Abstract
Possibilities of using the skin for somatic gene therapy have been investigated for more than 20 years. Strategies have included both direct gene transfer into the skin and indirect gene transfer utilizing cultured cells as an intermediate step for gene manipulation. Viral as well as nonviral vectors have been used, and both gene addition and gene editing have been performed. Although cutaneous gene therapy has now begun translating into clinical medicine (as seen by the first clinical gene therapy project of an inherited skin disorder) further developments are still required.
Introduction
The skin was one of the first tissues considered for somatic gene therapy—initially as a general vehicle for the supply of gene products. Thus in 1987 publications described cutaneous expression of three different proteins: human growth hormone, factor IX and adenosine deaminase Citation1–3. Since these pioneering studies numerous experiments have been performed, exploring possibilities for gene therapy of diseases such as inherited skin diseases and wounds (reviewed in Citation4–6). Several factors are inhibiting the translation of these projects into the clinic including loss of gene expression, immune reactions and limited blood supply Citation7–10.
Epidermis is a natural source of secreted proteins with both local and systemic effects, and after gene transfer numerous medically relevant proteins can be secreted from genetically modified epidermal tissue into the blood Citation11. These proteins include hormones (such as leptin, erythropoietin, and human growth hormone), the coagulation factors factor VIII and factor IX, apolipoprotein E, α1‐antitrypsin, various cytokines, monoclonal antibodies, and antimicrobial peptides Citation8,Citation12–19. Most studies used constitutive expression of these proteins, but regulated gene expression can also be achieved after both viral and nonviral gene transfer using both the tetracycline system and synthetic ligand‐driven intracellular heterodimerization Citation20–23. It is possible to select in vivo for the gene‐targeted cells Citation24. Pfützner et al. showed that colchicine treatment increased the percentage of multidrug resistance (MDR) expressing keratinocytes to almost 100% on mice Citation25. The transduced keratinocytes were able to proliferate normally and capable of forming a stratified differentiated epidermis.
In tissue culture, gene transfer using optimized murine retroviral vectors can lead to transduction frequencies approaching 100%, making it unnecessary to select for transduced cells Citation26. The transgene expression and copy number in the keratinocytes can be even further increased by employing a centrifugation technique for the transduction Citation27. Fibronectin can also be used to enhance the transduction frequency Citation28.
Key messages
After more than 20 years of research cutaneous gene therapy has now reached the clinical phase.
Future developments will include improved gene delivery methods.
Cutaneous gene therapy, initially relevant for rare inherited skin diseases, may eventually be used for systemic disorders as well.
Stem cells
Epidermal stem cells have been extensively studied and are obviously important for skin gene therapy. In general, stem cells can be defined as cells with the capacity for unlimited self‐renewal and the ability to generate daughter cells that undergo differentiation. In human interfollicular epidermis, basal cells thought to be one class of epidermal stem cells, are found in clusters located at the tip of the dermal papilla Citation29. These cells are multipotent and can be induced to differentiate into hair and cells of the sebaceous gland (reviewed in Citation30). Stem cells in the bulge area of the hair follicle are probably more multipotent and can give rise not only to the hair lineages but also to sebocytes and interfollicular epidermis Citation31. One prevailing model is that, under steady state conditions, stem cells are unipotent (i.e. producing only follicular or only interfollicular epidermis), whereas during regeneration after tissue damage, they display multipotency. Stem cells in the hair follicle bulge do not normally contribute cells to the epidermis, but after epidermal injury they are recruited into the epidermis. Although the bulge‐derived cells acquire an epidermal phenotype, most are eliminated from the epidermis over several weeks Citation32. Thus, epidermal and hair follicle stem cells are considered as distinct populations. Plucked hair follicle‐derived cells have been used in preparing skin equivalents, and can form epithelium after implantation in deep wounds Citation33,34. Thus the hair follicle bulge area is considered as an abundant, easily accessible source of actively growing, pluripotent adult stem cells Citation35. Interestingly, hair follicle stem cells have been shown to be able to differentiate into neurons, glial cells, keratinocytes and smooth muscle cells, and in animal models they enhanced the rate of nerve regeneration and the restoration of nerve function Citation36.
Telomerase in stem cells
Telomerase is active in the germ line, immortal, and tumor cells, and in cells from continuously renewing normal tissues, and is thought to be correlated with an indefinite life span (reviewed in Citation37. In general, it is hypothesized that stem cells do not have sufficient telomerase to prevent telomere shortening associated with continuous tissue renewal. However, telomerase cannot be used as a skin stem cell marker, since an enriched population of epidermal stem cells exhibited less telomerase activity than the more actively proliferating transit amplifying cells Citation38. Telomerase and telomeres are important for the normal function of epidermal stem cells. Shortening of telomeres inhibits mobilization of stem cells, impairs hair growth, and suppresses their proliferative capacity. In contrast, overexpression of Tert, the protein component of telomerase, promoted stem cell mobilization, hair growth, and stem cell proliferation Citation39,40. This function does not require the telomerase RNA component, which encodes the template for telomere addition, and therefore operates through a novel mechanism independent of its activity in synthesizing telomere repeats.
Ectopic expression of telomerase has been used to immortalize various human cell types, and ectopic expression of the telomerase catalytic subunit in combination with two oncogenes results in direct tumorigenic conversion of normal human epithelial and fibroblast cells Citation41,42. We reported the establishment of epidermolysis bullosa simplex (EBS) keratinocytes with an extended life span by telomerase expression Citation43. After immortalization the cells retain normal growth and differentiation characteristics such as the ability to generate an epidermis with normal morphology in organ cultures and also a normal karyotype.
Direct transfer of genes into the skin
Genetic material—in the form of both viral and nonviral vectors—can be transferred directly to the skin. Naked DNA applied topically onto the skin leads to gene expression, but the expression is transient and at low levels Citation44. Plasmid DNA in various liposomal spray formulations sprayed onto mouse or human skin likewise leads to gene expression, but at low levels Citation45. A novel gene transfer method based on biphasic lipid vesicles is reported to enhance DNA transfer through intact stratum corneum Citation46. Higher levels of gene expression after direct delivery can be obtained using intracutaneous injection of DNA, liposomes, a gene gun or electroporation (reviewed in Citation27).
For prolonged gene expression in epidermis, integration into the genome of stem cells is required. Both retro‐ and lentiviral vectors are efficient for integration into cultured cells, including stem cells, and after direct injection both vectors result in long‐term gene expression in vivoCitation13,Citation47. Vectors based on adeno‐associated virus (AAV) have also been evaluated for in vivo application. Subcutaneous injection of AAV leads to long‐term expression of human factor IX, erythropoietin, and interferon Citation48, and intracutaneous injection of an AAV vector leads to gene expression for more than 6 weeks with expression in keratinocytes, both in the hair follicle and in eccrine sweat glands Citation49.
Viral and nonviral gene transfer methods may be combined. Retroviral vectors were produced in tissue‐cultured primary human keratinocytes after DNA‐mediated gene transfer followed by transduction of cocultured keratinocytes and prolonged reporter gene expression Citation50. This method was evaluated in a pig model using selection for transduced keratinocytes (employing MDR as selective marker) (unpublished data).
The ‘metabolic sink’ approach
Several studies investigated the capacity for genetically modified keratinocytes or skin fibroblasts to metabolize toxic compounds accumulating in various inborn errors of metabolism (reviewed in Citation51). Both fibroblasts and keratinocytes were able to produce significant amounts of adenosine deaminase (ADA) and metabolize deoxyadenosine and adenosine (accumulating in ADA deficiency), but it was calculated that blood supply would be rate‐limiting for clinical applications Citation2,Citation10. Keratinocytes from patients with ornithine aminotransferase deficiency (OAT deficiency) overexpressing OAT have a large capacity for protein expression, and ornithine uptake and metabolism was increased to supranormal levels Citation52,53. We used similar strategies to investigate the possibility of using the skin for gene therapy of phenylketonuria (PKU) caused by deficiency of phenylalanine hydroxylase (PAH) and increased levels of phenylalanine Citation54. The PAH enzyme requires a cofactor (BH4), and the rate‐limiting step in the synthesis of BH4 is GTP cyclohydrolase I (GTP‐CH). In tissue culture we showed that primary human keratinocytes and patient fibroblasts overexpressing PAH and GTP‐CH were able to metabolize phenylalanine. Transgenic mice expressing these two enzymes under control of skin‐specific promoters were cross‐bred to PKU mice to obtain mice only expressing the enzymes in the skin Citation55. Homozygous PKU mice having expression of the enzymes in the basal layer of epidermis (driven by the K14 promoter) had statistically significant decreased levels of serum phenylalanine compared to control homozygous mice without the transgenes. However, the decrease was only minor showing that the system should be developed further. Additional steps in the clearance pathway—both transport of phenylalanine into the cells and downstream processes—were subsequently manipulated in tissue culture in order to enhance the clearance of phenylalanine Citation56. These results suggested that enzymes other than GTP‐CH involved in BH4 synthesis or regeneration are rate‐limiting, such as 6‐pyruvoel‐tetrahydrobiopterin synthase (PTPS) or 4a‐carbinolamine dehydratase (PCD). Interestingly, in the muscle tissue, recent data show that expression of PTPS, in addition to PAH and GTP‐CH, leads to very high levels of phenylalanine clearance (Beat Thony, personal communication).
Wound healing
Non‐healing wounds represent a major clinical problem. Several strategies have been investigated with the purpose of promoting wound healing by use of gene transfer, including stimulation of the granulation process, the vascularization, the reepithelialization or the scar quality Citation57,58. Recent progress in this field includes transient expression of human host defense peptides, and gene transfer of multiple genes to improve the wound healing process Citation59–61. One of the major regulators of tissue repair in the skin is platelet‐derived growth factor (PDGF) Citation62. This protein is produced at the injury site, promotes neovascularization, and stimulates dermal fibroblasts to proliferate, migrate, and synthesize extracellular matrix components. Normally the protein has no direct effect on keratinocytes since these cells do not have PDGF receptors. We showed that expression of human PDGF receptors can be achieved in epidermal keratinocytes by retroviral transduction, and that ligand activation of such gene‐modified skin equivalent enhances cell proliferation Citation63. Gene transfer resulting in the production of both PDGF A and B has been described to enhance the wound healing process, and, thus, it may be of advantage to make adjacent epidermis sensitive to PDGF stimuli.
Gene editing
Specific repair of disease‐causing mutations can be achieved using several methods. One strategy that has been used in the skin is based on oligonucleotides that stimulate the correction of single‐base mutations in mammalian cells Citation64,65. In cultured melanocytes a point mutation in the tyrosinase gene was corrected resulting in restoration of tyrosinase enzymatic activity, melanin synthesis, and pigmentation Citation66. Likewise, topical application and intradermal injection of the oligonucleotides to albino mouse skin resulted in dark pigmentation of several hairs in a localized area Citation67. Gene repair in primary human keratinocytes may be more difficult by these methods Citation68. Thus, a better understanding of the underlying mechanisms of the gene conversion process may help to increase the targeting frequency, and the promising repair technique based on artificial zinc finger proteins is worth trying for cutaneous applications Citation69,70. In epidermolysis bullosa simplex, caused by keratin mutants expressed in basal cells, gene‐corrected keratinocytes from the basal layer will have a growth advantage Citation71. Thus strategies aiming at correcting keratin mutations in keratinocytes are indeed worth pursuing.
Gene therapy of inherited skin diseases
Numerous experiments aiming at the development of gene therapy of inherited skin diseases have been described, and a clinical trial was initiated in Italy involving ex vivo retroviral mediated transfer of the β3 gene of laminin‐5 to patients with junctional epidermolysis bullosa (JEB) Citation72,73. This disease is caused by defects in proteins important in the adhesion of basal layer cells to the dermis, including laminin‐5, a major basal lamina component formed by three distinct polypeptides, α3, β3, and γ2. Stable transduction of JEB patient stem cells, long‐term expression in vivo, and complete phenotypic correction of mutated keratinocytes, combined with the facts that corrected cells are more adherent to the basal lamina, and thus may have a selective advantage in vivo, led to trial.
Several research groups have focused on the development of gene therapy of recessive dystrophic epidermolysis bullosa (RDEB). This disease is caused by mutations in the COL7A1 gene that codes for the epidermal adhesion protein collagen VII. PhiC31 bacteriophage integrase was used to integrate COL7A1 cDNA into keratinocytes from RDEB patients and normalize the disease phenotype Citation74. Intradermal injection of RDEB fibroblasts overexpressing type VII collagen into intact RDEB skin is another strategy that stably restored correctly localized type VII collagen expression in vivoCitation75. Finally, injection of lentiviral vectors expressing human type VII collagen transduced dermal cells, which synthesized and exported type VII collagen into the extracellular space and reversed the RDEB phenotype Citation76.
Xeroderma pigmentosum (XP) is an inherited disease associated with increased risk for the development of skin tumors in sun‐exposed areas. Impaired nucleotide excision repair is deficient leading to ultraviolet‐induced hypermutagenesis and, thus, predisposition to cancer. In tissue culture, the phenotype of XP keratinocytes was normalized after retrovirus‐mediated transfer of the adequate wild‐type XP gene Citation77, but even more impressing, recombinant adenovirus carrying a human XPA gene was used for in vivo gene therapy in UVB‐irradiated skin of XP‐mutant mice, and here injected virus prevented development of squamous cell carcinoma Citation78. Gene delivery to the skin is therefore a promising tool for reconstitution of specific DNA repair defects in XP patients.
Hair follicle gene therapy
As mentioned previously, hair follicle stem cells are highly pluripotent and can form neurons, glial cells, and other cell types, and it was suggested that hair follicle stem cells may serve as general gene therapy targets for regenerative medicine Citation79.
Retroviral vectors encoding reporter genes have been used to transduce cultured hair follicle stem cells. After skin reconstitution, transgene expression was observed in all skin epithelial compartments including the hair follicle epithelium, sebaceous gland, and epidermis. In addition, transgene expression was observed for at least 6 months Citation80.
The protein Sonic hedgehog (Shh) is involved in the initiation of hair growth. After adenoviral mediated expression of the protein in the skin after direct injection, it was found that the hair follicle size increased and that there was a marked acceleration of the onset of new hair growth in the region of adenovirus administration Citation81. It was suggested that the strategy may be beneficial in the treatment of some forms of alopecia associated with chemotherapy Citation82.
Immune reactions
The skin is a very immunologically active tissue, and gene transfer to skin has been shown to induce transgene‐specific immune responses (reviewed in Citation83). Ex vivo gene transfer approaches, where keratinocytes are transduced in culture and transplanted back to patients, may avoid signals provided to the immune system by in vivo administration of vectors Citation84. It was shown that activation of transgene‐specific immune responses in ex vivo gene transfer targeted to keratinocytes requires cross‐presentation of transgene product to antigen‐presenting cells. This process is amenable to immune modulation, and thus immunological reactions may be circumvented using this strategy.
Gene transfer into the skin has elicited effective immune responses against a large variety of different infectious agents and tumor antigens in animal models (reviewed in Citation85. DNA transfer into the skin can lead to tumor regression by enhancing the tumor‐directed immune response, and cytokine genes may be delivered directly to a tumor to stimulate destruction by the host immune system Citation86–90. Several cell types that are present in the skin microenvironment, including keratinocytes in epidermis and fibroblasts in dermis, have the capacity to secrete cytokines that initiate and control immune responses Citation91. Furthermore, the skin has resident epidermal and dermal dendritic cells, a very potent (‘professional’) antigen‐presenting cell. Transfected dendritic cells have been shown to present transgenic peptides through both MHC class I‐ and II‐restricted pathways Citation92,93. In addition, this cell type is able to take up proteins from transfected neighboring cells in the case of secreted proteins or by phagocytosis of necrotic or apoptotic cells Citation94,95. Both of these mechanisms can induce humoral and cellular immune responses.
Conclusions
For gene therapy applications, the skin, the largest organ in the body, offers several advantages compared to other organs including easy accessibility, well understood biology, and safety parameters. Important factors arguing for continued focus on this field include controlled gene expression, 100% targeting of cultured cells in vitro including stem cells, prolonged gene expression even after in vivo applications, and excellent transplantation methods. Thus cutaneous gene therapy will remain a potential approach not only for local diseases but also in the future most likely for systemic diseases. Development of vectors allowing topical gene transfer, efficient targeting of long‐lived cells and regulated gene expression will pave the way for the treatment of diseases requiring short‐term or long‐term effects.
Figure 2. Combined viral and nonviral gene transfer into human keratinocytes performed as previously describedCitation50. a Monolayer cultures of human keratinocytes. The cells were transfected with a retroviral construct containing the LDL receptor gene (left panel) or cotransfected with the same construct and the pPAM3 plasmid containing gag pol and env genes (right panel). The LDL receptor protein was visualized using immunostaining at the indicated time points after transfection. (Green = FITC‐labeled LDL receptor protein; red = propidium iodine‐staining of nuclei) for colours please see (50). After cotransfection the cells produce retroviral vectors that subsequently transduce 100% of the cells. b Raft cultures of human keratinocytes cultured in the liquid‐air interphase. The cells were transfected in monolayer with a retroviral construct containing a GFP gene and the pPAM3 plasmid containing gag pol and env genes and subsequently induced to differentiate to a multilayered tissue. GFP protein was visualized using immunostaining as described Citation50. The anti‐GFP antibody was visualized using peroxidase‐staining leading to the brown color. GFP‐positive cells are found in all layers of the tissue. In control cultures transfected with the GFP construct alone no GFP‐positive cells were found at this time point (13 days after transfection) (data not shown).
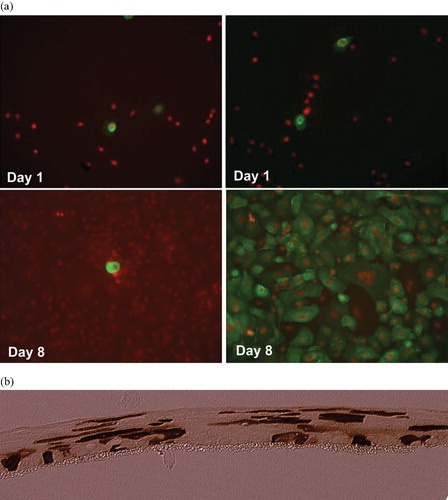
References
- Morgan J. R., Barrandon Y., Green H., Mulligan R. C. Expression of an exogenous growth hormone gene by transplantable human epidermal cells. Science 1987; 237: 1476–9
- Palmer T. D., Hock R. A., Osborne W. R., Miller A. D. Efficient retrovirus‐mediated transfer and expression of a human adenosine deaminase gene in diploid skin fibroblasts from an adenosine deaminase‐deficient human. Proc Natl Acad Sci U S A 1987; 84: 1055–9
- Anson D. S., Hock R. A., Austen D., Smith K. J., Brownlee G. G., Verma I. M., et al. Towards gene therapy for hemophilia B. Mol Biol Med 1987; 4: 11–20
- Hengge U. R. Progress and prospects of skin gene therapy: a ten year history. Clin Dermatol 2005; 23: 107–14
- Khavari P. A., Rollman O., Vahlquist A. Cutaneous gene transfer for skin and systemic diseases. J Intern Med 2002; 252: 1–10
- Christensen R., Jensen U. B., Jensen T. G. Cutaneous gene therapy—an update. Histochem Cell Biol 2001; 115: 73–82
- Palmer T. D., Rosman G. J., Osborne W. R., Miller A. D. Genetically modified skin fibroblasts persist long after transplantation but gradually inactivate introduced genes. Proc Natl Acad Sci U S A 1991; 88: 1330–4
- Jensen U. B., Jensen T. G., Jensen P. K., Rygaard J., Hansen B. S., Fogh J., et al. Gene transfer into cultured human epidermis and its transplantation onto immunodeficient mice: an experimental model for somatic gene therapy. J Invest Dermatol 1994; 103: 391–4
- Fenjves E. S., Yao S. N., Kurachi K., Taichman L. B. Loss of expression of a retrovirus‐transduced gene in human keratinocytes. J Invest Dermatol 1996; 106: 576–8
- Fenjves E. S., Schwartz P. M., Blaese R. M., Taichman L. B. Keratinocyte gene therapy for adenosine deaminase deficiency: a model approach for inherited metabolic disorders. Hum Gene Ther 1997; 8: 911–7
- Katz A. B., Taichman L. B. Epidermis as a secretory tissue: an in vitro tissue model to study keratinocyte secretion. J Invest Dermatol 1994; 102: 55–60
- Larcher F., Del Rio M., Serrano F., Segovia J. C., Ramirez A., Meana A., et al. A cutaneous gene therapy approach to human leptin deficiencies: correction of the murine ob/ob phenotype using leptin‐targeted keratinocyte grafts. FASEB J 2001; 15: 1529–38
- Baek S. C., Lin Q., Robbins P. B., Fan H., Khavari P. A. Sustainable systemic delivery via a single injection of lentivirus into human skin tissue. Hum Gene Ther 2001; 12: 1551–8
- Fakharzadeh S. S., Zhang Y., Sarkar R., Kazazian H. H., Jr. Correction of the coagulation defect in hemophilia A mice through factor VIII expression in skin. Blood 2000; 95: 2799–805
- Gerrard A. J., Hudson D. L., Brownlee G. G., Watt F. M. Towards gene therapy for haemophilia B using primary human keratinocytes. Nat Genet 1993; 3: 180–3
- Hengge U. R., Chan E. F., Foster R. A., Walker P. S., Vogel J. C. Cytokine gene expression in epidermis with biological effects following injection of naked DNA. Nat Genet 1995; 10: 161–6
- Meng X., Sawamura D., Tamai K., Hanada K., Ishida H., Hashimoto I. Keratinocyte gene therapy for systemic diseases. Circulating interleukin 10 released from gene‐transferred keratinocytes inhibits contact hypersensitivity at distant areas of the skin. J Clin Invest 1998; 101: 1462–7
- Noel D., Dazard J. E., Pelegrin M., Jacquet C., Piechaczyk M. Skin as a potential organ for ectopic monoclonal antibody production. J Invest Dermatol 2002; 118: 288–94
- Carretero M., Del Rio M., Garcia M., Escamez M. J., Mirones I., Rivas L., et al. A cutaneous gene therapy approach to treat infection through keratinocyte‐targeted overexpression of antimicrobial peptides. FASEB J 2004; 18: 1931–3
- Yao F., Eriksson E. A novel tetracycline‐inducible viral replication switch. Hum Gene Ther 1999; 10: 419–27
- Freiberg R. A., Ho S. N., Khavari P. A. Transcriptional control in keratinocytes and fibroblasts using synthetic ligands. J Clin Invest 1997; 99: 2610–5
- Gandarillas A., Watt F. M. c‐Myc promotes differentiation of human epidermal stem cells. Genes Dev 1997; 11: 2869–82
- Cao T., Tsai S. Y., O'Malley B. W., Wang X. J., Roop D. R. The epidermis as a bioreactor: topically regulated cutaneous delivery into the circulation. Hum Gene Ther 2002; 13: 1075–80
- Ghazizadeh S., Harrington R., Garfield J., Taichman L. B. Retrovirus‐mediated transduction of porcine keratinocytes in organ culture. J Invest Dermatol 1998; 111: 492–6
- Pfutzner W., Terunuma A., Tock C. L., Snead E. K., Kolodka T. M., Gottesman M. M., et al. Topical colchicine selection of keratinocytes transduced with the multidrug resistance gene (MDR1) can sustain and enhance transgene expression in vivo. Proc Natl Acad Sci U S A 2002; 99: 13096–101
- Deng H., Choate K. A., Lin Q., Khavari P. A. High‐efficiency gene transfer and pharmacologic selection of genetically engineered human keratinocytes. Biotechniques 1998; 25: 274–80
- Jensen T. G. Gene transfer into human epidermis as an experimental model for somatic gene therapy. Dan Med Bull 2004; 51: 155–66
- Bajaj B. G., Lei P., Andreadis S. T. Efficient gene transfer to human epidermal keratinocytes on fibronectin: in vitro evidence for transduction of epidermal stem cells. Mol Ther 2005; 11: 969–79
- Jensen U. B., Lowell S., Watt F. M. The spatial relationship between stem cells and their progeny in the basal layer of human epidermis: a new view based on whole‐mount labelling and lineage analysis. Development 1999; 126: 2409–18
- Watt F. M. The stem cell compartment in human interfollicular epidermis. J Dermatol Sci 2002; 28: 173–80
- Cotsarelis G. Epithelial stem cells: a folliculocentric view. J Invest Dermatol 2006; 126: 1459–68
- Ito M., Liu Y., Yang Z., Nguyen J., Liang F., Morris R. J., et al. Stem cells in the hair follicle bulge contribute to wound repair but not to homeostasis of the epidermis. Nat Med 2005; 11: 1351–4
- Hoeller D., Huppertz B., Roos T. C., Poblete Gutierrez P., Merk H. F., Frank J., et al. An improved and rapid method to construct skin equivalents from human hair follicles and fibroblasts. Exp Dermatol 2001; 10: 264–71
- Navsaria H. A., Ojeh N. O., Moiemen N., Griffiths M. A., Frame J. D. Reepithelialization of a full‐thickness burn from stem cells of hair follicles micrografted into a tissue‐engineered dermal template (Integra). Plast Reconstr Surg 2004; 113: 978–81
- Hoffman R. M. The hair follicle and its stem cells as drug delivery targets. Expert Opin Drug Deliv 2006; 3: 437–43
- Amoh Y., Li L., Katsuoka K., Penman S., Hoffman R. M. Multipotent nestin‐positive, keratin‐negative hair‐follicle bulge stem cells can form neurons. Proc Natl Acad Sci U S A 2005; 102: 5530–4
- Flores I., Benetti R., Blasco M. A. Telomerase regulation and stem cell behaviour. Curr Opin Cell Biol 2006; 18: 254–60
- Bickenbach J. R., Vormwald‐Dogan V., Bachor C., Bleuel K., Schnapp G., Boukamp P. Telomerase is not an epidermal stem cell marker and is downregulated by calcium. J Invest Dermatol 1998; 111: 1045–52
- Sarin K. Y., Cheung P., Gilison D., Lee E., Tennen R. I., Wang E., et al. Conditional telomerase induction causes proliferation of hair follicle stem cells. Nature 2005; 436: 1048–52
- Flores I., Cayuela M. L., Blasco M. A. Effects of telomerase and telomere length on epidermal stem cell behavior. Science 2005; 309: 1253–6
- Hahn W. C., Counter C. M., Lundberg A. S., Beijersbergen R. L., Brooks M. W., Weinberg R. A. Creation of human tumour cells with defined genetic elements. Nature 1999; 400: 464–8
- Bodnar A. G., Ouellette M., Frolkis M., Holt S. E., Chiu C. P., Morin G. B., et al. Extension of life‐span by introduction of telomerase into normal human cells. Science 1998; 279: 349–52
- Jensen T. G., Sorensen C. B., Jensen U. B., Bolund L. Epidermolysis Bullosa Simplex Keratinocytes with Extended Lifespan established by ectopic Expression of Telomerase. Exp Dermatol 2003; 12: 71–7
- Fan H., Lin Q., Morrissey G. R., Khavari P. A. Immunization via hair follicles by topical application of naked DNA to normal skin. Nat Biotechnol 1999; 17: 870–2
- Meykadeh N., Mirmohammadsadegh A., Wang Z., Basner‐Tschakarjan E., Hengge U. R. Topical application of plasmid DNA to mouse and human skin. J Mol Med 2005; 83: 897–903
- Foldvari M., Kumar P., King M., Batta R., Michel D., Badea I., et al. Gene delivery into human skin in vitro using biphasic lipid vesicles. Curr Drug Deliv 2006; 3: 89–93
- Ghazizadeh S., Harrington R., Taichman L. In vivo transduction of mouse epidermis with recombinant retroviral vectors: implications for cutaneous gene therapy. Gene Ther 1999; 6: 1267–75
- Donahue B. A., McArthur J. G., Spratt S. K., Bohl D., Lagarde C., Sanchez L., et al. Selective uptake and sustained expression of AAV vectors following subcutaneous delivery. J Gene Med 1999; 1: 31–42
- Hengge U. R., Mirmohammadsadegh A. Adeno‐associated virus expresses transgenes in hair follicles and epidermis. Mol Ther 2000; 2: 188–94
- Jensen T. G., Jensen U. B., Bolund L. Production of retroviral vectors in primary human keratinocytes after DNA‐mediated gene transfer leads to prolonged gene expression. Acta Derm Venereol 2003; 83: 83–7
- Jensen T. G. Strategies for long‐term gene expression in the skin to treat metabolic disorders. Expert Opin Biol Ther 2004; 4: 677–82
- Sullivan D. M., Jensen T. G., Taichman L. B., Csaky K. G. Ornithine‐delta‐aminotransferase expression and ornithine metabolism in cultured epidermal keratinocytes: toward metabolic sink therapy for gyrate atrophy. Gene Ther 1997; 4: 1036–44
- Jensen T. G., Sullivan D. M., Morgan R. A., Taichman L. B., Nussenblatt R. B., Blaese R. M., et al. Retrovirus‐mediated gene transfer of ornithine‐delta‐aminotransferase into keratinocytes from gyrate atrophy patients. Hum Gene Ther 1997; 8: 2125–32
- Christensen R., Kolvraa S., Blaese R. M., Jensen T. G. Development of a skin‐based metabolic sink for phenylalanine by overexpression of phenylalanine hydroxylase and GTP cyclohydrolase in primary human keratinocytes. Gene Ther 2000; 7: 1971–8
- Christensen R., Alhonen L., Wahlfors J., Jakobsen M., Jensen T. G. Characterization of transgenic mice with the expression of phenylalanine hydroxylase and GTP cyclohydrolase I in the skin. Exp Dermatol 2005; 14: 535–42
- Christensen R., Kolvraa S., Jensen T. G. Manipulation of the phenylalanine metabolism in human keratinocytes by retroviral mediated gene transfer. Cells Tissues Organs 2005; 179: 170–8
- Petrie N. C., Vranckx J. J., Hoeller D., Yao F., Eriksson E. Gene delivery of PDGF for wound healing therapy. J Tissue Viability 2005; 15: 16–21
- Rogers B., Lineaweaver W. C. Skin wound healing and cell‐mediated DNA transport. J Long Term Eff Med Implants 2002; 12: 125–30
- Jacobsen F., Mittler D., Hirsch T., Gerhards A., Lehnhardt M., Voss B., et al. Transient cutaneous adenoviral gene therapy with human host defense peptide hCAP‐18/LL‐37 is effective for the treatment of burn wound infections. Gene Ther 2005; 12: 1494–502
- Liu P. Y., Liu K., Wang X. T., Badiavas E., Rieger‐Christ K. M., Tang J. B., et al. Efficacy of combination gene therapy with multiple growth factor cDNAs to enhance skin flap survival in a rat model. DNA Cell Biol 2005; 24: 751–7
- Jeschke M. G., Klein D. Liposomal gene transfer of multiple genes is more effective than gene transfer of a single gene. Gene Ther 2004; 11: 847–55
- Moulin V. Growth factors in skin wound healing. Eur J Cell Biol 1995; 68: 1–7
- Rollman O., Jensen U. B., Ostman A., Bolund L., Gustafsdottir S. M., Jensen T. G. Platelet derived growth factor (PDGF) responsive epidermis formed from human keratinocytes transduced with the PDGF beta receptor gene. J Invest Dermatol 2003; 120: 742–9
- Yoon K., Cole‐Strauss A., Kmiec E. B. Targeted gene correction of episomal DNA in mammalian cells mediated by a chimeric RNA.DNA oligonucleotide. Proc Natl Acad Sci U S A 1996; 93: 2071–6
- Andersen M. S., Sorensen C. B., Bolund L., Jensen T. G. Mechanisms underlying targeted gene correction using chimeric RNA/DNA and single‐stranded DNA oligonucleotides. J Mol Med 2002; 80: 770–81
- Alexeev V., Yoon K. Stable and inheritable changes in genotype and phenotype of albino melanocytes induced by an RNA‐DNA oligonucleotide. Nat Biotechnol 1998; 16: 1343–6
- Alexeev V., Igoucheva O., Domashenko A., Cotsarelis G., Yoon K. Localized in vivo genotypic and phenotypic correction of the albino mutation in skin by RNA‐DNA oligonucleotide. Nat Biotechnol 2000; 18: 43–7
- Santana E., Peritz A. E., Iyer S., Uitto J., Yoon K. Different frequency of gene targeting events by the RNA‐DNA oligonucleotide among epithelial cells. J Invest Dermatol 1998; 111: 1172–7
- Urnov F. D., Miller J. C., Lee Y. L., Beausejour C. M., Rock J. M., Augustus S., et al. Highly efficient endogenous human gene correction using designed zinc‐finger nucleases. Nature 2005; 435: 646–51
- Porteus M. H., Carroll D. Gene targeting using zinc finger nucleases. Nat Biotechnol 2005; 23: 967–73
- Cao T., Longley M. A., Wang X. J., Roop D. R. An inducible mouse model for epidermolysis bullosa simplex: implications for gene therapy. J Cell Biol 2001; 152: 651–6
- Ferrari S., Pellegrini G., Matsui T., Mavilio F., De Luca M. Gene therapy in combination with tissue engineering to treat epidermolysis bullosa. Expert Opin Biol Ther 2006; 6: 367–78
- Ferrari S., Pellegrini G., Mavilio F., De Luca M. Gene therapy approaches for epidermolysis bullosa. Clin Dermatol 2005; 23: 430–6
- Ortiz‐Urda S., Thyagarajan B., Keene D. R., Lin Q., Fang M., Calos M. P., et al. Stable nonviral genetic correction of inherited human skin disease. Nat Med 2002; 8: 1166–70
- Ortiz‐Urda S., Lin Q., Yant S. R., Keene D., Kay M. A., Khavari P. A. Sustainable correction of junctional epidermolysis bullosa via transposon‐mediated nonviral gene transfer. Gene ther 2003; 10: 1099–104
- Chen M., Kasahara N., Keene D. R., Chan L., Hoeffler W. K., Finlay D., et al. Restoration of type VII collagen expression and function in dystrophic epidermolysis bullosa. Nat Genet 2002; 32: 670–5
- Arnaudeau‐Begard C., Brellier F., Chevallier‐Lagente O., Hoeijmakers J., Bernerd F., Sarasin A., et al. Genetic correction of DNA repair‐deficient/cancer‐prone xeroderma pigmentosum group C keratinocytes. Hum Gene Ther 2003; 14: 983–96
- Marchetto M. C., Muotri A. R., Burns D. K., Friedberg E. C., Menck C. F. Gene transduction in skin cells: preventing cancer in xeroderma pigmentosum mice. Proc Natl Acad Sci U S A 2004; 101: 17759–64
- Domashenko A., Gupta S., Cotsarelis G. Efficient delivery of transgenes to human hair follicle progenitor cells using topical lipoplex. Nat Biotechnol 2000; 18: 420–3
- Sugiyama‐Nakagiri Y., Akiyama M., Shimizu H. Hair follicle stem cell‐targeted gene transfer and reconstitution system. Gene Ther 2006; 13: 732–7
- Sato N., Leopold P. L., Crystal R. G. Induction of the hair growth phase in postnatal mice by localized transient expression of Sonic hedgehog. J Clin Invest 1999; 104: 855–64
- Sato N., Leopold P. L., Crystal R. G. Effect of adenovirus‐mediated expression of Sonic hedgehog gene on hair regrowth in mice with chemotherapy‐induced alopecia. J Natl Cancer Inst 2001; 93: 1858–64
- Hengge U. R., Bardenheuer W. Gene therapy and the skin. Am J Med Genet C Semin Med Genet 2004; 131C: 93–100
- Lu Z., Ghazizadeh S. Host immune responses in ex vivo approaches to cutaneous gene therapy targeted to keratinocytes. Exp Dermatol 2005; 14: 727–35
- Larregina A. T., Falo L. D., Jr. Generating and regulating immune responses through cutaneous gene delivery. Hum Gene Ther 2000; 11: 2301–5
- Rakhmilevich A. L., Turner J., Ford M. J., McCabe D., Sun W. H., Sondel P. M., et al. Gene gun‐mediated skin transfection with interleukin 12 gene results in regression of established primary and metastatic murine tumors. Proc Natl Acad Sci U S A 1996; 93: 6291–6
- Sun W. H., Burkholder J. K., Sun J., Culp J., Turner J., Lu X. G., et al. In vivo cytokine gene transfer by gene gun reduces tumor growth in mice. Proc Natl Acad Sci U S A 1995; 92: 2889–93
- Nabel G. J., Nabel E. G., Yang Z. Y., Fox B. A., Plautz G. E., Gao X., et al. Direct gene transfer with DNA‐liposome complexes in melanoma: expression, biologic activity, and lack of toxicity in humans. Proc Natl Acad Sci U S A 1993; 90: 11307–11
- Nabel G. J., Gordon D., Bishop D. K., Nickoloff B. J., Yang Z. Y., Aruga A., et al. Immune response in human melanoma after transfer of an allogeneic class I major histocompatibility complex gene with DNA‐liposome complexes. Proc Natl Acad Sci U S A 1996; 93: 15388–93
- Quintin‐Colonna F., Devauchelle P., Fradelizi D., Mourot B., Faure T., Kourilsky P., et al. Gene therapy of spontaneous canine melanoma and feline fibrosarcoma by intratumoral administration of histoincompatible cells expressing human interleukin‐2. Gene Ther 1996; 3: 1104–12
- Peachman K. K., Rao M., Alving C. R. Immunization with DNA through the skin. Methods 2003; 31: 232–42
- Condon C., Watkins S. C., Celluzzi C. M., Thompson K., Falo L. D., Jr. DNA‐based immunization by in vivo transfection of dendritic cells. Nat Med 1996; 2: 1122–8
- Porgador A., Irvine K. R., Iwasaki A., Barber B. H., Restifo N. P., Germain R. N. Predominant role for directly transfected dendritic cells in antigen presentation to CD8+ T cells after gene gun immunization. J Exp Med 1998; 188: 1075–82
- Celluzzi C. M., Falo L. D., Jr. Physical interaction between dendritic cells and tumor cells results in an immunogen that induces protective and therapeutic tumor rejection. J Immunol 1998; 160: 3081–5
- Albert M. L., Sauter B., Bhardwaj N. Dendritic cells acquire antigen from apoptotic cells and induce class I‐restricted CTLs. Nature 1998; 392: 86–9