Abstract
Lipid rafts and caveolae are detergent‐insoluble plasma membrane microdomains, involved in cellular endocytic processes and signalling. Several viruses, including a human pathogen, echovirus 1, and an extensively studied simian virus 40 utilize these domains for internalization into the host cells. Interaction of viruses with receptors on the cell surface triggers specific conformational changes of the virus particle and can give rise to signalling events, which determine the mechanisms of virus entry. After internalization via cell surface lipid rafts or caveolae, virus‐containing vesicles can fuse with caveosomes, pre‐existing cytoplasmic organelles, or dock on other intracellular organelles. These pathways may deliver viruses further to different cellular destinations, where the viral replication cycle then takes place. The information concerning the viral entry processes is important for understanding the details of the infections, for finding new targets for antiviral therapy and for elucidating the cellular internalization pathways in general.
Key messages
Interaction of viruses with their cell surface receptors determines the subsequent internalization events. The entry process is often controlled by specific signalling cascades.
In addition to classical clathrin‐mediated endocytosis, viruses can use several other internalization mechanisms, like those involving cell surface caveolae and lipid rafts, which direct them to distinct intracellular locations for replication.
Viruses can be used as tools to study the complex cellular endocytic mechanisms.
Early events in virus infection
Viruses consist of a protein capsid surrounding the nucleic acid (DNA or RNA) genome and in some viruses this structure is further covered by a lipid envelope. To initiate infection in the host, the virus must be able to deliver its genetic material into the target cells. This requires specific recognition of cell surface molecules and subsequent entry process. During evolution, viruses have adapted to utilize receptors with different physiological functions for the initiation of their life cycle () (reviewed in Citation1). Specific sites in the virus particle are responsible for these interactions that can include complex recognition events followed by conformational changes in the virus particle and the receptors.
Table 1. Examples of molecules known to act as virus receptors.
The expression of cell surface molecules varies in different tissues due to the distinct functions of the cells. Specific virus‐cell recognition events are essential in viral tissue tropism and pathogenesis of the infection. In some cases the clinical outcome of the infection can be largely explained by the receptor specificity. In Epstein‐Barr virus infection, B‐cells expressing the complement receptors recognized by the virus Citation2 are the main target, and in rabies, the virus interacts with the acetylcholine receptor during migration from the exposed peripheral area to the central nervous system Citation3. However, the pathogenic process is usually complex and in addition to receptor recognition, intracellular interactions between cellular and viral macromolecules play an important role.
Viruses may either use several receptor molecules during the early events (attachment, entry and uncoating) of infection, or, in some cases, only one molecule may be sufficient to bring about all these steps. For instance, human immunodeficiency virus (HIV) is bound to the CD4 molecule on leukocytes but this interaction does not directly lead to the initiation of the infection. Instead, it gives rise to conformational changes in the virus structure allowing further interaction with chemokine receptors and this cascade eventually facilitates the entry of the virus core into the host cells. The same or highly similar cell surface molecules are recognized by different viruses (). Some cell surface molecules (e.g. poliovirus receptor, coxsackievirus‐adenovirus receptor) have been primarily identified due to their ability to bind viruses and further studies have elucidated their cellular functions.
After recognition of the cell surface receptor, viruses usually enter the cells utilizing processes which are used for internalization of extracellular material necessary during the physiological life cycle of the cells (reviewed in Citation4). The most thoroughly studied mechanism of viral entry is the clathrin‐mediated endocytosis pathway (). This route is utilized, for instance, by influenza viruses and adenoviruses. After interaction between the virus and the receptor, the virus particles move into clathrin‐coated pits and the vesicles are internalized into the cytoplasm. They can fuse with the early endosomes, and the ligand can be further delivered to the late endosomes or to other intracellular organelles. Subsequently, the viral genome needs to be released from the membranous vesicle. This procedure varies between viruses and may take advantage of viral fusion peptides or virus‐induced rupture of the vesicle. Some viruses (e.g. HIV and measles virus) are able to enter the cells by direct fusion of the viral envelope with the plasma membrane, which leads to release of the viral core into the cytoplasm of the cell.
Figure 1 Schematic presentation of viral endocytosis. Virus‐receptor interaction 1) gives rise to formation of virus‐containing membrane vesicles 2), which are internalized into the host cell 3). Subsequently, the viral core is released from these structures 4) by a fusion of the viral envelope and the cellular vesicular membrane or by a rupture of the endocytic vesicle.
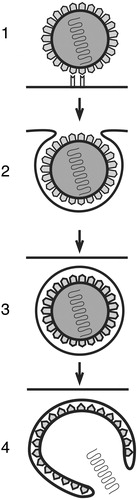
As outlined below, recent research has revealed new viral entry routes, including caveolae‐ and lipid raft‐mediated endocytosis, and their detailed characterization is underway. These investigations will lead to further understanding of general cellular processes used in the internalization of extracellular material, elucidation of viral pathogenesis as well as finding novel potential targets for antiviral therapy.
Lipid rafts: Composition and cellular function
The original view of plasma membrane structure is based on the fluid‐mosaic model by Singer and Nicholson Citation5. They proposed that membrane proteins and lipids can freely diffuse within the plane of the bilayer. Since then, numerous studies revisiting the architecture of biological membranes have created a more complex picture. Presently, plasma membranes are considered to be laterally heterogeneous and composed of structurally and functionally distinct microdomains, including lipid rafts. Despite the fact that rafts have been extensively studied for a relatively long time, there are still various hypotheses about their nature.
The most commonly cited hypothesis Citation6 describes lipid rafts as relatively large (larger than 50 nm) structures, enriched with cholesterol and (glyco)sphingolipids and certain proteins. Based on this proposal, the outer leaflet of the plasma membrane rafts contains sphingomyelin and glycosphingolipids, such as ganglioside GM1 Citation7, while the inner leaflet contains glycerophospholipids (reviewed in Citation6). The more recently published ‘shell’ hypothesis of lipid rafts Citation8 proposes that certain proteins interact with cholesterol‐rich assemblies and form small shells. These shells can then, in activating conditions, fuse together and form larger rafts. Moreover, the experiments with glycosylphosphatidylinositol (GPI)‐anchored proteins in living cells Citation9–11, suggest that pre‐existing lipid assemblies are small and dynamic high density structures, which can be induced to form larger rafts.
Certain proteins, such as GPI‐anchored proteins Citation9, Citation12, and signalling molecules, like tyrosine kinases of the Src family Citation13, Citation14 can be associated with lipid rafts. The morphologically distinct raft microdomain invaginations, with high amounts of caveolin proteins on the cytoplasmic leaflet, are termed caveolae Citation15. Several studies indicate that many proteins are not constitutively present in rafts but protein cross‐linking with a ligand or antibodies may collect them to the raft regions Citation16 and induce signalling events (reviewed in Citation17). The functions of rafts can be associated, for example, with cholesterol transport (reviewed in Citation18), endocytosis Citation19, and signal transduction (reviewed in Citation17).
Assembly of rafts takes place in the Golgi complex from where they move to the plasma membrane. The recycling back to the Golgi may regulate their distribution and composition Citation20. The raft composition is dependent especially on cellular control of cholesterol biosynthesis, uptake and deposition. Defects in these processes or in the composition and amount of rafts can result in diseases (reviewed in Citation21). For example, in Alzheimer disease, raft‐dependent processing of amyloid‐beta‐peptide, a hallmark of the illness, is impaired Citation22.
Traditionally, purification of lipid rafts and their components has been based on their insolubility in detergents, such as cold 1% Triton‐X‐100 Citation12 and on their low density in sucrose gradients. Therefore, lipid rafts have been considered to be detergent‐resistant membranes (DRMs) Citation12. Also, sequestering of cholesterol with chemical compounds or oxidation of cholesterol by cholesterol oxidase may prevent raft formation (reviewed in Citation23). While these approaches have been widely used to identify the protein components and to monitor raft‐mediated signalling, they may result in wrong interpretations. For example, many cell components may be detergent‐resistant even though they are not present in rafts (reviewed in Citation24) and, moreover, rafts are not always sensitive to cholesterol depletion Citation25. Therefore, it remains to be seen whether some earlier described structural and functional properties of rafts must be reconsidered. Other detection methods for rafts, including characterization of determinants of protein segregation to lipid rafts with fluorescent probes Citation26, are required for future studies.
Caveolae and caveosomes
Caveolae are the best characterized types of lipid rafts. They were described over 50 years ago as stable flask‐shaped invaginations of the plasma membrane Citation27, Citation28. Caveolae are present on the surface of many cell types and they differ from non‐caveolar lipid rafts by containing caveolin‐1 as their main protein component Citation15, Citation29. Caveolin‐1 is a palmitylated, cholesterol‐binding protein Citation30, Citation31, expressed in various tissues. The caveolin gene family also includes two other members: caveolin‐2, which is usually coexpressed with caveolin‐1 Citation32, Citation33, and caveolin‐3 which is found in muscle tissue Citation34, Citation35. Rapid transport of cholesterol to the cell surface is dependent on caveolin‐1 expression Citation36 and cholesterol, in turn, is required for the existence of caveolae Citation15, Citation37, Citation38. Caveolins can oligomerize in the endoplasmic reticulum (ER) and the Golgi Citation39 to form a filamentous coat of cell surface caveolae Citation40. A pool of caveolin‐1 is found in the Golgi complex Citation29, in caveosomes Citation41 and also in endosomes of clathrin‐mediated endocytosis Citation42, Citation43.
Originally, Parton et al. Citation44 demonstrated that the internalization of caveolae could be regulated by kinase inhibitors, and this was followed by another study Citation45 showing that the purified caveolae contain molecular components needed for regulated transport, including various signalling molecules. The later findings revealed that caveolar signalling molecules include serine/threonine protein kinase Raf‐1 Citation46, protein kinase C‐alpha Citation47 and protein tyrosine kinases of src‐family Citation14. Also, endothelial nitric oxide synthase (eNOS) is targeted to caveolae via palmitylation and thus caveolae may regulate the synthesis of nitric oxide (NO) Citation48. Tyrosine phosphorylation of caveolin‐1 Citation49 is important for some of these signalling events.
Because caveolae regulate several signalling cascades, mutations or defects in caveolin proteins play a role in the development of illnesses, including diabetes, cancer, cardiovascular diseases, atherosclerosis, pulmonary fibrosis, and a variety of degenerative muscular dystrophies (reviewed in Citation50). Mutations in caveolin‐3 gene are linked to certain hereditary forms of muscular dystrophy Citation51 and its expression is upregulated in the brain tissue of Alzheimer patients Citation52. Surprisingly, caveolin‐1 knock‐out mice are viable and fertile even though they exhibit a complete loss of endothelial caveolae Citation53. However, their life‐span is shortened Citation54 and they suffer from pulmonary defects and vascular and hyperproliferative abnormalities related to impaired NO and calcium signalling Citation53.
Caveolin‐1 and caveolae are partially immobile at the plasma membrane Citation55 and their endocytosis requires a stimulus such as a ligand binding. Caveolar fission from the cell surface to the cytoplasm is inducible by GTPases Citation19, like dynamin‐2 Citation56, Citation57, and it may also require protein kinase C Citation58 and actin Citation44, Citation59. However, dynamin‐2 is not a specific marker for caveolar fission, because it also acts in formation of clathrin‐coated vesicle Citation60 and may, in addition, be involved in currently less well‐defined endocytic mechanisms Citation61. The fission of vesicles is regulated by a balance of caveolin‐1, cholesterol and glycosphingolipids at the plasma membrane Citation62. Because of the tight regulation of caveolar function, caveolae can process surface‐bound ligands differentially Citation45. The ligands of the caveolar route involve autocrine mobility factor Citation63, cholera toxin Citation64, Citation65, bacteria Citation66, viruses Citation67 and prions Citation68.
The cytoplasmic caveolae are discrete carrier vesicles that can merge with endosomal vesicles of the clathrin‐mediated pathway to release their cargo in there Citation43 or fuse with caveosomes Citation41. Caveosomes are pH‐neutral, caveolin‐1 positive, pre‐existing organelles rich in cholesterol and glycosphingolipids Citation41. The caveolar endocytosis, however, may not be the only route from the cell surface to caveosomes Citation69. From caveosomes, the ligands can be sorted to other cellular locations, such as the ER Citation41, Citation70.
Viruses that enter host cells via caveolar endocytosis and caveosomes
The endocytic routes of simian virus 40 Citation41, Citation69, Citation71, Citation72 and echovirus 1 Citation73, Citation74 to caveosomes are discussed here in detail, because their entry mechanisms have been investigated most extensively (). Other viruses that may utilize the caveolar entry pathway in certain cell types include polyomaviruses Citation75, influenza viruses Citation76, and coronaviruses Citation77. Caveolar pathway has also been associated with the endocytosis of some papillomavirus types Citation78 and respiratory syncytial virus Citation79, Citation80.
Figure 2 Endocytosis of simian virus 40 and echovirus 1 to the caveosomes.A. Simian virus 40 is endocytosed into caveosomes via caveolae and caveolar vesicles. In some cell types the virus can enter the caveosomes directly from lipid rafts in non‐coated vesicles. Some of internalized SV40 particles can also be found in the endosomes. B. Echovirus 1 is internalized together with its receptor, α2β1 integrin, into caveosomes via cell surface caveolae or by an alternative pathway, which may originate from lipid rafts and does not involve clathrin‐coated pits. EV1 may remain in caveosomes prior to initiation of replication.
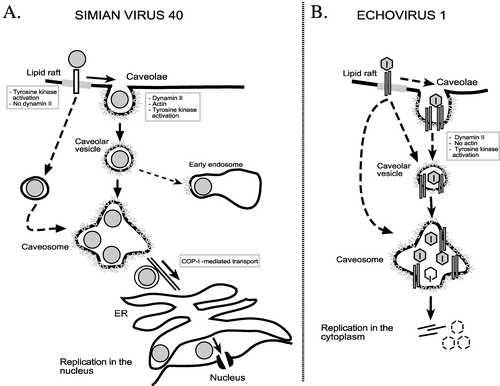
Simian virus 40 (SV40)
This non‐enveloped DNA virus of the papovavirus family, can bind onto the cell surface using two different receptors, ganglioside GM1, located in lipid rafts Citation81, and major histocompatibility (MHC) class I molecule Citation82. The original studies showed that binding of SV40 to the MHC class I molecules results in the receptor clustering and redistribution with the virus to the cell surface caveolae Citation83, Citation84. The virus is tightly enclosed into caveolae which are smaller in the presence of the virus Citation83. SV40 may recruit more caveolae from the cytoplasm and, moreover, even new caveolae may form to the site of entry Citation85. After 20 min, the virus‐containing caveolae invaginate from the cell membrane which is caused by a cascade of virus‐induced signalling events. These involve local tyrosine kinase phosphorylation and protein kinase C activation Citation41, Citation86, as well as production of phosphatidylinositol 4,5‐bisphosphate Citation85. The signalling leads first to the transient breakdown of actin stress fibers and then to recruitment of actin to caveolae where it polymerizes again and forms patches that serve as platforms for actin tail formation Citation72. Tyrosine kinase phosphorylation recruits dynamin‐2 transiently to the neck of caveolae. Finally, the caveolar vesicles are formed and released into the cytoplasm Citation72, where they associate with caveosomes. The MHC class I molecule is not endocytosed with the virus Citation83.
In normal conditions, a majority of SV40 particles are found in caveosomes, however, a small proportion of the virus is also trapped from caveolar vesicles into early endosomes by GTPase (guanosine 5'‐triphosphate‐hydrolysing enzyme) Rab5‐dependent manner Citation43. Overexpression of a constantly active form of Rab5 results in an artificial guiding of most of the virus into the endosomes, however, the infection does not proceed Citation43.
In the green monkey kidney cell line CV‐1, the uptake and replication of SV40 is efficiently prevented with inhibitors specific for lipid rafts and/or caveolae Citation71, Citation72 and with dominant negative mutants of caveolin‐1, caveolin‐3 and dynamin‐2 Citation41, Citation72, Citation87. Somewhat unexpectedly, SV40 was recently shown to be able to infect caveolin‐1 knock‐out mouse cells Citation69. The alternative pathway, like caveolar endocytosis of SV40, bypasses the endocytic organelles of clathrin route, is dependent on cholesterol and carries the virus into the caveosome‐like organelles in cells lacking caveolin‐1. However, the alternative pathway is more rapid and does not require dynamin‐2 Citation69. Interestingly, this pathway can also be occupied in cells that express caveolin‐1. The uptake of SV40 via the alternative pathway may begin from non‐caveolar lipid rafts of the plasma membrane. Then, the virus is internalized in small intracellular, uncoated vesicles to caveosome‐like organelles or to caveosomes Citation69.
Once in the caveosomes, SV40 can remain there for several hours, after which it is sorted by tubular, caveolin‐1 negative carriers that move along the microtubules to the ER Citation41, Citation88. These events may involve COPI‐ and COPII‐coated carrier vesicles Citation88. From the ER, SV40 enters the nucleus through the nuclear pore complexes for replication (reviewed in Citation89) ().
A related virus, murine polyomavirus, binds also to gangliosides (GD1a, GT1b), present in lipid rafts Citation81, Citation90, and depending on the target host cells, the virus is internalized via a non‐caveolar, non‐clathrin dependent manner Citation91, Citation92 or through caveolae Citation75, Citation93. From caveolae, the internalization proceeds to caveosomes and by a microtubule‐dependent manner to the ER Citation93.
Echovirus 1 (EV1)
Echovirus 1 is a member of the picornavirus family of non‐enveloped, single‐stranded RNA viruses. On the host cell, EV1 binds to the I domain of α2β1 integrin, a collagen receptor Citation94, Citation95. Antibodies against β2‐microglobulin can also prevent EV1 infection, but whether this molecule has a role in the virus entry is currently unknown Citation96. Based on cryo‐electron microscopy reconstruction of EV1‐α2β1 integrin interaction, the multiple integrin heterodimers can bind to the adjacent sites of the virus capsid Citation97. This interaction may induce clustering of the integrin molecules Citation97 and result in relocation of integrins from lipid rafts to the caveolae‐like structures Citation98. Immuno‐electron microscopy of the infected cells reveals that some EV1 particles are clearly located into uncoated cell surface structures that are morphologically indistinguishable from caveolae Citation73. However, in live fluorescence microscopy, most EV1 particles were not detected in green fluorescent protein (GFP)‐tagged caveolin 1‐containing cell surface structures Citation74. This indicated that EV1 may, like SV40 Citation69, also enter the host cell caveosomes by an alternative mechanism, which could involve non‐caveolar lipid rafts ().
Protein kinase Cα is activated during the EV1 internalization process and tyrosine phosphorylation is required for the efficient replication cycle Citation74, Citation98. The infection cycle is partially dependent on dynamin‐2 and cholesterol and it can be, to some extent, inhibited by a dominant negative mutant of caveolin‐3 Citation73, Citation74. The requirement of the intact actin cortex in EV1 uptake depends on the cell type, since the drugs interfering the actin network had no effect on the EV1 infectivity in the green monkey kidney cell line CV‐1, but actin‐stabilizing agent diminished viral internalization into another cell line Citation74. Dominant negative mutant of caveolin‐1, that inhibits SV40 entry, had no effect on EV1 internalization or infectivity, suggesting significant differences in the early entry steps between these two viruses.
After rapid internalization, intracellular EV1 is found in small, caveolin‐1 positive vesicles and then already in 15 min in larger, caveolin‐1 positive organelles. The large organelles were identified to be caveosomes because EV1 partially colocalized with SV40 during the uptake, the infection was blocked by lipid raft/caveolae/caveosomal inhibitors, and the virus was observed in caveosome‐like structures in the EM Citation73, Citation74. The uptake of EV1 into caveosomes seems to be faster than the internalization of SV40 into these structures. The α2β1 integrin remains associated with EV1 in caveosomes Citation74.
EV1 has not so far been located in any other intracellular organelle like in the ER or the Golgi after the uptake into caveosomes. A microtubule‐disturbing agent has no effect on EV1 infectivity, implicating that the virus is not transported further from caveosomes by a microtubule‐dependent step. Since a remarkable amount of genomic viral RNA was observed in the caveosomes, these organelles could act as the final destination of the virus, and the uncoating and the release of the viral genome could be initiated there Citation74 ().
The uptake of both SV40 Citation41, Citation69, Citation72 and EV1 Citation73, Citation74, Citation98 into caveosomes appears to be caveolae/non‐caveolar lipid raft‐derived, tyrosine kinase‐dependent and largely bypasses endosomes and lysosomes. In a recent genome‐wide analysis of human kinases during SV40 endocytosis, integrin signalling was observed to control SV40 entry pathway Citation99. Interestingly, α2β1 integrin‐mediated signalling may also direct internalization of EV1 into caveosomes Citation98. However, there are some significant differences in detailed endocytic mechanisms of these viruses (e.g. the speed of virus internalization). Moreover, the caveosomal route directs SV40 and EV1 into different sites of replication in the cell; SV40 to the nucleus and EV1 to the cytoplasm. It will be relevant to find out how the alternative pathway(s) to caveosomes and further endocytic sorting of viruses from caveosomes can affect the replication efficiency of viruses and the outcome of infection.
Viruses that use the non‐caveolar raft‐mediated endocytosis
Lipid rafts may facilitate cell‐surface interactions and internalization of several viruses, including avian sarcoma and leucosis virus (ASLV) Citation100, HIV Citation101, measles virus Citation102, certain picornaviruses Citation74, Citation103–105, rotaviruses Citation106, and SV40 Citation69. For example, viruses may be transported by non‐caveolar lipid raft‐mediated endocytosis into caveosomes Citation69, as discussed in the previous section, or into endosomes, as shown with ASLV Citation100. However, the detailed uptake mechanisms via non‐caveolar rafts are poorly characterized.
Enteroviruses
Echovirus 11 (EV11), a picornavirus, which belongs to the enterovirus genus like EV1, may use lipid rafts during the internalization process Citation103. Decay accelerating factor (DAF), a complement regulatory protein, acts as an attachment receptor for a wide group of enteroviruses including EV11 Citation107. DAF belongs to GPI‐anchored glycoproteins that can be located in lipid rafts Citation16. The uptake of the DAF‐binding strain of EV11 can be inhibited with drugs interfering with cholesterol traffic, actin cytoskeleton and microtubules. Moreover, the virus has been copurified with the DRM fraction, strongly indicating the involvement of rafts in the internalization process. Caveolin‐1 was present in the virus‐positive raft fraction in one cell line but not detectable in another cell line, suggesting EV11 could enter alternatively via rafts/caveolae or through lipid rafts alone Citation103. Another DAF‐utilizing enterovirus, coxsackievirus B4 (CBV4), was reported to enter via rafts to the Golgi Citation105. Moreover, the internalization of a related enterovirus, coxsackievirus A9 (CAV9), may be lipid raft‐dependent Citation104. However, the role of caveolin‐1 containing structures in the endocytosis of CAV9 and CBV4 remains to be determined.
Other viruses
The receptors of rotavirus, non‐enveloped double‐stranded RNA virus, include a ganglioside GM1, integrins and a heat shock protein 70 that are associated with DRMs on the cell surface Citation108. Likewise, infectious rotavirus is located within lipid rafts during the entry Citation108 and cholesterol and dynamin‐2 GTPase are required for an efficient infection. The fact that rotavirus can infect cells where caveolar or clathrin‐mediated uptake routes are inhibited Citation106 suggests that non‐caveolar lipid rafts, instead of caveolae, are used in its endocytosis.
Findings concerning the role of lipid rafts in HIV entry are still controversial. HIV‐1 infection triggers lateral diffusion of specific membrane components after the virus has interacted with CD4, enabling subsequent interactions with coreceptors Citation101, Citation109. These interactions are proposed to occur mainly in lipid rafts containing CD4 and result in productive infection of T cells Citation110, Citation111. Also, viral entry is inhibited if membrane rafts are destroyed with cholesterol depletion before virus binding Citation101 and HIV entry to the brain microvascular endothelial cells by macropinocytosis is dependent on lipid rafts Citation112. Other studies propose that the presence of HIV‐1 receptors in the rafts is not required for virus entry and that cholesterol can modulate the endocytic process independently of its ability to promote raft formation Citation113. However, fusion of the virus with the plasma membrane and subsequent steps of entry may require lipid rafts Citation114.
Conclusions
Our knowledge of the role of lipid rafts, caveolae and caveosomes in cellular endocytic processes is rapidly increasing. From a virological point of view, it is of special interest that studies on the entry process of SV40 resulted in the identification of caveosomes, previously unrecognized cellular organelles Citation41. Further research has revealed that other viruses also utilize this pathway or take advantage of the non‐caveolar lipid rafts. Several recent reports reveal previously unknown connections between different endocytotic pathways in the cells and viruses are one powerful tool to investigate these phenomena.
Pathogenesis of virus infections is largely dependent on the expression of specific molecules on the cell surface for virus attachment and entry. Our understanding on the viral receptors is still largely limited to the cultured cells and it can be expected that more complex interactions take place during clinical infections. The role of different entry routes in the outcome of the viral infections is poorly understood but this information can provide us with deeper insight into the initiation of clinical infections and it can give us additional tools for their prevention and treatment. It has already been shown that the entry and uncoating of some viruses (e.g. HIV and rhinoviruses) can be prevented by drugs and the future will most probably offer new examples of the clinical usefulness of the molecular information. In gene therapy, targeting of viral vectors to correct tissues and cells is essential and our knowledge of receptors and internalization pathways will undoubtedly help to improve these methods in the future.
References
- Hyypiä T. Viral host cell receptors. Nature Publishing Group, London 2003
- Fingeroth J. D., Weis J. J., Tedder T. F., Strominger J. L., Biro P. A., Fearon D. T. Epstein‐Barr virus receptor of human B lymphocytes is the C3d receptor CR2. Proc Natl Acad Sci U S A 1984; 81: 4510–4
- Lentz T. L., Burrage T. G., Smith A. L., Crick J., Tignor G. H. Is the acetylcholine receptor a rabies virus receptor?. Science 1982; 215: 182–4
- Smith A. E., Helenius A. How viruses enter animal cells. Science 2004; 304: 237–42
- Singer S. J., Nicholson G. L. The fluid‐mosaic model of the structure of cell membranes. Science 1972; 175: 720–31
- Simons K., Ikonen E. Functional rafts in cell membranes. Nature 1997; 387: 569–72
- Parton R. G. Ultrastructural localization of gangliosides; GM1 is concentrated in caveolae. J Histochem Cytochem 1994; 42: 155–66
- Anderson R. G., Jacobson K. A role for lipid shells in targeting proteins to caveolae, rafts, and other lipid domains. Science 2002; 296: 1821–5
- Varma R., Mayor S. GPI‐anchored proteins are organized in submicron domains at the cell surface. Nature 1998; 394: 798–801
- Sharma P., Varma R., Sarasij R. C., Ira, Gousset K., Krishnamoorthy G. Nanoscale organization of multiple GPI‐anchored proteins in living cell membranes. Cell 2004; 116: 577–89
- Friedrichson T., Kurzchalia T. V. Microdomains of GPI‐anchored proteins in living cells revealed by crosslinking. Nature 1998; 394: 802–5
- Brown D. A., Rose J. K. Sorting of GPI‐anchored proteins to glycolipid‐enriched membrane subdomains during transport to the apical cell surface. Cell 1992; 68: 533–44
- Foster L. J., De Hoog C. L., Mann M. Unbiased quantitative proteomics of lipid rafts reveals high specificity for signaling factors. Proc Natl Acad Sci U S A 2003; 100: 5813–8
- Sargiacomo M., Sudol M., Tang Z., Lisanti M. P. Signal transducing molecules and glycosyl‐phosphatidylinositol‐linked proteins form a caveolin‐rich insoluble complex in MDCK cells. J Cell Biol 1993; 122: 789–807
- Rothberg K. G., Heuser J. E., Donzell W. C., Ying Y. S., Glenney J. R., Anderson R. G. Caveolin, a protein component of caveolae membrane coats. Cell 1992; 68: 673–82
- Mayor S., Rothberg K. G., Maxfield F. R. Sequestration of GPI‐anchored proteins in caveolae triggered by cross‐linking. Science 1994; 264: 1948–51
- Simons K., Toomre D. Lipid rafts and signal transduction. Nat Rev Mol Cell Biol 2000; 1: 31–9
- Simons K., Ikonen E. How cells handle cholesterol. Science 2000; 290: 1721–6
- Schnitzer J. E., Oh P., McIntosh D. P. Role of GTP hydrolysis in fission of caveolae directly from plasma membranes. Science 1996; 274: 239–42
- Nichols B. J., Kenworthy A. K., Polishchuk R. S., Lodge R., Roberts T. H., Hirschberg K. Rapid cycling of lipid raft markers between the cell surface and Golgi complex. J Cell Biol 2001; 153: 529–41
- Simons K., Ehehalt R. Cholesterol, lipid rafts, and disease. J Clin Invest 2002; 110: 597–603
- Ehehalt R., Keller P., Haass C., Thiele C., Simons K. Amyloidogenic processing of the Alzheimer beta‐amyloid precursor protein depends on lipid rafts. J Cell Biol 2003; 160: 113–23
- Ikonen E., Parton R. G. Caveolins and cellular cholesterol balance. Traffic 2000; 1: 212–7
- Munro S. Lipid rafts: elusive or illusive?. Cell 2003; 115: 377–88
- Schuck S., Honsho M., Ekroos K., Shevchenko A., Simons K. Resistance of cell membranes to different detergents. Proc Natl Acad Sci U S A 2003; 100: 5795–800
- Zacharias D. A., Violin J. D., Newton A. C., Tsien R. Y. Partitioning of lipid‐modified monomeric GFPs into membrane microdomains of live cells. Science 2002; 296: 913–6
- Palade G. Fine structure of blood capillaries. J Appl Physiol 1953; 24: 1424
- Yamada E. The fine structure of the gall bladder epithelium of the mouse. J Biophys Biochem Cytol 1955; 1: 455–8
- Kurzchalia T. V., Dupree P., Parton R. G., Kellner R., Virta H., Lehnert M. VIP21, a 21‐kD membrane protein is an integral component of trans‐Golgi‐network‐derived transport vesicles. J Cell Biol 1992; 118: 1003–14
- Dietzen D. J., Hastings W. R., Lublin D. M. Caveolin is palmitoylated on multiple cysteine residues. Palmitoylation is not necessary for localization of caveolin to caveolae. J Biol Chem 1995; 270: 6838–42
- Murata M., Peränen J., Schreiner R., Wieland F., Kurzchalia T. V., Simons K. VIP21/caveolin is a cholesterol‐binding protein. Proc Natl Acad Sci U S A 1995; 92: 10339–43
- Scheiffele P., Verkade P., Fra A. M., Virta H., Simons K., Ikonen E. Caveolin‐1 and ‐2 in the exocytic pathway of MDCK cells. J Cell Biol 1998; 140: 795–806
- Scherer P. E., Okamoto T., Chun M., Nishimoto I., Lodish H. F., Lisanti M. P. Identification, sequence, and expression of caveolin‐2 defines a caveolin gene family. Proc Natl Acad Sci U S A 1996; 93: 131–5
- Parton R. G., Way M., Zorzi N., Stang E. Caveolin‐3 associates with developing T‐tubules during muscle differentiation. J Cell Biol 1997; 136: 137–54
- Way M., Parton R. G. M‐caveolin, a muscle‐specific caveolin‐related protein. FEBS Lett 1995; 376: 108–12
- Smart E. J., Ying Y., Donzell W. C., Anderson R. G. A role for caveolin in transport of cholesterol from endoplasmic reticulum to plasma membrane. J Biol Chem 1996; 271: 29427–35
- Hailstones D., Sleer L. S., Parton R. G., Stanley K. K. Regulation of caveolin and caveolae by cholesterol in MDCK cells. J Lipid Res 1998; 39: 369–79
- Chang W. J., Rothberg K. G., Kamen B. A., Anderson R. G. Lowering the cholesterol content of MA104 cells inhibits receptor‐mediated transport of folate. J Cell Biol 1992; 118: 63–9
- Monier S., Parton R. G., Vogel F., Behlke J., Henske A., Kurzchalia T. V. VIP21‐caveolin, a membrane protein constituent of the caveolar coat, oligomerizes in vivo and in vitro. Mol Biol Cell 1995; 6: 911–27
- Fernandez I., Ying Y., Albanesi J., Anderson R. G. Mechanism of caveolin filament assembly. Proc Natl Acad Sci U S A 2002; 99: 11193–8
- Pelkmans L., Kartenbeck J., Helenius A. Caveolar endocytosis of simian virus 40 reveals a new two‐step vesicular‐transport pathway to the ER. Nat Cell Biol 2001; 3: 473–83
- Gagescu R., Demaurex N., Parton R. G., Hunziker W., Huber L. A., Gruenberg J. The recycling endosome of Madin‐Darby canine kidney cells is a mildly acidic compartment rich in raft components. Mol Biol Cell 2000; 11: 2775–91
- Pelkmans L., Burli T., Zerial M., Helenius A. Caveolin‐stabilized membrane domains as multifunctional transport and sorting devices in endocytic membrane traffic. Cell 2004; 118: 767–80
- Parton R. G., Joggerst B., Simons K. Regulated internalization of caveolae. J Cell Biol 1994; 127: 1199–215
- Schnitzer J. E., McIntosh D. P., Dvorak A. M., Liu J., Oh P. Separation of caveolae from associated microdomains of GPI‐anchored proteins. Science 1995; 269: 1435–9
- Mineo C., James G. L., Smart E. J., Anderson R. G. Localization of epidermal growth factor‐stimulated Ras/Raf‐1 interaction to caveolae membrane. J Biol Chem 1996; 271: 11930–5
- Mineo C., Ying Y. S., Chapline C., Jaken S., Anderson R. G. Targeting of protein kinase Calpha to caveolae. J Cell Biol 1998; 141: 601–10
- Garcia‐Cardena G., Oh P., Liu J., Schnitzer J. E., Sessa W. C. Targeting of nitric oxide synthase to endothelial cell caveolae via palmitoylation: implications for nitric oxide signaling. Proc Natl Acad Sci U S A 1996; 93: 6448–53
- Glenney J. R., J. r. Tyrosine phosphorylation of a 22‐kDa protein is correlated with transformation by Rous sarcoma virus. J Biol Chem 1989; 264: 20163–6
- Cohen A. W., Hnasko R., Schubert W., Lisanti M. P. Role of caveolae and caveolins in health and disease. Physiol Rev 2004; 84: 1341–79
- Woodman S. E., Sotgia F., Galbiati F., Minetti C., Lisanti M. P. Caveolinopathies: mutations in caveolin‐3 cause four distinct autosomal dominant muscle diseases. Neurology 2004; 62: 538–43
- Nishiyama K., Trapp B. D., Ikezu T., Ransohoff R. M., Tomita T., Iwatsubo T. Caveolin‐3 upregulation activates beta‐secretase‐mediated cleavage of the amyloid precursor protein in Alzheimer's disease. J Neurosci 1999; 19: 6538–48
- Drab M., Verkade P., Elger M., Kasper M., Lohn M., Lauterbach B. Loss of caveolae, vascular dysfunction, and pulmonary defects in caveolin‐1 gene‐disrupted mice. Science 2001; 293: 2449–52
- Park D. S., Cohen A. W., Frank P. G., Razani B., Lee H., Williams T. M. Caveolin‐1 null (‐/‐) mice show dramatic reductions in life span. Biochemistry 2003; 42: 15124–31
- Mundy D. I., Machleidt T., Ying Y. S., Anderson R. G., Bloom G. S. Dual control of caveolar membrane traffic by microtubules and the actin cytoskeleton. J Cell Sci 2002; 115: 4327–39
- Henley J. R., Krueger E. W., Oswald B. J., McNiven M. A. Dynamin‐mediated internalization of caveolae. J Cell Biol 1998; 141: 85–99
- Oh P., McIntosh D. P., Schnitzer J. E. Dynamin at the neck of caveolae mediates their budding to form transport vesicles by GTP‐driven fission from the plasma membrane of endothelium. J Cell Biol 1998; 141: 101–14
- Smart E. J., Foster D. C., Ying Y. S., Kamen B. A., Anderson R. G. Protein kinase C activators inhibit receptor‐mediated potocytosis by preventing internalization of caveolae. J Cell Biol 1994; 124: 307–13
- Conrad P. A., Smart E. J., Ying Y. S., Anderson R. G., Bloom G. S. Caveolin cycles between plasma membrane caveolae and the Golgi complex by microtubule‐dependent and microtubule‐independent steps. J Cell Biol 1995; 131: 1421–33
- Damke H., Baba T., Warnock D. E., Schmid S. L. Induction of mutant dynamin specifically blocks endocytic coated vesicle formation. J Cell Biol 1994; 127: 915–34
- Lamaze C., Dujeancourt A., Baba T., Lo C. G., Benmerah A., Dautry‐Varsat A. Interleukin 2 receptors and detergent‐resistant membrane domains define a clathrin‐independent endocytic pathway. Mol Cell 2001; 7: 661–71
- Sharma D. K., Brown J. C., Choudhury A., Peterson T. E., Holicky E., Marks D. L. Selective stimulation of caveolar endocytosis by glycosphingolipids and cholesterol. Mol Biol Cell 2004; 15: 3114–22
- Benlimame N., Le P. U., Nabi I. R. Localization of autocrine motility factor receptor to caveolae and clathrin‐independent internalization of its ligand to smooth endoplasmic reticulum. Mol Biol Cell 1998; 9: 1773–86
- Montesano R., Roth J., Robert A., Orci L. Non‐coated membrane invaginations are involved in binding and internalization of cholera and tetanus toxins. Nature 1982; 296: 651–3
- Nichols B. J. A distinct class of endosome mediates clathrin‐independent endocytosis to the Golgi complex. Nat Cell Biol 2002; 4: 374–8
- Shin J. S., Gao Z., Abraham S. N. Involvement of cellular caveolae in bacterial entry into mast cells. Science 2000; 289: 785–8
- Pelkmans L., Helenius A. Endocytosis via caveolae. Traffic 2002; 3: 311–20
- Peters P. J., Mironov A., Peretz D., Jr, van Donselaar E., Leclerc E., Erpel S. Trafficking of prion proteins through a caveolae‐mediated endosomal pathway. J Cell Biol 2003; 162: 703–17
- Damm E. M., Pelkmans L., Kartenbeck J., Mezzacasa A., Kurzchalia T., Helenius A. Clathrin‐ and caveolin‐1‐independent endocytosis: entry of simian virus 40 into cells devoid of caveolae. J Cell Biol 2005; 168: 477–88
- Le P. U., Nabi I. R. Distinct caveolae‐mediated endocytic pathways target the Golgi apparatus and the endoplasmic reticulum. J Cell Sci 2003; 116: 1059–71
- Anderson H. A., Chen Y., Norkin L. C. Bound simian virus 40 translocates to caveolin‐enriched membrane domains, and its entry is inhibited by drugs that selectively disrupt caveolae. Mol Biol Cell 1996; 7: 1825–34
- Pelkmans L., Puntener D., Helenius A. Local actin polymerization and dynamin recruitment in SV40‐induced internalization of caveolae. Science 2002; 296: 535–9
- Marjomäki V., Pietiäinen V., Matilainen H., Upla P., Ivaska J., Nissinen L. Internalization of echovirus 1 in caveolae. J Virol 2002; 76: 1856–65
- Pietiäinen V., Marjomäki V., Upla P., Pelkmans L., Helenius A., Hyypiä T. Echovirus 1 endocytosis into caveosomes requires lipid rafts, dynamin II, and signaling events. Mol Biol Cell 2004; 15: 4911–25
- Richterova Z., Liebl D., Horak M., Palkova Z., Stokrova J., Hozak P. Caveolae are involved in the trafficking of mouse polyomavirus virions and artificial VP1 pseudocapsids toward cell nuclei. J Virol 2001; 75: 10880–91
- Nunes‐Correia I., Eulalio A., Nir S., Pedroso de Lima M. C. Caveolae as an additional route for influenza virus endocytosis in MDCK cells. Cell Mol Biol Lett 2004; 9: 47–60
- Nomura R., Kiyota A., Suzaki E., Kataoka K., Ohe Y., Miyamoto K. Human coronavirus 229E binds to CD13 in rafts and enters the cell through caveolae. J Virol 2004; 78: 8701–8
- Bousarghin L., Touze A., Sizaret P. Y., Coursaget P. Human papillomavirus types 16, 31, and 58 use different endocytosis pathways to enter cells. J Virol 2003; 77: 3846–50
- Brown G., Jeffree C. E., McDonald T., Rixon H. W., Aitken J. D., Sugrue R. J. Analysis of the interaction between respiratory syncytial virus and lipid‐rafts in Hep2 cells during infection. Virology 2004; 327: 175–85
- Werling D., Hope J. C., Chaplin P., Collins R. A., Taylor G., Howard C. J. Involvement of caveolae in the uptake of respiratory syncytial virus antigen by dendritic cells. J Leukoc Biol 1999; 66: 50–8
- Tsai B., Gilbert J. M., Stehle T., Lencer W., Benjamin T. L., Rapoport T. A. Gangliosides are receptors for murine polyoma virus and SV40. EMBO J 2003; 22: 4346–55
- Bernacchi S., Mueller G., Langowski J., Waldeck W. Characterization of simian virus 40 on its infectious entry pathway in cells using fluorescence correlation spectroscopy. Biochem Soc Trans 2004; 32: 746–9
- Anderson H. A., Chen Y., Norkin L. C. MHC class I molecules are enriched in caveolae but do not enter with simian virus 40. J Gen Virol 1998; 79: 1469–77
- Stang E., Kartenbeck J., Parton R. G. Major histocompatibility complex class I molecules mediate association of SV40 with caveolae. Mol Biol Cell 1997; 8: 47–57
- Pelkmans L., Helenius A. Insider information: what viruses tell us about endocytosis. Curr Opin Cell Biol 2003; 15: 414–22
- Dangoria N. S., Breau W. C., Anderson H. A., Cishek D. M., Norkin L. C. Extracellular simian virus 40 induces an ERK/MAP kinase‐independent signalling pathway that activates primary response genes and promotes virus entry. J Gen Virol 1996; 77: 2173–82
- Roy S., Luetterforst R., Harding A., Apolloni A., Etheridge M., Stang E. Dominant‐negative caveolin inhibits H‐Ras function by disrupting cholesterol‐rich plasma membrane domains. Nat Cell Biol 1999; 1: 98–105
- Richards A. A., Stang E., Pepperkok R., Parton R. G. Inhibitors of COP‐mediated transport and cholera toxin action inhibit simian virus 40 infection. Mol Biol Cell 2002; 13: 1750–64
- Kasamatsu H., Nakanishi A. How do animal DNA viruses get to the nucleus?. Annu Rev Microbiol 1998; 52: 627–86
- Smith A. E., Lilie H., Helenius A. Ganglioside‐dependent cell attachment and endocytosis of murine polyomavirus‐like particles. FEBS Lett 2003; 555: 199–203
- Gilbert J. M., Goldberg I. G., Benjamin T. L. Cell penetration and trafficking of polyomavirus. J Virol 2003; 77: 2615–22
- Gilbert J. M., Benjamin T. L. Early steps of polyomavirus entry into cells. J Virol 2000; 74: 8582–8
- Gilbert J., Benjamin T. Uptake pathway of polyomavirus via ganglioside GD1a. J Virol 2004; 78: 12259–67
- Bergelson J. M., Shepley M. P., Chan B. M., Hemler M. E., Finberg R. W. Identification of the integrin VLA‐2 as a receptor for echovirus 1. Science 1992; 255: 1718–20
- Bergelson J. M., St John N. F., Kawaguchi S., Pasqualini R., Berdichevsky F., Hemler M. E. The I domain is essential for echovirus 1 interaction with VLA‐2. Cell Adhes Commun 1994; 2: 455–64
- Ward T., Powell R. M., Pipkin P. A., Evans D. J., Minor P. D., Almond J. W. Role for beta2‐microglobulin in echovirus infection of rhabdomyosarcoma cells. J Virol 1998; 72: 5360–5
- Xing L., Huhtala M., Pietiäinen V., Käpyla J., Vuorinen K., Marjomäki V. Structural and functional analysis of integrin alpha2I domain interaction with echovirus 1. J Biol Chem 2004; 279: 11632–8
- Upla P., Marjomäki V., Kankaanpää P., Ivaska J., Hyypiä T., Van Der Goot F. G. Clustering induces a lateral redistribution of alpha 2 beta 1 integrin from membrane rafts to caveolae and subsequent protein kinase C‐dependent internalization. Mol Biol Cell 2004; 15: 625–36
- Pelkmans L., Fava E., Grabner H., Hannus M., Habermann B., Krausz E. Genome‐wide analysis of human kinases in clathrin‐ and caveolae/raft‐mediated endocytosis. Nature 2005
- Narayan S., Barnard R. J., Young J. A. Two retroviral entry pathways distinguished by lipid raft association of the viral receptor and differences in viral infectivity. J Virol 2003; 77: 1977–83
- Manes S., del Real G., Lacalle R. A., Lucas P., Gomez‐Mouton C., Sanchez‐Palomino S. Membrane raft microdomains mediate lateral assemblies required for HIV‐1 infection. EMBO Rep 2000; 1: 190–6
- Avota E., Muller N., Klett M., Schneider‐Schaulies S. Measles virus interacts with and alters signal transduction in T‐cell lipid rafts. J Virol 2004; 78: 9552–9
- Stuart A. D., Eustace H. E., McKee T. A., Brown T. D. A novel cell entry pathway for a DAF‐using human enterovirus is dependent on lipid rafts. J Virol 2002; 76: 9307–22
- Triantafilou K., Triantafilou M. Lipid raft microdomains: key sites for Coxsackievirus A9 infectious cycle. Virology 2003; 317: 128–35
- Triantafilou K., Triantafilou M. Lipid‐raft‐dependent Coxsackievirus B4 internalization and rapid targeting to the Golgi. Virology 2004; 326: 6–19
- Sanchez‐San Martin C., Lopez T., Arias C. F., Lopez S. Characterization of rotavirus cell entry. J Virol 2004; 78: 2310–8
- Ward T., Pipkin P. A., Clarkson N. A., Stone D. M., Minor P. D., Almond J. W. Decay‐accelerating factor CD55 is identified as the receptor for echovirus 7 using CELICS, a rapid immuno‐focal cloning method. EMBO J 1994; 13: 5070–4
- Isa P., Realpe M., Romero P., Lopez S., Arias C. F. Rotavirus RRV associates with lipid membrane microdomains during cell entry. Virology 2004; 322: 370–81
- Nisole S., Krust B., Hovanessian A. G. Anchorage of HIV on permissive cells leads to coaggregation of viral particles with surface nucleolin at membrane raft microdomains. Exp Cell Res 2002; 276: 155–73
- Popik W., Alce T. M., Au W. C. Human immunodeficiency virus type 1 uses lipid raft‐colocalized CD4 and chemokine receptors for productive entry into CD4(+) T cells. J Virol 2002; 76: 4709–22
- Del Real G., Jimenez‐Baranda S., Lacalle R. A., Mira E., Lucas P., Gomez‐Mouton C. Blocking of HIV‐1 infection by targeting CD4 to nonraft membrane domains. J Exp Med 2002; 196: 293–301
- Liu N. Q., Lossinsky A. S., Popik W., Li X., Gujuluva C., Kriederman B. Human immunodeficiency virus type 1 enters brain microvascular endothelia by macropinocytosis dependent on lipid rafts and the mitogen‐activated protein kinase signaling pathway. J Virol 2002; 76: 6689–700
- Percherancier Y., Lagane B., Planchenault T., Staropoli I., Altmeyer R., Virelizier J. L. HIV‐1 entry into T‐cells is not dependent on CD4 and CCR5 localization to sphingolipid‐enriched, detergent‐resistant, raft membrane domains. J Biol Chem 2003; 278: 3153–61
- Popik W., Alce T. M. CD4 receptor localized to non‐raft membrane microdomains supports HIV‐1 entry. Identification of a novel raft localization marker in CD4. J Biol Chem 2004; 279: 704–12