of plasma TG was not abolished under 6-meals-a-day conditions (Bruinstroop et al. Citation2013). Therefore, the present results show that the daily feeding rhythm is an important factor in the control of the daily rhythm of TG secretion, but TG secretion is not the only factor to control basal plasma TG concentrations.
In the present study, we found that the 24 h rhythm in plasma TG levels shows a peak in the middle of the light period and a trough in the middle of the dark period. This daily rhythm shows a shift of a few hours (2–4 h) compared to that of some other studies (Martin et al. Citation2011; Rudic et al. Citation2004; Sukumaran et al. Citation2010; Yasumoto et al. Citation2016). We think this shift may be due to the 4h fasting period we had to include before each sample in our study due to the Tyloxapol experiment. Also, TG secretion showed a clear daily rhythm, but with a peak at the end of the dark period and a trough at the end of the light period. Comparing the daily plasma TG and TG secretion rhythm shows that plasma TG levels reach a peak 6 h after TG secretion reaches peak levels and that plasma TG levels reach a trough 6 h after TG secretion reaches its lowest level, i.e. the daily rhythm of plasma TG is 6 h delayed as compared to the rhythm in TG secretion. Recently Moran-Ramos et al. (Moran-Ramos et al. Citation2017) and Kooijman et al. (Kooijman et al. Citation2015) showed that also TG clearance shows a day/night rhythm, with lowest TG uptake being found in the light period and highest uptake rates in the dark period. Clearly, such a day/night rhythm in plasma TG clearance will also affect the daily plasma TG rhythm, as the increased TG clearance in the dark period will result in a delay for the increased TG secretion to elicit an increase in plasma TG levels. Taking these findings together, we speculate that the daily rhythm in TG secretion is an important contributor for the generation of a daily rhythm in plasma TG, but clearly other contributors (such as a daily rhythm in TG uptake) are also involved.
Recently, Pan et al. (Pan and Hussain Citation2007) showed that the acute change in plasma TG levels in fasting mice after refeeding was mainly due to a change in non-HDL (high density lipoprotein) apoB-lipoproteins, such as VLDL. This indicates that food availability plays a major role in controlling plasma TG levels. Pan et al. (Pan and Hussain Citation2007) also showed that day-time restricted feeding induced two peaks in the daily plasma TG rhythm. One peak was during the time of restricted feeding and the other peak was at the end of the night. Additionally, other studies (Escobar et al. Citation1998; Lanza-Jacoby et al. Citation1986; Pan et al. Citation2013) showed that the daily rhythm in plasma TG was shifted in animals only able to feed during the light period. In the study of Shamsi et al. (Shamsi et al. Citation2014), day time feeding abolished the daily rhythm of plasma TG when animals were housed under a 16/8:light/dark cycle. The above findings indicate that the daily feeding rhythm is an important signal in the control of the daily rhythm in plasma TG. In the liver, Mttp and ApoB, two enzymes important for TG secretion, show a clear daily rhythm in their expression and activity level (Pan and Hussain Citation2007; Pan and Hussain Citation2009; Pan et al. Citation2013). Some studies (Pan and Hussain Citation2007; Citation2009; Pan et al. Citation2013) reported that restricted feeding shifts the daily rhythm of Mttp and ApoB expression, thus the daily feeding rhythm may also affect the daily rhythm of TG secretion. In the present study, we show that when a daily feeding rhythm was abolished due to the 6-meals-a-day feeding schedule, no significant difference in TG secretion was present anymore between ZT11 and ZT23 (i.e. the time points with the lowest and highest TG secretion rates in AL fed animals). This indicates that the daily rhythm of TG secretion may be lost in 6M animals. This idea is strengthened by the fact that the 6M schedule also abolished the daily rhythm of hepatic genes involved in TG secretion and the liver being the main organ to secrete TGs. In a previous study, we found that during the 6M feeding schedule, postprandial plasma TG levels increase most during the day time meals (Bruinstroop et al. Citation2013; Su et al. Citation2016b). In the present study, we found that feeding according the 6M schedule increased TG secretion during the day time (ZT11). These findings are nicely in line with previous human data showing that night time meals caused higher plasma TG levels, i.e. feeding at the wrong time of day causes increased plasma TG levels (Al-Naimi et al. Citation2004; Romon et al. Citation1992; Sopowski et al. Citation2001). Together, these findings clearly demonstrate that the daily rhythm in feeding activity is an important determinant of the daily rhythm in TG secretion.
Escobar et al. (Escobar et al. Citation1998) found that plasma TG levels show a persistent circadian rhythmicity in rats after 96 h fasting. Similarly, Fukagawa et al. (Fukagawa et al. Citation1994) also showed sustained daily plasma TG rhythms in fasted rats. These findings indicate that the circadian clock system can maintain a daily rhythm in plasma TG, in the absence of feeding activity. Pan et al. (Pan and Hussain Citation2009; Pan et al. Citation2010) showed that the daily rhythm of plasma TG as well as the daily rhythm in MTTP expression, both at the protein and mRNA level, were lost in Clock mutant animals. It is also well known (Pan and Hussain Citation2009) that daily rhythms of other clock genes are dampened or lost in Clock mutant animals. Although the daily feeding pattern was not determined in that study, locomotor activity rhythms were less affected in these animals under a L/D cycle. This suggests that an intact molecular clock system is important for maintaining the daily rhythms of plasma TG and TG secretion. Pan et al. (Pan and Hussain Citation2009; Pan et al. Citation2010) also showed that restricted feeding (in the inactive period) failed to entrain the daily rhythm in plasma TG and genes involved in TG secretion in Clock mutant animals, indicating that feeding activity requires an intact circadian clock system to regulate the daily TG rhythm. Our own study and other studies (Cailotto et al. Citation2005; Kuroda et al. Citation2012; Sen et al. Citation2017; Su et al. Citation2016a) showed that daily clock gene rhythms in the liver were not abolished in 6M animals, indicating that the abolishment of the daily rhythms in TG secretion and related gene expression are due to the arrhythmic feeding in the 6M animals and not to arrhythmic clock gene expression rhythms. Sen et al. (Sen et al. Citation2017) recently showed that the daily rhythm of clock gene expression was shifted under a 6M schedule in mice, therefore it cannot be excluded yet that the arrhythmic feeding abolished the daily rhythms in TG secretion and related gene expression via a differential shift of some daily clock gene rhythms. Hence, both the circadian clock system and a daily feeding rhythm are essential to maintain the daily rhythm in TG secretion, if one of these two signals is abolished, TG secretion becomes arrhythmic.
Some studies (Pan and Hussain Citation2007; Pan and Hussain Citation2009) have shown that the daily rhythm of plasma TG levels and MTTP activity were abolished under constant light conditions, indicating also that the light-sensitive central clock (the suprachiasmatic nucleus of the hypothalamus (SCN)) is important for regulating plasma TG levels and MTTP activity. However, in these conditions, feeding rhythms will also be disturbed. Many studies (Damiola et al. Citation2000; Hara et al. Citation2001; Stokkan et al. Citation2001) have shown that restricted feeding does not alter the daily rhythm of clock gene expression in the SCN. In the present study, we found that the daily rhythms of locomotor activity and body temperature were still intact in 6M animals, in agreement with the idea that the oscillator in the SCN is still intact under 6M conditions. Thus, arrhythmic feeding, and not an arrhythmic SCN, is the main factor responsible for abolishment of the daily rhythm in TG secretion and gene expression in the 6M animals.
In summary, in the present study, we found that plasma TG levels and TG secretion show a significant daily rhythm. The acrophase of TG secretion is 6 h in advance to that of plasma TG, thus, a daily rhythm in TG secretion contributes to the daily rhythm in plasma TG generation, but other factors, such as TG uptake, are also important for rhythm generation. We also found that arrhythmic feeding abolished the daily rhythm in TG secretion and related hepatic genes, indicating that the daily rhythm in feeding activity is an important determinant for maintaining the daily rhythm of TG production and secretion.
Declaration of interest
The authors report no conflicts of interest. The authors alone are responsible for the content and writing of the paper.
Additional information
Funding
References
- Al-Naimi S, Hampton SM, Richard P, Tzung C, Morgan LM. 2004. Postprandial metabolic profiles following meals and snacks eaten during simulated night and day shift work. Chronobiol Int. 21:937–47.
- Asp L, Claesson C, Boren J, Olofsson SO. 2000. ADP-ribosylation factor 1 and its activation of phospholipase D are important for the assembly of very low density lipoproteins. J Biol Chem. 275:26285–92.
- Baldissera MD, Souza CF, Grando TH, Doleski PH, Boligon AA, Stefani LM, Monteiro SG. 2017. Hypolipidemic effect of beta-caryophyllene to treat hyperlipidemic rats. Naunyn Schmiedebergs Arch Pharmacol. 390:215–23.
- Bruinstroop E, La Fleur SE, Ackermans MT, Foppen E, Wortel J, Kooijman S, Berbee JF, Rensen PC, Fliers E, Kalsbeek A. 2013. The autonomic nervous system regulates postprandial hepatic lipid metabolism. Am J Physiol Endocrinol Metab. 304:E1089–1096.
- Bruinstroop E, Pei L, Ackermans MT, Foppen E, Borgers AJ, Kwakkel J, Alkemade A, Fliers E, Kalsbeek A. 2012. Hypothalamic neuropeptide Y (NPY) controls hepatic VLDL-triglyceride secretion in rats via the sympathetic nervous system. Diabetes. 61:1043–50.
- Cailotto C, La Fleur SE, Van Heijningen C, Wortel J, Kalsbeek A, Feenstra M, Pevet P, Buijs RM. 2005. The suprachiasmatic nucleus controls the daily variation of plasma glucose via the autonomic output to the liver: Are the clock genes involved? Eur J Neurosci. 22:2531–40.
- Chen Y, Rui BB, Tang LY, Hu CM. 2015. Lipin family proteins–key regulators in lipid metabolism. Ann Nutr Metab. 66:10–18.
- Damiola F, Le Minh N, Preitner N, Kornmann B, Fleury-Olela F, Schibler U. 2000. Restricted feeding uncouples circadian oscillators in peripheral tissues from the central pacemaker in the suprachiasmatic nucleus. Genes Dev. 14:2950–61.
- Escobar C, Diaz-Munoz M, Encinas F, Aguilar-Roblero R. 1998. Persistence of metabolic rhythmicity during fasting and its entrainment by restricted feeding schedules in rats. Am J Physiol. 274:R1309–1316.
- Fukagawa K, Gou HM, Wolf R, Tso P. 1994. Circadian rhythm of serum and lymph apolipoprotein AIV in ad libitum-fed and fasted rats. Am J Physiol. 267:R1385–1390.
- Gorne LD, Acosta-Rodriguez VA, Pasquare SJ, Salvador GA, Giusto NM, Guido ME. 2015. The mouse liver displays daily rhythms in the metabolism of phospholipids and in the activity of lipid synthesizing enzymes. Chronobiol Int. 32:11–26.
- Grundy SM. 2004. Obesity, metabolic syndrome, and cardiovascular disease. J Clin Endocrinol Metab. 89:2595–600.
- Guerrero-Vargas NN, Espitia-Bautista E, Buijs RM, Escobar C. 2018. Shift-work: Is time of eating determining metabolic health? Evidence from animal models. Proc Nutr Soc. Jan 8:1–17.
- Hara R, Wan K, Wakamatsu H, Aida R, Moriya T, Akiyama M, Shibata S. 2001. Restricted feeding entrains liver clock without participation of the suprachiasmatic nucleus. Genes Cells. 6:269–78.
- Itani O, Kaneita Y, Tokiya M, Jike M, Murata A, Nakagome S, Otsuka Y, Ohida T. 2017. Short sleep duration, shift work, and actual days taken off work are predictive life-style risk factors for new-onset metabolic syndrome: A seven-year cohort study of 40,000 male workers. Sleep Med. 39:87–94.
- Jamil H, Dickson JK Jr., Chu CH, Lago MW, Rinehart JK, Biller SA, Gregg RE, Wetterau JR. 1995. Microsomal triglyceride transfer protein. Specificity of lipid binding and transport. J Biol Chem. 270:6549–54.
- Kalsbeek A, Strubbe JH. 1998. Circadian control of insulin secretion is independent of the temporal distribution of feeding. Physiol Behav. 63:553–58.
- Kooijman S, Van Den Berg R, Ramkisoensing A, Puig LS, Cooman CP, Meijer JH, Al E. 2015. Daily and seasonal encoding of rhythm in brown adipose tissue activity. In Thesis”Neural control of lipid metabolism and inflammation” 2015.Leiden: Leiden University.
- Kuroda H, Tahara Y, Saito K, Ohnishi N, Kubo Y, Seo Y, Otsuka M, Fuse Y, Ohura Y, Hirao A, et al. 2012. Meal frequency patterns determine the phase of mouse peripheral circadian clocks. Sci Rep. 2:711.
- Lam TK, Gutierrez-Juarez R, Pocai A, Bhanot S, Tso P, Schwartz GJ, Rossetti L. 2007. Brain glucose metabolism controls the hepatic secretion of triglyceride-rich lipoproteins. Nat Med. 13:171–80.
- Lanza-Jacoby S, Stevenson NR, Kaplan ML. 1986. Circadian changes in serum and liver metabolites and liver lipogenic enzymes in ad libitum- and meal-fed, lean and obese Zucker rats. J Nutr. 116:1798–809.
- Liu S, Brown JD, Stanya KJ, Homan E, Leidl M, Inouye K, Bhargava P, Gangl MR, Dai L, Hatano B, et al. 2013. A diurnal serum lipid integrates hepatic lipogenesis and peripheral fatty acid use. Nature. 502:550–54.
- Lu YC, Wang CP, Yu TH, Tsai IT, Hung WC, Lu IC, Hsu CC, Tang WH, Houng JY, Chung FM, et al. 2017. Shift work is associated with metabolic syndrome in male steel workers-the role of resistin and WBC count-related metabolic derangements. Diabetol Metab Syndr. 9:83.
- Martin C, Passilly-Degrace P, Gaillard D, Merlin JF, Chevrot M, Besnard P. 2011. The lipid-sensor candidates CD36 and GPR120 are differentially regulated by dietary lipids in mouse taste buds: Impact on spontaneous fat preference. PLoS One. 6:e24014.
- Miller M, Stone NJ, Ballantyne C, Bittner V, Criqui MH, Ginsberg HN, Goldberg AC, Howard WJ, Jacobson MS, Kris-Etherton PM, et al. 2011. Triglycerides and cardiovascular disease: A scientific statement from the American heart association. Circulation. 123:2292–333.
- Mondola P, Gambardella P, Santangelo F, Santillo M, Greco AM. 1995. Circadian rhythms of lipid and apolipoprotein pattern in adult fasted rats. Physiol Behav. 58:175–80.
- Moran-Ramos S, Guerrero-Vargas NN, Mendez-Hernandez R, Basualdo MDC, Escobar C, Buijs RM. 2017. The suprachiasmatic nucleus drives day-night variations in postprandial triglyceride uptake into skeletal muscle and brown adipose tissue. Exp Physiol. 102:1584–95.
- Mukherji A, Kobiita A, Damara M, Misra N, Meziane H, Champy MF, Chambon P. 2015. Shifting eating to the circadian rest phase misaligns the peripheral clocks with the master SCN clock and leads to a metabolic syndrome. Proc Natl Acad Sci U S A. 112:E6691–6698.
- Olofsson SO, Boren J. 2005. Apolipoprotein B: A clinically important apolipoprotein which assembles atherogenic lipoproteins and promotes the development of atherosclerosis. J Intern Med. 258:395–410.
- Olofsson SO, Stillemark-Billton P, Asp L. 2000. Intracellular assembly of VLDL: Two major steps in separate cell compartments. Trends Cardiovasc Med. 10:338–45.
- Otway S, Robinson DS. 1967a. The effect of a non-ionic detergent (Triton WR 1339) on the removal of triglyceride fatty acids from the blood of the rat. J Physiol. 190:309–19.
- Otway S, Robinson DS. 1967b. The use of a non-ionic detergent (Triton WR 1339) to determine rates of triglyceride entry into the circulation of the rat under different physiological conditions. J Physiol. 190:321–32.
- Pan X, Hussain MM. 2007. Diurnal regulation of microsomal triglyceride transfer protein and plasma lipid levels. J Biol Chem. 282:24707–19.
- Pan X, Hussain MM. 2009. Clock is important for food and circadian regulation of macronutrient absorption in mice. J Lipid Res. 50:1800–13.
- Pan X, Munshi MK, Iqbal J, Queiroz J, Sirwi AA, Shah S, Younus A, Hussain MM. 2013. Circadian regulation of intestinal lipid absorption by apolipoprotein AIV involves forkhead transcription factors A2 and O1 and microsomal triglyceride transfer protein. J Biol Chem. 288:20464–76.
- Pan X, Zhang Y, Wang L, Hussain MM. 2010. Diurnal regulation of MTP and plasma triglyceride by CLOCK is mediated by SHP. Cell Metab. 12:174–86.
- Pramono LA, Harbuwono DS. 2015. Managing hypertriglyceridemia in daily practice. Acta Med Indones. 47:265–71.
- Rasouli M, Tahmouri H, Mosavi-Mehr M. 2016. The long term kinetic of plasma lipids and lipoproteins in tyloxapol injected rats. J Clin Diagn Res. 10:BF01–05.
- Reiner Z. 2017. Hypertriglyceridaemia and risk of coronary artery disease. Nat Rev Cardiol. 14:401–11.
- Romon M, Nuttens MC, Fievet C, Pot P, Bard JM, Furon D, Fruchart JC. 1992. Increased triglyceride levels in shift workers. Am J Med. 93:259–62.
- Rudic RD, McNamara P, Curtis AM, Boston RC, Panda S, Hogenesch JB, Fitzgerald GA. 2004. BMAL1 and CLOCK, two essential components of the circadian clock, are involved in glucose homeostasis. PLoS Biol. 2:e377.
- Salgado-Delgado R, Angeles-Castellanos M, Saderi N, Buijs RM, Escobar C. 2010. Food intake during the normal activity phase prevents obesity and circadian desynchrony in a rat model of night work. Endocrinology. 151:1019–29.
- Schernhammer ES, Thompson CA. 2011. Light at night and health: The perils of rotating shift work. Occup Environ Med. 68:310–11.
- Schurr PE, Schultz JR, Parkinson TM. 1972. Triton-induced hyperlipidemia in rats as an animal model for screening hypolipidemic drugs. Lipids. 7:68–74.
- Sen S, Raingard H, Dumont S, Kalsbeek A, Vuillez P, Challet E. 2017. Ultradian feeding in mice not only affects the peripheral clock in the liver, but also the master clock in the brain. Chronobiol Int. 34:17–36.
- Shamsi NA, Salkeld MD, Rattanatray L, Voultsios A, Varcoe TJ, Boden MJ, Kennaway DJ. 2014. Metabolic consequences of timed feeding in mice. Physiol Behav. 128:188–201.
- Sopowski MJ, Hampton SM, Ribeiro DC, Morgan L, Arendt J. 2001. Postprandial triacylglycerol responses in simulated night and day shift: Gender differences. J Biol Rhythms. 16:272–76.
- Stafford JM, Yu F, Printz R, Hasty AH, Swift LL, Niswender KD. 2008. Central nervous system neuropeptide Y signaling modulates VLDL triglyceride secretion. Diabetes. 57:1482–90.
- Stokkan KA, Yamazaki S, Tei H, Sakaki Y, Menaker M. 2001. Entrainment of the circadian clock in the liver by feeding. Science. 291:490–93.
- Su Y, Cailotto C, Foppen E, Jansen R, Zhang Z, Buijs R, Fliers E, Kalsbeek A. 2016a. The role of feeding rhythm, adrenal hormones and neuronal inputs in synchronizing daily clock gene rhythms in the liver. Mol Cell Endocrinol. 422:125–31.
- Su Y, Foppen E, Zhang Z, Fliers E, Kalsbeek A. 2016b. Effects of 6-meals-a-day feeding and 6-meals-a-day feeding combined with adrenalectomy on daily gene expression rhythms in rat epididymal white adipose tissue. Genes Cells. 21:6–24.
- Sukumaran S, Xue B, Jusko WJ, Dubois DC, Almon RR. 2010. Circadian variations in gene expression in rat abdominal adipose tissue and relationship to physiology. Physiol Genomics. 42A:141–52.
- Van Oostrom AJ, Castro Cabezas M, Ribalta J, Masana L, Twickler TB, Remijnse TA, Erkelens DW. 2000. Diurnal triglyceride profiles in healthy normolipidemic male subjects are associated to insulin sensitivity, body composition and diet. Eur J Clin Invest. 30:964–71.
- Yasumoto Y, Hashimoto C, Nakao R, Yamazaki H, Hiroyama H, Nemoto T, Yamamoto S, Sakurai M, Oike H, Wada N, et al. 2016. Short-term feeding at the wrong time is sufficient to desynchronize peripheral clocks and induce obesity with hyperphagia, physical inactivity and metabolic disorders in mice. Metabolism. 65:714–27.
- Zhao I, Bogossian F, Turner C. 2012. A cross-sectional analysis of the association between night-only or rotating shift work and overweight/obesity among female nurses and midwives. J Occup Environ Med. 54:834–40.