Abstract
Prolyl 4-hydroxylases (P4Hs) have central roles in the synthesis of collagens and the regulation of oxygen homeostasis. The 4-hydroxyproline residues generated by the endoplasmic reticulum (ER) luminal collagen P4Hs (C-P4Hs) are essential for the stability of the collagen triple helix. Vertebrate C-P4Hs are α2β2 tetramers with three isoenzymes differing in their catalytic α subunits. Another P4H family, the HIF-P4Hs, hydroxylates specific prolines in the α subunit of the hypoxia-inducible transcription factor (HIF), a master regulator of hypoxia-inducible genes, and controls its stability in an oxygen-dependent manner. The HIF-P4Hs are cytoplasmic and nuclear enzymes, likewise with three isoenzymes in vertebrates. A third vertebrate P4H type is an ER transmembrane protein that can act on HIF-α but not on collagens. All P4Hs require Fe2+, 2-oxoglutarate, O2, and ascorbate. C-P4Hs are regarded as attractive targets for pharmacological inhibition to control excessive collagen accumulation in fibrotic diseases and severe scarring, while HIF-P4H inhibitors are believed to have beneficial effects in the treatment of diseases such as myocardial infarction, stroke, peripheral vascular disease, diabetes, and severe anemias. Studies with P4H inhibitors in various animal models of fibrosis, anemia, and ischemia and ongoing clinical trials with HIF-P4H inhibitors support this hypothesis by demonstrating efficacy in many applications.
Introduction
Most of the 4-hydroxyproline in mammalian proteins is found in the -X-4Hyp-Gly- sequences of the 29 currently known collagen types and more than 20 additional proteins with collagen-like triple-helical domains Citation1–4. 4-Hydroxyproline residues have a vital role in providing the collagen triple helices with thermal stability (). Non-hydroxylated collagen polypeptide chains cannot form functional molecules in vivo, and almost complete hydroxylation of the proline residues in -X-Pro-Gly- triplets is required for the generation of a molecule that is stable at human body temperature. This hydroxylation is catalyzed by collagen prolyl 4-hydroxylases (C-P4Hs) located within the lumen of the endoplasmic reticulum (ER). The mammalian C-P4Hs are α2β2 tetramers in which the β subunit is identical to the enzyme and chaperone protein disulfide isomerase (PDI) (for reviews, see Citation5–8). Three C-P4H isoenzymes differing in their catalytic α subunit have been identified and characterized from human, mouse, and rat tissues Citation5–10. C-P4H families have also been identified in other animal species, e.g. the nematode Caenorhabditis elegans has 4 C-P4H α subunit isoforms, and the fly Drosophila melanogaster has at least 19 C-P4H α subunit-like polypeptides Citation1, Citation5–8 (see also Citation11–15). The main functions of PDI as the C-P4H β subunit are to keep the highly insoluble α subunits in a catalytically active, non-aggregated conformation and to retain the C-P4Hs within the lumen of the ER via its C-terminal retention signal.
Abbreviations
Figure 1. Hydroxylation of proline residues by C-P4Hs is essential for the thermal stability of collagen triple helices. Non-hydroxylated collagen polypeptide chains cannot form functional molecules in vivo.
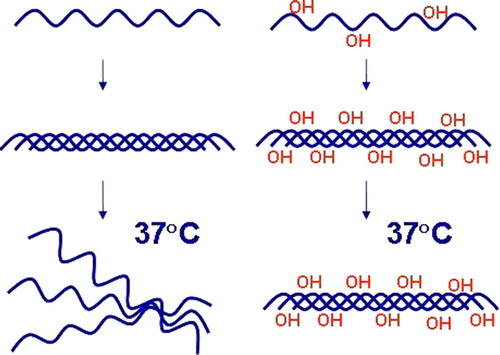
A novel role for 4-hydroxyproline was identified in 2001 in the hypoxia-inducible transcription factor (HIF) Citation16–18. HIF is an αβ heterodimer in which the stability of the α subunit is regulated in an oxygen-dependent manner. The HIF-α subunit is synthesized continuously, and one or two critical proline residues in two -Leu-X-X-Leu-Ala-Pro- sequences of its oxygen-dependent degradation domain (ODDD) are hydroxylated under normoxic conditions by a novel cytoplasmic and nuclear HIF-P4H family () Citation19–21. This family consists of three members in the human, mouse, and rat, while C. elegans and D. melanogaster have only a single HIF-P4H Citation19, Citation20, Citation22. The 4-hydroxyproline residues formed by the HIF-P4Hs are required for the binding of HIF-α to the von Hippel-Lindau (VHL) E3 ubiquitin ligase complex and its rapid subsequent proteasomal degradation in normoxia () (for reviews, see Citation23–27). Under hypoxic conditions this oxygen-requiring hydroxylation is inhibited, and HIF-α escapes degradation and dimerizes with HIF-β (). The dimer is then translocated into the nucleus and becomes bound to the HIF-responsive elements in more than 100 hypoxia-regulated genes that facilitate adaptation to hypoxia and associated metabolic compromise such as those involved in hematopoiesis, angiogenesis, iron transport, glucose utilization, resistance to oxidative stress, cell proliferation, survival and apoptosis, and tumor progression, including enzymes involved in collagen synthesis, namely C-P4Hs, lysyl hydroxylases and lysyl oxidases Citation23–33. The HIF-α also has three isoforms in humans Citation26, Citation27, Citation34. The closely related HIF-1α and HIF-2α isoforms regulate common target genes but also show specificity in their targets, in that HIF-1α appears to act more effectively on genes for glycolytic enzymes, for instance, and HIF-2α on the gene for erythropoietin (Epo) Citation30, Citation31, Citation35–38.
Key messages
Prolyl 4-hydroxylases (P4Hs) have essential roles in the synthesis of collagens and the regulation of oxygen homeostasis. The 4-hydroxyproline residues generated by collagen P4Hs (C-P4Hs) are essential for the stability of the collagen triple helix, while the 4-hydroxyproline residues generated on the hypoxia-inducible transcription factor (HIF) by HIF-P4Hs regulate its stability in an oxygen-dependent manner.
C-P4Hs are regarded as attractive targets for pharmacological inhibition to control excessive collagen accumulation in fibrotic diseases and severe scarring. HIF-P4H inhibitors are believed to have beneficial effects in the treatment of diseases such as myocardial infarction, stroke, peripheral vascular disease, diabetes, and severe anemias.
Figure 2. Regulation of the stability of the HIF-1α by oxygen-dependent prolyl 4-hydroxylation. Under normoxic conditions, HIF-1α is hydroxylated by HIF-P4Hs. Hydroxylation is required for binding of the VHL E3 ubiquitin ligase complex and for subsequent proteasomal degradation. Hypoxia inhibits the HIF-P4Hs, HIF-1α escapes degradation and forms a stable dimer with HIF-β. The dimer is translocated into the nucleus and binds to the HIF-responsive elements in a number of hypoxia-inducible genes.
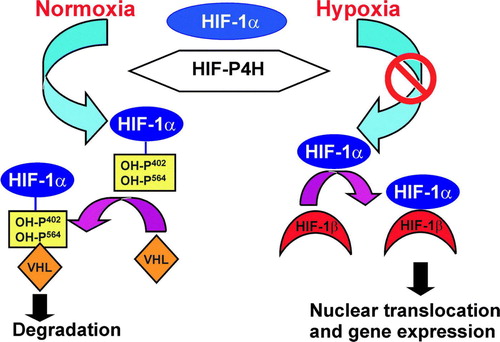
A further P4H possesses a transmembrane domain close to its N terminus (P4H-TM) and resembles the C-P4Hs more closely than the HIF-P4Hs in terms of its amino acid sequence and location in the ER membrane with its catalytic site inside the ER lumen Citation39, Citation40. It nevertheless resembles the HIF-P4Hs in that it hydroxylates HIF-αs in cultured cells and in vitro Citation39, Citation40.
All P4Hs belong to the 2-oxoglutarate-dependent dioxygenases, and their hydroxylation reaction requires Fe2+, 2-oxoglutarate, O2, and ascorbate and involves oxidative decarboxylation of 2-oxoglutarate Citation5–8, Citation23, Citation25–27. The C-P4Hs are regarded as potential targets for pharmacological inhibition to control excessive collagen accumulation in fibrotic diseases and severe scarring, while HIF-P4H inhibitors are believed to have beneficial effects in the treatment of diseases such as myocardial infarction, stroke, peripheral vascular disease, diabetes, and severe anemias. This review will concentrate on the molecular and functional properties of the human P4Hs and their potential as therapeutic targets.
Human C-P4Hs
The three human C-P4H α subunits assemble into α2β2 tetramers with PDI, the [α(I)]2β2, [α(II)]2β2, and [α(III)]2β2 tetramers being referred to as C-P4Hs I, II, and III, respectively Citation5–10. The vertebrate α subunits do not appear to form tetramers with dissimilar catalytic subunits Citation5–8, while in C. elegans a tetramer with two dissimilar catalytic subunits is the main C-P4H responsible for the hydroxylation of cuticle collagens Citation13. All attempts to assemble an active C-P4H tetramer from the dissociated subunits in vitro have been unsuccessful, but assembly of active recombinant C-P4Hs has been achieved by coexpression of the α subunit and PDI polypeptide both in mammalian cells and in insect, yeast, and plant cells and Escherichia coli Citation8, Citation41–44. This has enabled the development of high-level recombinant expression systems for hydroxylated human collagens in non-mammalian host cells Citation41, Citation42, Citation45–49.
Molecular properties of the catalytic α subunit
The three human α subunits consist of 514–525 amino acids () and are synthesized with a signal peptide of 17–21 additional residues. The overall sequence identity between the human α(I) and α(II) subunits is 65%, and those between α(I) and α(III) and between α(II) and α(III) are 35%–37% Citation5–9. The highest degree of identity is seen within the C-terminal regions, which contain the catalytically important amino acids (), the identity between the last 120 C-terminal residues of α(I) and α(II) being 80% and that between α(III) and the other two α subunits 56%–57% Citation5–9. The Fe2 + is bound by three residues of a conserved -His-X-Asp-…-His- motif, these being His412, Asp414, and His483 in the human α(I) subunit () Citation50. The C5 carboxyl group of 2-oxoglutarate is bound by a lysine residue, Lys493 in human α(I) () Citation50. The catalytic site is discussed further below.
Figure 3. Schematic representation of the human C-P4H α(I), α(II) and α(III) subunits, P4H-TM and HIF-P4Hs 1–3. The lengths of the polypeptides are indicated on the right, and the catalytically critical residues are shown above the polypeptides. The peptide-substrate- binding domain in the C-P4H α subunits and the TM domain of P4H-TM are also indicated.
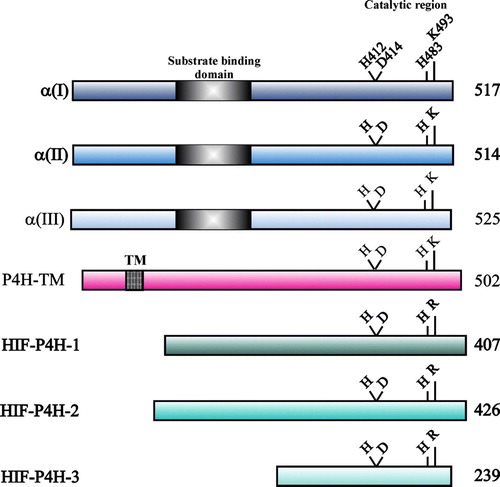
The human C-P4H α subunits have a peptide-substrate-binding domain that is separate from the C-terminal catalytic domain () Citation51, being located in the N-terminal half of the polypeptide and spanning approximately 100 residues. The peptide-binding properties of the various C-P4H isoenzymes can mostly be explained by the properties of binding to this domain rather than the catalytic domain Citation52. Crystallization of this domain has shown that it belongs to the family of tetratricopeptide repeat domains that are involved in many protein-protein interactions and possesses a deep groove lined by aromatic residues which appear to act as the binding site for proline-rich peptides Citation53.
The human α(I) and α(II) subunit mRNAs have two forms due to alternative splicing, whereas there is no evidence for alternative splicing of the α(III) transcripts Citation8, Citation9, Citation54. As both alternatively spliced forms of the α(I) and α(II) mRNAs are expressed in a variety of tissues, and as polypeptides corresponding to both forms assemble into active C-P4H tetramers, the potential biological significance of this alternative splicing remains to be deciphered.
C-P4Hs in tissues, diseases, and knock-out mouse models
C-P4H-I is the major form in most cell types and tissues, with C-P4H-II representing about 10%–30% of the total C-P4H activity in cultured human embryonic and adult skin and lung fibroblasts and fibrosarcoma cells, and 5%–15% in various chick embryo tissues Citation8. C-P4H-II is the main form in chondrocytes, osteoblasts, capillary endothelial cells, and some other cell types, however Citation8. C-P4H-II is therefore likely to play an important role in the development of cartilage, cartilaginous bone, and capillaries. The α(III) subunit mRNA is expressed in many adult and fetal human tissues but at much lower levels than the α(I) and α(II) mRNAs Citation9.
Excessive collagen formation plays a major role in the pathogenesis of fibrotic conditions, e.g. liver and pulmonary fibroses that interfere with the normal architecture and function of the affected tissue, and in abnormal wound healing characterized by severe scarring Citation55. The increased collagen synthesis is accompanied by increased amounts of C-P4H activity in various experimental fibrosis models and fibrotic diseases. It has also been shown that the expression of collagen II and C-P4H-II is increased in osteoarthritic articular chondrocytes relative to healthy chondrocytes Citation56.
No heritable human disease is known to be caused by mutations in any of the C-P4H α subunit genes. Knock-out mice have recently been generated for the C-P4H α(I) and α(II) subunits Citation1, Citation8, Citation57. The absence of C-P4H-I causes embryonic lethality, as α(I) null mice die at E10.5, showing an overall developmental delay and rupture of the basement membranes due to a lack of collagen IV Citation57. The level of C-P4H activity in the null embryos was about 20% of that in the wild type, this evidently being due to C-P4Hs II and III. α(II) null mice are viable and have no obvious phenotypic abnormalities Citation1, Citation8.
Human HIF-P4Hs
Identification of the HIF-P4Hs was facilitated by searches of the C. elegans and mammalian databases for sequences related to the C-P4H α subunits and having predicted β-barrel secondary structure motifs and catalytically critical residues that are common to all members of the 2-oxoglutarate dioxygenases Citation19, Citation20. The human HIF-P4Hs 1, 2, and 3 were originally named prolyl hydroxylase domain (PHD) enzymes 1, 2, and 3, Egl-nine (EGLN) 2, 1, and 3, and HIF prolyl hydroxylases (HPHs) 3, 2, and 1 Citation19–21, but for consistency in nomenclature with the C-P4Hs they are referred to as HIF-P4Hs in this review. In contrast to the C-P4Hs located within the lumen of the ER, the HIF-P4Hs are cytoplasmic and nuclear enzymes, HIF-P4H-2 being located mainly in the cytoplasm and HIF-P4H-1 exclusively in the nucleus, while HIF-P4H-3 is found in both cell compartments Citation58. Hypoxia does not influence the cellular location of the HIF-P4Hs Citation58.
Molecular properties
The HIF-P4H-1 and -2 polypeptides consist of 407 and 426 residues, while HIF-P4H-3 is considerably smaller, 239 residues () Citation19–21. The three isoenzymes have a 42%–59% sequence identity to each other but no significant sequence similarity to the C-P4H α subunits, except for the catalytically critical residues (). The Fe2 + -binding residues are provided by the -His-X-Asp-…-His- motif, being His297, Asp299, and His358 in HIF-P4H-1, while the residue binding the C5 carboxyl group of 2-oxoglutarate is an arginine in position +9 with respect to the second Fe2 + -binding histidine () Citation19–21. At least HIF-P4H-2 is likely to exist as a monomer in solution Citation59. The N-terminal region of HIF-P4H-2 contains a zinc finger domain that has been reported to inhibit its catalytic activity Citation60.
The HIF-P4H-2 and -3 mRNAs are subject to alternative splicing, resulting in at least two inactive variants of HIF-P4H-2 and one of HIF-P4H-3 Citation61, Citation62. These splicing forms are expressed in all tissues studied, the levels of mRNA encoding the inactive HIF-P4H-2 variants being considerably lower than that of the full-length mRNA, while the mRNA for the inactive HIF-P4H-3 variant is found in about equal amounts to the full-length one Citation61. A second, at least partially active, HIF-P4H-3 splice variant is restricted to primary cancer tissues Citation62. Furthermore, two HIF-P4H-1 forms are generated through alternative initiation, the shorter one having full catalytic activity but decreased stability Citation63. Changes in splicing pattern and initiation can thus affect the expression levels of active HIF-P4Hs and may thus add to the complexity of the HIF system.
HIF-P4Hs in tissues, diseases, and knock-out mouse models
The mRNAs for the three HIF-P4Hs are widely expressed in various vertebrate tissues and cell lines, but at different levels Citation61, Citation64–67. The HIF-P4H-2 mRNA has relatively uniform expression levels in all tissues studied, while HIF-P4H-1 has its highest expression in the placenta and is the only one detected in the testis, and HIF-P4H-3 has its highest expression in the heart Citation64, Citation65, Citation67. All three HIF-P4Hs are expressed in the kidney, higher levels being found in the medulla than in the cortex, and HIF-P4H-2 is the most abundant isoform Citation68.
HIF-P4H-2 is also the most abundant isoenzyme in most cultured cell types studied Citation66. Silencing of HIF-P4H-2 alone by short interfering RNA (siRNA) is sufficient to stabilize HIF-α in normoxia Citation66, Citation69, indicating that this isoenzyme is the major oxygen sensor setting low steady-state levels of HIF-α in normoxia, although each HIF-P4H contributes to stabilization in a manner largely dependent on its cellular abundance Citation66. The siRNA data further suggest that the HIF-P4Hs may not act identically on the different HIF-α isoforms, with HIF-P4H-2 having more effect on HIF-1α and HIF-P4H-3 on HIF-2α Citation66. The expression of HIF-P4H-2 and -3 mRNAs, but not of HIF-P4H-1 mRNA, is induced by hypoxia Citation20, Citation58, Citation65–67, Citation69–74, which suggests that HIF-P4H-2 and -3 may have a role in a negative feedback pathway providing for the rapid degradation of HIF-α upon reoxygenation.
Two inherited heterozygous mutations, Pro317Arg and Arg371His, have recently been reported in HIF-P4H-2 in two families with erythrocytosis Citation75, Citation76. The Pro317Arg mutation is located two residues from the Fe2 + -binding aspartate in a C-terminal direction and has been found to substantially reduce the catalytic activity of the mutant HIF-P4H-2 and its binding to HIF-αs Citation75. The Arg371His is located close to the other mutation in the tertiary structure of the enzyme and suggests the presence of a HIF binding groove Citation76.
HIF-P4H-2 null mice die between days 12.5 and 14.5 of embryonic development due to severe placental and heart defects, while HIF-P4H-1 and HIF-P4H-3 null mice are viable Citation77. Studies on conditional HIF-P4H-2 knock-out mice have recently shown that lack of its activity leads to increased angiogenesis and angiectasia, highly perfusable blood vessels, severe erythrocytosis, polycythemia, and congestive heart failure Citation78–80. Lack of HIF-P4H-1 in mice induces hypoxia tolerance by reprogramming glucose metabolism from oxidative to more anaerobic energy production Citation81. A double knock-out of HIF-P4Hs 1 and 3 leads to moderate erythrocytosis, accumulation of HIF-2α in the liver, and activation of the hepatic Epo pathway, while lack of HIF-P4H-2 activity in adult mice causes accumulation of HIF-1α in the kidney and activation of the renal Epo pathway Citation79.
P4H-TM
P4H-TM was identified based on sequence similarity to the C-P4H α subunits and was found to be a homodimeric ER transmembrane protein with its catalytic site inside the lumen Citation39, Citation40. Its existence seems to be restricted to vertebrates, as it is not found in nematodes or flies Citation40. The P4H-TM polypeptide consists of 502 residues, the transmembrane domain spanning residues 59–82. The P4H-TM sequence is 14%–15% and 10%–13% identical to the C-P4H α subunits and HIF-P4Hs, respectively, the corresponding identities between the catalytic C-terminal regions being 26%–28% and 13%–15% Citation40. P4H-TM also resembles the C-P4H α subunits in that the basic residue that binds the C5 carboxyl group of 2-oxoglutarate is a lysine in position +10 with respect to the second Fe2 + -binding histidine (). The P4H-TM polypeptide does not show any sequence similarity to the peptide-substrate-binding domain of the C-P4H α subunits, however.
P4H-TM levels in cultured cells are increased by hypoxia, but its location is not affected Citation40. P4H-TM mRNA is expressed in many human tissues, with the highest levels in the brain, heart, skeletal muscle, kidney, pancreas, and placenta Citation39, Citation40.
Catalytic properties
Cosubstrates and reaction mechanism
The hydroxylation reaction catalyzed by the P4Hs requires Fe2 + , 2-oxoglutarate, O2, and ascorbate () Citation5–8, Citation23, Citation24. A P4H-Fe2 + complex is formed first, followed by binding of 2-oxoglutarate, the substrate, and O2 in this order Citation23, Citation24. The 2-oxoglutarate is stoichiometrically decarboxylated during hydroxylation, with one atom of the O2 molecule being incorporated into the succinate and the other into the hydroxy group formed on the proline residue (). Ascorbate is not consumed stoichiometrically, and P4Hs can catalyze a number of reaction cycles in its absence. P4Hs also catalyze uncoupled decarboxylation of 2-oxoglutarate, however, even in the presence of saturating peptide substrate concentrations, i.e. decarboxylation without subsequent hydroxylation of a proline residue, and ascorbate is consumed stoichiometrically and acts as an alternative oxygen acceptor in these cycles () Citation5–8, Citation23, Citation24.
Figure 4. Reactions catalyzed by P4Hs. 2-Oxoglutarate is stoichiometrically decarboxylated during the hydroxylation reaction, which does not require ascorbate (A). The enzymes also catalyze the uncoupled decarboxylation of 2-oxoglutarate without subsequent hydroxylation of the peptide substrate. Ascorbate is stoichiometrically consumed in the uncoupled reaction, which may occur in either the presence (B) or absence (C) of the peptide substrate.]
![Figure 4. Reactions catalyzed by P4Hs. 2-Oxoglutarate is stoichiometrically decarboxylated during the hydroxylation reaction, which does not require ascorbate (A). The enzymes also catalyze the uncoupled decarboxylation of 2-oxoglutarate without subsequent hydroxylation of the peptide substrate. Ascorbate is stoichiometrically consumed in the uncoupled reaction, which may occur in either the presence (B) or absence (C) of the peptide substrate.]](/cms/asset/c6874f54-68b4-494f-8547-4c2155c0e31e/iann_a_298825_f0004_b.gif)
Many 2-oxoglutarate dioxygenases have now been crystallized Citation82, including a truncated HIF-P4H-2 form Citation59 and a monomeric 253-residue P4H from the green alga Chlamydomonas reinhardtii that hydroxylates various proline-rich cell wall glycoproteins Citation83, Citation84. Although the overall sequence similarity between the various 2-oxoglutarate dioxygenases is very low, their catalytic sites are all located in a common core fold, a double-stranded β-helix (jelly-roll) fold that consists of eight β-strands Citation82. The catalytic site of the P4Hs consists of a set of separate locations for binding of the cosubstrates () Citation85. The Fe2 + binding site is coordinated by the conserved -His-X-Asp-…-His- motif Citation19–21, Citation50, Citation59, Citation84. The 2-oxoglutarate binding site can be divided into at least two distinct subsites: 1) a positively charged residue located at position +10 (C-P4Hs) or +9 (HIF-P4Hs) with respect to the distal Fe2 + -binding histidine, together with some other residues Citation19–21, Citation50, Citation59, Citation84 that bind the C5 carboxyl group; and 2) two co-ordination sites of the enzyme-bound Fe2 + that bind the C1-C2 moiety () Citation59, Citation84, Citation85.
Figure 5. Schematic representation of the catalytic site of the α(I) subunit of human C-P4H-I. Fe2 + is coordinated with the enzyme by His412, Asp414, and His483. Subsite I of the 2-oxoglutarate binding site consists of Lys493, which ionically binds the C5 carboxyl group of 2-oxoglutarate, while subsite II consists of two cis-positioned equatorial coordination sites of the enzyme-bound Fe2 + and is chelated by the C1 carboxyl and C2 oxo functions of the 2-oxoglutarate. Molecular oxygen is bound end-on in an axial position, producing a dioxygen unit.
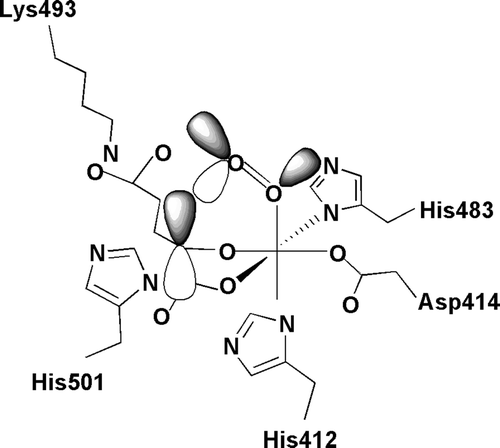
The specific activity of a purified recombinant human C-P4H-I has been reported to be 400 mol/mol/min, while values of 20 mol/mol/min and 40–50 mol/mol/min have been determined for endogenous HIF-P4H-2 in crude cell lysates and recombinant human HIF-P4Hs 1-3 purified from insect cells, respectively Citation86, Citation87. The Km values of recombinant human C-P4Hs I–III and HIF-P4Hs 1–3 for Fe2 + , 2-oxoglutarate, and ascorbate are summarized in Citation8, Citation9, Citation50, Citation54, Citation61, Citation87–90. The Km values of all three C-P4Hs are essentially identical, except that the Km of C-P4H-III for Fe2 + is one-fourth of those of C-P4Hs I and II (). The Km values of HIF-P4H 1 and 2 are very similar, while the values of HIF-P4H-3 for Fe2 + and 2-oxoglutarate are 3–10-fold higher than those of the other two () Citation87, Citation89, Citation91. In general, the Km values of the HIF-P4Hs for Fe2 + and 2-oxoglutarate are lower than those of the C-P4Hs, especially in the case of HIF-P4H-1 and -2 (). The Km values of HIF-P4Hs for ascorbate are also about a half of those of the C-P4Hs (), whereas their Km values for O2 are higher (). The Km values of HIF-P4Hs for O2, when measured with a 19-residue peptide substrate representing the C-terminal hydroxylation site in HIF-1α, are very high, 230–250 µM, i.e. slightly above the concentration of dissolved O2 in the air Citation61, but these Km values depend on the length of the substrate used Citation88, Citation90, being about 65–100 µM in the presence of longer substrates representing the full-length HIF-1 ODDDs or their N- or C-terminal halves (). As even these values are above the O2 concentrations measured in almost all tissues under normoxic conditions in vivo Citation92, the in vivo activities of the HIF-P4Hs are likely to be limited by O2 and to be altered by changes in its concentrations in a very sensitive way, making the HIF-P4Hs effective oxygen sensors Citation88.
Table I. Km values of recombinant human C-P4Hs I-III and HIF-P4Hs 1-3 for Fe2 + , 2-oxoglutarate, O2 and ascorbatea.
Substrates and interacting proteins
The C-P4Hs act on proline residues only in peptide linkages, the minimum requirement being fulfilled by an X-Pro-Gly tripeptide, which agrees with the presence of 4-hydroxyproline in collagens almost exclusively in positions preceding a glycine Citation5, Citation6, Citation8, although the glycine can be replaced by an alanine or a glutamate in some rare cases. The hydroxylation efficiency is also affected by the amino acid preceding the proline and by other nearby amino acids, and the peptide chain length has a major effect in that the Km decreases with an increasing number of -X-Pro-Gly- repeats Citation5, Citation6, Citation8, Citation93. The major determinant for this phenomenon has been shown to be the higher binding affinity of the peptide-substrate-binding domain for long peptides Citation52. Hydroxylation of -X-Pro-Gly- triplets in collagen polypeptides must occur before the assembly of the collagen triple helix, as the triple-helical peptide conformation completely prevents hydroxylation.
The three C-P4H isoenzymes have certain differences in their peptide-binding properties. The Km values of C-P4Hs I and III for the peptide (Pro-Pro-Gly)10 are essentially identical, while that of C-P4H-II is about 5-fold higher Citation5–9. Poly(L-proline) inhibits C-P4H-I very effectively in a competitive manner with respect to the peptide substrate, whereas it inhibits C-P4H-II only at very high concentrations, C-P4H-III apparently being inhibited with intermediate efficiency Citation5–9. The peptide-substrate-binding domain has been shown to be the principal region responsible for these differences Citation52, and according to site-directed mutagenesis studies most of the differences in peptide binding between C-P4Hs I and II are caused by the presence of a glutamate and glutamine in the α(II) subunit positions corresponding to Ile182 and Tyr233 in α(I) Citation51.
4-Hydroxyproline is also found in more than 20 other proteins that have triple-helical collagen-like domains but are not classified as collagens, including the subcomponent C1q of complement, a C1q-like factor, adiponectin, several humoral lectins of the innate immune system, the tail structure of acetylcholinesterase, three macrophage receptors, ectodysplasin, gliomedin, elastic fiber-associated glycoproteins, and a src-homologous-and-collagen protein Citation1. Elastin, which does not have a triple-helical collagen-like domain, also has 4-hydroxyproline in the Y positions of its repeating -X-Y-Gly- sequences Citation6.
The HIF-P4Hs also hydroxylate only peptidyl prolines, but their sequence specificity is distinct from that of the C-P4Hs. The hydroxylated prolines in HIF-αs are present in -Leu-X-X-Leu-Ala-Pro- sequences, and it was therefore first suggested that the HIF-P4Hs may require this conserved core motif Citation16, Citation17. It was subsequently shown, however, that the two leucines can be replaced by many other residues, alanine being the only relatively, but still not absolutely, strict requirement in addition to the proline itself, the substrate specificity probably being achieved by multiple interactions, none of which is absolutely critical Citation94, Citation95. The N-terminal prolines in the HIF-1α and HIF-2α ODDDs are hydroxylated much less effectively than the C-terminal ones, especially in the case of HIF-P4H-3 Citation61, Citation88, Citation90, Citation96, Citation97. The HIF-P4Hs require longer substrates than the C-P4Hs, the shortest HIF-α-like peptide hydroxylated by all three HIF-P4Hs having 11 residues Citation61, and as in the case of the C-P4Hs, an increase in peptide length increases the hydroxylation efficiency by reducing the Km Citation61, Citation88, Citation90. No specific conformation is required, as a 19-residue synthetic HIF-1α peptide which is efficiently hydroxylated by all three HIF-P4Hs has no structured conformation in solution Citation95, Citation98.
The three HIF-P4Hs exhibit certain substrate preferences. RNA interference studies in cultured cells have suggested that HIF-P4H-2 may act more effectively on HIF-1α than on HIF-2α, while HIF-P4H-3 seems to have the opposite preference Citation66. Differences in the Km values of the three HIF-P4Hs for HIF-α-like synthetic peptides and HIF-1α and HIF-2α ODDDs Citation61, Citation88 do not explain these observations, however. It is therefore highly likely that the relative contributions of the three HIF-P4Hs to the regulation of HIF-1α and HIF-2α are determined by many factors, including differences in the Km values, abundance and cellular location of each isoenzyme, and the physiological status of the cell.
Recent data indicate that the HIF-P4Hs are also likely to act on proteins other than the HIF-αs. Indirect evidence suggests that the large subunit of RNA polymerase II, iron regulatory protein IRP-2, IκB kinase-β, and the activating transcription factor ATF4 are regulated by HIF-P4Hs in an oxygen-dependent manner and may therefore be novel substrates Citation99–103. Non-substrate proteins interacting with HIF-P4Hs may also affect the hypoxia response by regulating the amount or activity of HIF-P4Hs or the recruitment of additional proteins, and may provide links with other signaling pathways. The cytosolic chaperonin TriC, for example, associates with HIF-P4H-3 and may regulate its activity Citation104, while the ring finger protein Siah2 negatively regulates HIF-P4H-1 and -3 levels by targeting them to proteasomal degradation Citation105, Citation106, OS-9 interacts with both HIF-P4Hs and HIF-1α and promotes hydroxylation and degradation of HIF-1α Citation107, the candidate tumor suppressor ING4 associates with HIF-P4Hs and is recruited by this means to HIF to act as an adapter for the binding of transcriptional repressors of HIF activity Citation108, the mitogen-activated protein kinase organizer Morg1 acts as a molecular scaffold for HIF-P4H-3 Citation109, and the peptidyl prolyl cis/trans isomerase FKBP38 interacts with HIF-P4H-2 and prolongs its stability Citation110.
Despite the cellular location of P4H-TM and its higher sequence identity with the C-P4H α subunits than the HIF-P4Hs, P4H-TM was found to hydroxylate HIF-1α but not type I procollagen chains in vitro Citation39, Citation40. Furthermore, overexpression of P4H-TM suppressed HIF activity and reduced cellular recombinant HIF-α ODDD levels, whereas silencing of P4H-TM increased the HIF-1α protein level Citation39, Citation40. These data indicate that P4H-TM can also act on HIF-α in cellulo. P4H-TM resembles HIF-P4H-3 in that it acts very inefficiently on the N-terminal hydroxylation site of HIF-1α Citation40. A low level of hydroxylation was nevertheless obtained with P4H-TM even with a mutant ODDD in which both known target prolines of the HIF-P4Hs were changed to alanine Citation40. This indicates that the substrate specificity of P4H-TM differs from those of the HIF-P4Hs, and it is thus possible that P4H-TM may have additional, as yet unknown, physiological substrates.
Inhibitors
The central roles of the C-P4Hs and HIF-P4Hs in synthesis of the extracellular matrix and regulation of the hypoxia response, respectively, have prompted attempts to develop inhibitors of their activity for therapeutic purposes (see below). Numerous compounds are known to inhibit the C-P4Hs competitively with respect to some of the cosubstrates or the peptide substrate (for reviews, see Citation5, Citation6, Citation8). As C-P4H inhibitors have been extensively discussed in the previous literature, this chapter will mainly concentrate on recent advances that have been made in this field since the discovery of the HIF-P4Hs Citation111, Citation112. All P4Hs are inhibited by iron chelators such as α,α’-dipyridyl, desferrioxamine, and ciclopirox olamine, the HIF-P4Hs being inhibited much less effectively than C-P4Hs, however, presumably due to their markedly tighter binding of Fe2 + Citation19, Citation20, Citation87, Citation113. Many bivalent cations such as Zn2 + , Co2 + , Cd2 + , and Ni2 + inhibit P4Hs competitively with respect to Fe2 + () Citation19, Citation20, Citation87, and Co2 + and Ni2 + are known hypoxia mimetics and stabilize HIF-1α in cultured cells Citation28, Citation87. As competitive inhibition of HIF-P4Hs, especially of HIF-P4H-2, by these metals was not particularly effective, it is possible that other reported mechanisms, i.e. depletion of intracellular ascorbate or direct binding to HIF-1α, inhibiting its proteasomal degradation, may also contribute to the metal-induced stabilization of HIF-1α Citation87, Citation114, Citation115.
Table II. Inhibition of purified recombinant C-P4H-I and HIF-P4Hs 1-3 by certain metals and 2-oxoglutarate analoguesa.
Induction of nitric oxide formation in normoxic cell cultures leads to the inhibition of collagen synthesis and stabilization of HIF-1α Citation116, Citation117. Nitric oxide competes with the binding of oxygen to 2-oxoglutarate dioxygenases Citation23 and inhibits HIF-P4Hs under normoxic conditions Citation117, Citation118. Reactive oxygen species also reduce HIF-P4H activity and lead to subsequent accumulation of HIF, but the exact mechanism remains undetermined Citation119–121.
A number of structural analogues of 2-oxoglutarate inhibit P4Hs competitively with respect to this cosubstrate Citation5, Citation6, Citation8, Citation21, Citation61, Citation111, Citation112, Citation122–128 (). Distinct differences are found in the inhibitory potency of such compounds with respect to the C-P4Hs and HIF-P4Hs Citation61 (), indicating that their catalytic sites differ sufficiently to allow the development of specific inhibitors of the two P4H classes, which would in turn enable the development of selective therapeutics. Pyridine 2,5-dicarboxylate, for example, is a very potent inhibitor of the C-P4Hs but does not inhibit the HIF-P4Hs Citation61 (). It should also be noted that certain differences are to be found in the inhibition of the individual HIF-P4H isoenzymes Citation61 ().
2-Oxoglutarate is a metabolic intermediate generated by the mitochondrial tricarboxylic acid cycle (TCA), and certain TCA intermediates such as fumarate and succinate have been shown to inhibit the HIF-P4Hs and C-P4Hs () Citation88, Citation129–132. Mutations in the genes for the TCA cycle enzymes fumarate hydratase and succinate dehydrogenase predispose subjects to dominantly inherited highly vascular tumors with accumulated HIF-1α and elevated fumarate and succinate levels Citation133, respectively, and the inhibition of HIF-P4Hs is thus likely to play an important role in the pathology of these tumors.
Therapeutic possibilities
Normal wound repair after injury involves the formation of scars and fibrous tissue, but in some situations collagen accumulates in excessive amounts, leading to pathological fibrosis, which compromises the normal architecture and functioning of the affected tissue. Fibrosis can occur in essentially any organ or tissue, but most commonly affects the liver, kidneys, lungs, and skin. The central role of collagen in fibrosis has prompted attempts to develop drugs that inhibit its accumulation, and the critical function of 4-hydroxyproline in collagens has made C-P4Hs an attractive target for antifibrotic therapy (for reviews, see Citation5, Citation55, Citation134). Many compounds that inhibit C-P4Hs are now known (see above), and some of them have shown promising results in various models of fibrosis Citation5, Citation6, Citation8, but no such inhibitor is yet in clinical use. Candidate inhibitors should be highly selective, as the treatment of chronic fibrotic conditions will most probably require long-term C-P4H inhibition, and this should not interfere with the functioning of the HIF-P4Hs.
The activation of HIF may prove to be of great therapeutic value in a number of pathological ischemic and hypoxic conditions Citation27, Citation33, Citation34, Citation111, Citation112, Citation135, Citation136. The central role of the HIF-P4Hs in the regulation of the stability of HIF-1α and HIF-2α has provided an attractive possibility for the development of therapies. The identification and design of small-molecule HIF-P4H inhibitors that lead to the stabilization of HIF has therefore attracted considerable attention. Evidence of the effectiveness of such inhibitors is now rapidly accumulating from preclinical and clinical studies.
The biological process known as preconditioning, i.e. cytoprotective adaptation triggered by brief periods of sublethal ischemia, is known to enhance cellular mechanisms that provide protection against postischemic injury Citation135, Citation137, Citation138. HIF target genes are important mediators of this phenomenon, as neuron-specific inactivation of HIF-1α, for instance, has been shown to increase brain injury in a mouse model of transient focal cerebral ischemia Citation139, and a partial deficiency in HIF-1α leads to a complete loss of the cardioprotection induced by hypoxic preconditioning in mice Citation140. The P4H inhibitors ethyl 3,4-dihydroxybenzoate and dimethyloxalylglycine protect the cardiomyocytes from stress caused by metabolic inhibition and attenuate postischemic myocardial injury in rabbits Citation141, Citation142, and L-mimosine and dimethyloxalylglycine protect mouse kidneys from renal ischemia reperfusion injury Citation143. Various proprietory HIF-P4H inhibitors developed at FibroGen Inc. have been shown to inhibit the death of embryonic rat cortical neurons caused by oxidative stress and to mediate a preconditioning that results in reduction of the neuronal damage induced by permanent focal brain ischemia in adult rats in vivo Citation144, to improve cardiac function after acute myocardial infarction in rats and rabbits Citation141, Citation145, to alleviate acute ischemic renal failure in rats Citation146, and to provide neuroprotection and renoprotection in rodent permanent focal brain ischemia and acute kidney injury models, respectively Citation147, Citation148. Remarkably, the protective efficacy in the two last-mentioned reports was not limited to preconditioning, but was even observed upon administration of the HIF-P4H inhibitor after the initiation of the ischemic insult. Other unique HIF-P4H inhibitors have also been shown to exert neuron protection in a rodent model of cerebrovascular disease Citation128.
Recombinant human Epo is used to treat severe anemias associated with renal disease and cancer, for example Citation149, Citation150. An interesting alternative strategy is provided by the induction of HIF through HIF-P4H inhibitors, which would not only enhance the expression of Epo but also upregulate other genes that are important for erythropoiesis Citation149–151. Such compounds have been shown to effectively correct anemia and improve iron utilization in rodent models of anemia Citation152 and to significantly increase erythropoiesis and prevent anemia induced by weekly phlebotomy in rhesus macaques Citation153. The increased erythropoiesis induced by these HIF-P4H inhibitors was found to occur in xenograft tumor models without the promotion of tumor progression in the presence or absence of concomitant chemotherapy Citation152. Furthermore, induction of effective erythropoiesis and increased hemoglobin concentrations has been obtained in anemic human patients with chronic kidney disease by means of the HIF-P4H inhibitors FG-2216 and FG-4592 in two phase 2 studies Citation154, Citation155, and the stimulation of Epo production has even been seen in anephric hemodialysis patients in a phase 1 study Citation156.
HIF activates the expression of several angiogenic growth factors, such as the vascular endothelial growth factor (VEGF), and their receptors Citation157. Single angiogenic growth factors are insufficient for the formation of a functional vasculature, as the newly formed vessels induced by VEGF, for example, are leaky and poorly connected to the existing vasculature Citation158. Activation of the angiogenic program by HIF may therefore offer major advantages in therapeutic angiogenesis. In agreement with this suggestion, gene delivery of a stabilized form of HIF-1α by an adeno-associated virus was shown to be superior to VEGF for angiogenesis in mouse skeletal muscle Citation159. Stabilization of HIF by HIF-P4H inhibition induced angiogenesis in rodent sponge models Citation128, Citation160, and angiogenic responses triggered by HIF-P4H inhibition improved lung growth and function in chronic lung disease of prematurity in primates Citation161, Citation162.
The data obtained with P4H inhibitors in various animal models of fibrosis, anemia, and ischemia, and in ongoing clinical trials with HIF-P4H inhibitors, suggest efficacy in a number of applications (see above). The development of P4H inhibitors for therapeutic purposes also presents several challenges, however. Although it seems possible to develop specific inhibitors for the two classes of P4Hs, their specificity against the large number of other 2-oxoglutarate dioxygenases, and thus the lack of unwanted off-target effects, remains to be established. Likewise, the variety of responses triggered by stabilization of HIF presents both challenges and opportunities. It is significant, however, that certain HIF-P4H inhibitors have been shown to display specificity with respect to the HIF target genes upregulated, e.g. by specifically mediating erythropoietic but not angiogenic responses Citation151.
References
- Myllyharju J, Kivirikko KI. Collagens, modifying enzymes and their mutations in humans, flies and worms. Trends Genet. 2004; 20: 33–43
- Ricard-Blum S, Ruggiero F. The collagen superfamily: from the extracellular matrix to the cell membrane. Pathol Biol. 2005; 53: 430–42
- Veit G, Kobbe B, Keene DR, Paulsson M, Koch M, Wagener R. Collagen XXVIII, a novel von Willebrand factor A domain-containing protein with many imperfections in the collagenous domain. J Biol Chem. 2006; 281: 3494–504
- Söderhäll C, Marenholz I, Kerscher T, Rüschendorf S, Esparza-Gordillo J, Worm M, et al. Variants in a novel epidermal collagen gene (COL29A1) are associated with atopic dermatitis. PLoS Biol. 2007; 5: e242
- Kivirikko KI, Myllyharju J. Prolyl 4-hydroxylases and their protein disulfide isomerase subunit. Matrix Biol. 1998; 16: 357–68
- Kivirikko KI, Pihlajaniemi T. Collagen hydroxylases and the protein disulfide isomerase subunit of prolyl 4-hydroxylases. Adv Enzymol Relat Areas Mol Biol. 1998; 72: 325–98
- Myllyharju J. Prolyl 4-hydroxylases, the key enzymes of collagen biosynthesis. Matrix Biol. 2003; 22: 15–24
- Myllyharju J. Intracellular post-translational modifications of collagens. Top Curr Chem. 2005; 247: 115–47
- Kukkola L, Hieta R, Kivirikko KI, Myllyharju J. Identification and characterization of a third human, rat, and mouse collagen prolyl 4-hydroxylase isoenzyme. J Biol Chem. 2003; 278: 47685–93
- Van Den Diepstraten C, Papay K, Bolender Z, Brown A, Pickering JG. Cloning of a novel prolyl 4-hydroxylase subunit expressed in the fibrous cap of human atherosclerotic plaque. Circulation. 2003; 108: 508–11
- Annunen P, Koivunen P, Kivirikko KI. Cloning of the α subunit of prolyl 4-hydroxylase from Drosophila and expression and characterization of the corresponding enzyme tetramer with some unique properties. J Biol Chem. 1999; 274: 6790–6
- Keskiaho K, Kukkola L, Page AP, Winter AD, Vuoristo J, Sormunen R, , et al Characterization of a novel Caenorhabditis elegans prolyl 4-hydroxylase with a unique substrate specificity and restricted expression in the pharynx and excretory duct. J Biol Chem. 2008, in press.
- Myllyharju J, Kukkola L, Winter AD, Page AP. The exoskeleton collagens in Caenorhabditis elegans are modified by prolyl 4-hydroxylases with unique combinations of subunits. J Biol Chem. 2002; 277: 29187–96
- Riihimaa P, Nissi R, Page AP, Winter AD, Keskiaho K, Kivirikko KI, et al. Egg shell collagen formation in Caenorhabditis elegans involves a novel prolyl 4-hydroxylase expressed in spermatheca and embryos and possessing many unique properties. J Biol Chem. 2002; 277: 18238–43
- Abrams EW, Mihoulides WK, Andrew DJ. Fork head and Sage maintain a uniform and patent salivary gland lumen through regulation of two downstream target genes, PH4alphaSG1 and PH4alphaSG2. Development. 2006; 133: 3517–27
- Ivan M, Kondo K, Yang H, Kim W, Valiando J, Ohh M, et al. HIFα targeted for VHL-mediated destruction by proline hydroxylation: implications for O2 sensing. Science. 2001; 292: 464–8
- Jaakkola P, Mole DR, Tian Y-M, Wilson MI, Gielbert J, Gaskell SJ, et al. Targeting of HIF-α to the von Hippel-Lindau ubiquitylation complex by O2-regulated prolyl hydroxylation. Science. 2001; 292: 468–72
- Yu F, White SB, Zhao Q, Lee FS. HIF-1α is regulated by stimulus sensitive proline hydroxylation. Proc Natl Acad Sci USA. 2001; 98: 9630–5
- Bruick RK, McKnight SL. A conserved family of prolyl 4-hydroxylases that modify HIF. Science. 2001; 294: 1337–40
- Epstein ACR, Gleadle JM, McNeill LA, Hewitson KS, O'Rourke J, Mole DR, et al. C. elegans EGL-9 and mammalian homologs define a family of dioxygenases that regulate HIF by prolyl hydroxylation. Cell. 2001; 107: 43–54
- Ivan M, Haberberger T, Gervasi DC, Michelson KS, Günzler V, Kondo K, et al. Biochemical purification and pharmacological inhibition of a mammalian prolyl hydroxylase acting on hypoxia-inducible factor. Proc Natl Acad Sci USA. 2002; 99: 13459–64
- Centanin L, Ratcliffe PJ, Wappner P. Reversion of lethality and growth defects in Fatiga oxygen-sensor mutant flies by loss of hypoxia-inducible factor-α/Sima. EMBO Rep. 2005; 6: 1070–5
- Schofield CJ, Ratcliffe PJ. Oxygen sensing by HIF hydroxylases. Nat Rev Mol Cell Biol. 2004; 5: 343–54
- Schofield CJ, Ratcliffe PJ. Signalling hypoxia by HIF hydroxylases. Biochem Biophys Res Commun. 2005; 338: 617–26
- Kaelin WG, Jr. Proline hydroxylation and gene expression. Annu Rev Biochem. 2005; 74: 115–28
- Ozer A, Bruick RK. Non-heme dioxygenases: cellular sensors and regulators jelly rolled into one?. Nat Chem Biol. 2007; 3: 144–53
- Siddiq A, Aminova LR, Ratan RR. Hypoxia inducible factor prolyl 4-hydroxylase enzymes: center stage in the battle against hypoxia, metabolic compromise and oxidative stress. Neurochem Res. 2007; 32: 931–46
- Semenza GL. HIF-1, O2, and the 3 PHDs: how animal cells signal hypoxia to the nucleus. Cell. 2001; 107: 1–3
- Hofbauer K-H, Gess B, Lohaus C, Meyer HE, Katschinski D, Kurtz A. Oxygen tension regulates the expression of a group of procollagen hydroxylases. Eur J Biochem. 2003; 270: 4515–22
- Wang V, Davis DA, Haque M, Huang LE, Yarchoan R. Differential gene up-regulation by hypoxia-inducible factor-1α and hypoxia-inducible factor-2α in HEK293T cells. Cancer Res. 2005; 65: 3299–306
- Elvidge GP, Glenny L, Appelhoff RJ, Ratcliffe PJ, Ragoussis J, Gleadle JM. Concordant regulation of gene expression by hypoxia and 2-oxoglutarate-dependent dioxygenase inhibition. The role of HIF-1α, HIF-2α, and other pathways. J Biol Chem. 2006; 281: 15215–26
- Erler JT, Bennewith KL, Nicolau M, Dornhöfer N, Kong C, Le Q-T, et al. Lysyl oxidase is essential for hypoxia-induced metastasis. Nature. 2006; 440: 1222–6
- Ratcliffe PJ. Understanding hypoxia signalling in cells—a new therapeutic opportunity?. Clin Med. 2006; 6: 573–8
- Ratcliffe PJ. HIF-1 and HIF-2: working alone or together in hypoxia?. J Clin Invest. 2007; 117: 862–5
- Hu C-J, Wang L-Y, Chodosh LA, Keith B, Simon MC. Differential roles of hypoxia-inducible factor 1α (HIF-1α) and HIF-2α in hypoxic gene regulation. Mol Cell Biol. 2003; 23: 9361–74
- Warnecke C, Zaborowska Z, Kurreck J, Erdmann VA, Wiesener M, Eckardt KU. Differentiating the functional role of hypoxia-inducible factor (HIF)-1α and HIF-2α (EPAS-1) by the use of RNA interference: erythropoietin is a HIF-2α target gene in Hep3B and Kelly cells. FASEB J. 2004; 18: 1462–4
- Gruber M, Hu C-J, Johnson RS, Brown EJ, Keith B, Simon MC. Acute postnatal ablation of HIF-2α results in anemia. Proc Natl Acad Sci U S A. 2007; 104: 2301–6
- Rankin EB, Biju MP, Liu Q, Unger TL, Rha J, Johnson RS, et al. Hypoxia-inducible factor-2 (HIF-2) regulates hepatic erythropoietin in vivo. J Clin Invest. 2007; 117: 1068–77
- Oehme F, Ellinghaus P, Kolkhof P, Smith TJ, Ramakrishnan S, Hütter J, et al. Overexpression of PH-4, a novel putative proline 4-hydroxylase, modulates activity of hypoxia-inducible transcription factors. Biochem Biophys Res Commun. 2002; 296: 343–9
- Koivunen P, Tiainen P, Hyvärinen J, Williams KE, Sormunen R, Klaus SJ, et al. An endoplasmic reticulum transmembrane prolyl 4-hydroxylase is induced by hypoxia and acts on hypoxia-inducible factor α. J Biol Chem. 2007; 282: 30544–52
- Vuorela A, Myllyharju J, Nissi R, Pihlajaniemi T, Kivirikko KI. Assembly of human prolyl 4-hydroxylase and type III collagen in the yeast Pichia pastoris: formation of a stable enzyme tetramer requires coexpression with collagen and assembly of a stable collagen requires coexpression with prolyl 4-hydroxylase. EMBO J. 1997; 16: 6702–12
- Merle C, Perret S, Lacour T, Jonval V, Hudaverdian S, Garrone R, et al. Hydroxylated human homotrimeric collagen I in Agrobacterium tumefaciens-mediated transient expression and in transgenic tobacco plant. FEBS Lett. 2002; 515: 114–8
- Kersteen EA, Higgin JJ, Raines RT. Production of human prolyl 4-hydroxylase in Escherichia coli. Protein Expr Purif. 2004; 38: 279–91
- Neubauer A, Neubauer P, Myllyharju J. High-level production of human collagen prolyl 4-hydroxylase in Escherichia coli. Matrix Biol. 2005; 24: 59–68
- Lamberg A, Helaakoski T, Myllyharju J, Peltonen S, Notbohm H, Pihlajaniemi T, et al. Characterization of human type III collagen expressed in a baculovirus system. Production of a protein with a stable triple helix requires coexpression with the two types of recombinant prolyl 4-hydroxylase subunit. J Biol Chem. 1996; 271: 11988–95
- Myllyharju J, Lamberg A, Notbohm H, Fietzek PP, Pihlajaniemi T, Kivirikko KI. Expression of wild-type and modified proα chains of human type I procollagen in insect cells leads to the formation of stable [α1(I)]2α2(I) collagen heterotrimers and [α1(I)]3 homotrimers but not [α2(I)]3 homotrimers. J Biol Chem. 1997; 272: 21824–30
- Toman PD, Chisholm G, McMullin H, Giere LM, Olsen DR, Kovach RJ, et al. Production of recombinant human type I procollagen trimers using a four-gene expression system in the yeast Saccharomyces cerevisiae. J Biol Chem. 2000; 275: 23303–9
- Nokelainen M, Tu H, Vuorela A, Notbohm H, Kivirikko KI, Myllyharju J. High-level production of recombinant human type I collagen in the yeast Pichia pastoris. Yeast. 2001; 18: 797–806
- Pakkanen O, Hämäläinen E-R, Kivirikko KI, Myllyharju J. Assembly of stable human type I and III collagen molecules from hydroxylated recombinant chains in the yeast Pichia pastoris. Effect of an engineered C-terminal oligomerization domain foldon. J Biol Chem. 2003; 278: 32478–83
- Myllyharju J, Kivirikko KI. Characterization of the iron- and 2-oxoglutarate-binding sites of human prolyl 4-hydroxylase. EMBO J. 1997; 16: 1173–80
- Myllyharju J, Kivirikko KI. Identification of a novel proline-rich peptide-binding domain in prolyl 4-hydroxylase. EMBO J. 1999; 18: 306–12
- Hieta R, Kukkola L, Permi P, Pirilä P, Kivirikko KI, Kilpeläinen I, et al. The peptide-substrate-binding domain of human collagen prolyl 4-hydroxylases. Backbone assignments, secondary structure, and binding of proline-rich peptides. J Biol Chem. 2003; 278: 34966–74
- Pekkala M, Hieta R, Bergmann U, Kivirikko KI, Wierenga RK, Myllyharju J. The peptide-substrate-binding domain of collagen prolyl 4-hydroxylases is a tetratricopeptide repeat domain with functional aromatic residues. J Biol Chem. 2004; 279: 52255–61
- Nokelainen M, Nissi R, Kukkola L, Helaakoski T, Myllyharju J. Characterization of the human and mouse genes for the α subunit of type II prolyl 4-hydroxylase. Identification of a previously unknown alternative spliced exon and its expression in various tissues. Eur J Biochem. 2001; 268: 5300–9
- Myllyharju J, Kivirikko KI. Collagens and collagen-related diseases. Ann Med. 2001; 33: 7–21
- Grimmer C, Balbus N, Lang U, Aigner T, Cramer T, Müller L, et al. Regulation of type II collagen synthesis during osteoarthritis by prolyl-4-hydroxylases. Possible influence of low oxygen levels. Am J Pathol. 2006; 169: 491–502
- Holster T, Pakkanen O, Soininen R, Sormunen R, Nokelainen M, Kivirikko KI, et al. Loss of assembly of the main basement membrane collagen, type IV, but not fibril-forming collagens and embryonic death in collagen prolyl 4-hydroxylase I null mice. J Biol Chem. 2007; 282: 2512–9
- Metzen E, Berchner-Pfannschmidt U, Stengel P, Marxsen J, Stolze I, Klinger M, et al. Intracellular localisation of human HIF-1α hydroxylases: implications for oxygen sensing. J Cell Sci. 2003; 116: 1319–26
- McDonough MA, Li V, Flashman E, Chowdury R, Mohr C, Liénard BMR, et al. Cellular oxygen sensing: crystal structure of hypoxia-inducible factor prolyl hydroxylase (PHD2). Proc Natl Acad Sci USA. 2006; 103: 9814–9
- Choi K-O, Lee T, Lee N, Kim J-H, Yang EG, Yoon JM, et al. Inhibition of the catalytic activity of hypoxia-inducible factor-1α-prolyl-hydroxylase 2 by a MYND-type zinc finger. Mol Pharmacol. 2005; 68: 1803–9
- Hirsilä M, Koivunen P, Günzler V, Kivirikko KI, Myllyharju J. Characterization of the human prolyl 4-hydroxylases that modify the hypoxia-inducible factor. J Biol Chem. 2003; 278: 30772–80
- Cervera AM, Apostolova N, Luna-Crespo F, Sanjuan-Pla A, Garcia-Pou R, McCreath KJ. An alternatively spliced transcript of the PHD3 gene retains prolyl hydroxylase activity. Cancer Lett. 2006; 233: 131–8
- Tian Y-M, Mole DR, Ratcliffe PJ, Gleadle JM. Characterization of different isoforms of the HIF prolyl hydroxylase PHD1 generated by alternative initiation. Biochem J. 2006; 397: 179–86
- Lieb ME, Menzies K, Moschella MC, Ni R, Taubman MB. Mammalian EGLN genes have distinct patterns of mRNA expression and regulation. Biochem Cell Biol. 2002; 80: 421–6
- Cioffi CL, Liu XQ, Kosinski PA, Garay M, Bowen BR. Differential regulation of HIF-1α prolyl-4-hydroxylase genes by hypoxia in human cardiovascular cells. Biochem Biophys Res Commun. 2003; 303: 947–53
- Appelhoff RJ, Tian Y-M, Raval RR, Turley H, Harris AL, Pugh CW, et al. Differential function of the prolyl hydroxylases PHD1, PHD2, and PHD3 in the regulation of hypoxia-inducible factor. J Biol Chem. 2004; 279: 38458–65
- Willam C, Maxwell PH, Nichols L, Lygate C, Tian YM, Bernhardt W, et al. HIF prolyl hydroxylases in the rat; organ distribution and changes in expression following hypoxia and coronary artery ligation. J Mol Cell Cardiol. 2006; 41: 68–77
- Li N, Yi F, Sundy CM, Chen L, Hilliker ML, Donley DK, et al. Expression and actions of HIF prolyl-4-hydroxylase in the rat kidneys. Am J Physiol Renal Physiol. 2007; 292: F207–16
- Berra E, Benizri E, Ginouvès A, Volmat V, Roux D, Pouysségur J. HIF prolyl-hydroxylase 2 is the key oxygen sensor setting low steady-state levels of HIF-1α in normoxia. EMBO J. 2003; 22: 4082–90
- D'Angelo G, Duplan E, Boyer N, Vigne P, Frelin C. Hypoxia up-regulates prolyl hydroxylase activity. A feedback mechanism that limits HIF-1 responses during reoxygenation. J Biol Chem. 2003; 278: 38183–7
- del Peso L, Castellanos MC, Temes E, Martín-Puig S, Cuevas Y, Olmos G, et al. The von Hippel Lindau/hypoxia-inducible factor (HIF) pathway regulates the transcription of the HIF-proline hydroxylase genes in response to low oxygen. J Biol Chem. 2003; 278: 48690–5
- Marxsen JH, Stengel P, Doege K, Heikkinen P, Jokilehto T, Wagner T, et al. Hypoxia-inducible factor-1 (HIF-1) promotes its degradation by induction of HIF-α-prolyl-4-hydroxylases. Biochem J. 2004; 381: 761–7
- To KKW, Huang LE. Suppression of hypoxia-inducible factor 1α (HIF-1α) transcriptional activity by the HIF prolyl hydroxylase EGLN1. J Biol Chem. 2005; 280: 38102–7
- Stiehl DP, Wirthner R, Köditz J, Spielmann P, Camenisch G, Wenger RH. Increased prolyl 4-hydroxylase domain proteins compensate for decreased oxygen levels. Evidence for an autoregulatory oxygen-sensing system. J Biol Chem. 2006; 281: 23482–91
- Percy MJ, Zhao Q, Flores A, Harrison C, Lappin TRJ, Maxwell PH, et al. A family with erythrocytosis establishes a role for prolyl hydroxylase domain protein 2 in oxygen homeostasis. Proc Natl Acad Sci USA. 2006; 103: 654–9
- Percy MJ, Furlow PW, Beer PA, Lappin TR, McMullin MF, Lee FS. A novel erythrocytosis-associated PHD2 mutation suggests the location of a HIF binding groove. Blood. 2007; 110: 2193–6
- Takeda K, Ho VC, Takeda H, Duan L-J, Nagy A, Fong G-H. Placental but not heart defects are associated with elevated hypoxia-inducible factor α levels in mice lacking prolyl hydroxylase domain protein 2. Mol Cell Biol. 2006; 26: 8336–46
- Takeda K, Cowan A, Fong G-H. Essential role for prolyl hydroxylase domain protein 2 in oxygen homeostasis of the adult vascular system. Circulation. 2007; 116: 774–81
- Takeda K, Aguila HL, Parikh NS, Li X, Lamothe K, Duan LJ, et al. Regulation of adult erythropoiesis by prolyl hydroxylase domain proteins. Blood. 2008; 111: 3229–35
- Minamishima YA, Moslehi J, Bardeesy N, Cullen D, Bronson RT, Kaelin WG, Jr. Somatic inactivation of the PHD2 prolyl hydroxylase causes polycythemia and congestive heart failure. Blood. 2008; 111: 3236–44
- Aragonés J, Schneider M, Van Geyte K, Fraisl P, Dresselaers T, Mazzone M, et al. Deficiency or inhibition of oxygen sensor Phd1 induces hypoxia tolerance by reprogramming basal metabolism. Nat Genet. 2008; 40: 170–80
- Clifton IJ, McDonough MA, Ehrismann D, Kershaw DJ, Granatino N, Schofield CJ. Structural studies on 2-oxoglutarate oxygenases and related double-stranded β-helix fold proteins. J Inorg Biochem. 2006; 100: 644–69
- Keskiaho K, Hieta R, Sormunen R, Myllyharju J. Chlamydomonas reinhardtii has multiple prolyl 4-hydroxylases, one of which is essential for proper cell wall assembly. Plant Cell. 2007; 19: 256–69
- Koski MK, Hieta R, Böllner C, Kivirikko KI, Myllyharju J, Wierenga RK. The active site of an algal prolyl 4-hydroxylase has a large structural plasticity. J Biol Chem. 2007; 282: 37112–23
- Hanauske-Abel HM, Popowicz AM. The HAG mechanism: a molecular rationale for the therapeutic application of iron chelators in human diseases involving the 2-oxoacid utilizing dioxygenases. Curr Med Chem. 2003; 10: 1005–19
- Tuckerman JR, Zhao Y, Hewitson KS, Tian Y-M, Pugh CW, Ratcliffe PJ, et al. Determination and comparison of specific activity of the HIF-prolyl hydroxylases. FEBS Lett. 2004; 576: 145–50
- Hirsilä M, Koivunen P, Xu L, Seeley T, Kivirikko KI, Myllyharju J. Effect of desferrioxamine and metals on the hydroxylases in the oxygen sensing pathway. FASEB J. 2005; 19: 1308–10
- Koivunen P, Hirsilä M, Kivirikko KI, Myllyharju J. The length of peptide substrates has a marked effect on hydroxylation by the hypoxia-inducible factor prolyl 4-hydroxylases. J Biol Chem. 2006; 281: 28712–20
- Koivunen P, Hirsilä M, Remes AM, Hassinen IE, Kivirikko KI, Myllyharju J. Inhibition of hypoxia-inducible factor (HIF) hydroxylases by citric acid cycle intermediates. Possible links between cell metabolism and stabilization of HIF. J Biol Chem. 2007; 282: 4524–32
- Ehrismann D, Flasman E, Genn DN, Mathioudakis N, Hewitson KS, Ratcliffe PJ, et al. Studies on the activity of the hypoxia-inducible-factor hydroxylases using an oxygen consumption assay. Biochem J. 2007; 401: 227–34
- McNeill LA, Flashmann E, Buck MRG, Hewitson KS, Clifton IJ, Jeschke G, et al. Hypoxia-inducible factor prolyl hydroxylase 2 has a high affinity for ferrous iron and 2-oxoglutarate. Mol Biosyst. 2005; 1: 321–4
- Acker H, Berchner-Pfannschmidt U, Wotzlaw C, Huckstorf C, Streller T. Optical analysis of the oxygen-sensing signal pathway. Oxygen Sensing, Responses and Adaptation to Hypoxia, S Lahiri, GL Semenza, NR Prabhakar. Marcel Dekker Inc, New York 2003; 507–21
- Kukkola L, Koivunen P, Pakkanen O, Page AP, Myllyharju J. Collagen prolyl 4-hydroxylase tetramers and dimers show identical decreases in Km values for peptide substrates with increasing chain length. Mutation of one of the two catalytic sites in the tetramer inactivates the enzyme by more than half. J Biol Chem. 2004; 279: 18656–61
- Huang J, Zhao Q, Mooney SM, Lee FS. Sequence determinants in hypoxia-inducible factor-1α for hydroxylation by the prolyl hydroxylases PHD1, PHD2, and PHD3. J Biol Chem. 2002; 277: 39792–800
- Li D, Hirsilä M, Koivunen P, Brenner MC, Xu L, Yang C, et al. Many amino acid substitutions in a hypoxia-inducible transcription factor (HIF)-1α-like peptide cause only minor changes in its hydroxylation by the HIF prolyl 4-hydroxylases. Substitution of 3,4-dehydroproline or azetidine-2-carboxylic acid for the proline leads to a high rate of uncoupled 2-oxoglutarate decarboxylation. J Biol Chem. 2004; 279: 55051–9
- Chan DA, Suthpin PD, Yen S-E, Giaccia AJ. Coordinate regulation of the oxygen-dependent degradation domains of hypoxia-inducible Factor 1α. Mol Cell Biol. 2005; 25: 6415–26
- Flashman E, Bagg EA, Chowdhury R, Mecinovic J, Loenarz C, McDonough MA, et al. Kinetic rationale for selectivity toward N- and C-terminal oxygen-dependent degradation domain substrates mediated by a loop region of hypoxia-inducible factor prolyl hydroxylases. J Biol Chem. 2008; 283: 3808–15
- Hon WC, Wilson MI, Harlos K, Claridge TDW, Schofield CJ, Pugh CW, et al. Structural basis for the recognition of hydroxyproline in HIF-1α by pVHL. Nature. 2002; 417: 975–8
- Kuznetsova AV, Meller J, Schnell PO, Nash JA, Ignacak ML, Sanchez Y, et al. von Hippel-Lindau protein binds hyperphosphorylated large subunit of RNA polymerase II through a proline hydroxylation motif and targets it for ubiquitination. Proc Natl Acad Sci USA. 2003; 100: 2706–11
- Hanson ES, Rawlins ML, Leibold EA. Oxygen and iron regulation of iron regulatory protein 2. J Biol Chem. 2003; 278: 40337–42
- Wang J, Chen G, Muckenthaler M, Galy B, Hentze MW, Pantopoulos K. Iron-mediated degradation of IRP2, an unexpected pathway involving a 2-oxoglutarate-dependent oxygenase activity. Mol Cell Biol. 2004; 24: 954–65
- Cummins EP, Berra E, Comerford KM, Ginouves A, Fitzgerald KT, Seeballuck F, et al. Prolyl hydroxylase-1 negatively regulates IκB kinase-β, giving insight into hypoxia-induced NFkB activity. Proc Natl Acad Sci U S A. 2006; 28: 18154–9
- Köditz J, Nesper J, Stiehl DP, Camenisch G, Franke C, Myllyharju J, et al. Oxygen-dependent ATF-4 stability is mediated by the PHD3 oxygen sensor. Blood. 2007; 110: 3610–7
- Masson N, Appelhoff RJ, Tuckerman JR, Tian YM, Demol H, Puype M, et al. The HIF prolyl hydroxylase PHD3 is a potential substrate of the TRiC chaperonin. FEBS Lett. 2004; 570: 166–70
- Nakayama K, Frew IJ, Hagensen M, Skals M, Habelhah H, Bhoumik A, et al. Siah2 regulates stability of prolyl-hydroxylases, controls HIF1α abundance, and modulates physiological responses to hypoxia. Cell. 2004; 117: 941–52
- Nakayama K, Gazdoiu S, Abraham R, Pan ZQ, Ronai Z. Hypoxia-induced assembly of prolyl hydroxylase PHD3 into complexes: implications for its activity and susceptibility for degradation by the E3 ligase Siah2. Biochem J. 2007; 401: 217–26
- Baek JH, Mahon PC, Oh J, Kelly B, Krishnamachary B, Pearson M, et al. OS-9 interacts with hypoxia-inducible factor 1α and prolyl hydroxylases to promote oxygen-dependent degradation of HIF-1α. Mol Cell. 2005; 17: 503–12
- Ozer A, Wu LC, Bruick RK. The candidate tumor suppressor ING4 represses activation of the hypoxia inducible factor (HIF). Proc Natl Acad Sci U S A. 2005; 102: 7481–6
- Hopfer U, Hopfer H, Jablonski K, Stahl RA, Wolf G. The novel WD-repeat protein Morg1 acts as a molecular scaffold for hypoxia-inducible factor prolyl hydroxylase 3 (PHD3). J Biol Chem. 2006; 281: 8645–55
- Barth S, Nesper J, Hasgall PA, Wirthner R, Nytko KJ, Edlich F, et al. The peptidyl prolyl cis/trans isomerase FKBP38 determines hypoxia-inducible transcription factor prolyl-4-hydroxylase PHD2 protein stability. Mol Cell Biol. 2007; 27: 3758–68
- Hewitson KS, Schofield CJ. The HIF pathway as a therapeutic target. Drug Discov Today. 2004; 9: 704–11
- Bruegge K, Jelkmann W, Metzen E. Hydroxylation of hypoxia-inducible transcription factors and chemical compounds targeting the HIF-α hydroxylases. Curr Med Chem. 2007; 14: 1853–62
- Linden T, Katschinski DM, Eckhardt K, Scheid A, Pagel H, Wenger RH. The antimycotic ciclopirox olamine induces HIF-1α stability, VEGF expression, and angiogenesis. FASEB J. 2003; 17: 761–3
- Yuan Y, Hilliard G, Ferguson T, Millhorn DE. Cobalt inhibits the interaction between hypoxia inducible factor-α and von Hippel-Lindau protein by direct binding to hypoxia inducible factor-α. J Biol Chem. 2003; 278: 15911–6
- Salnikow K, Donald SP, Bruick RK, Zhitkovich A, Phang JM, Kasprzak KS. Depletion of intracellular ascorbate by the carcinogenic metals nickel and cobalt results in the induction of hypoxic stress. J Biol Chem. 2004; 279: 40337–44
- Cao M, Westerhausen-Larson A, Niyibizi C, Kavalkovich K, Georgescu HI, Rizzo CF, et al. Nitric oxide inhibits the synthesis of type-II collagen without altering Col2A1 mRNA abundance: prolyl hydroxylase as a possible target. Biochem J. 1997; 324: 305–10
- Metzen E, Zhou J, Jelkmann W, Fandrey J, Brüne B. Nitric oxide impairs normoxic degradation of HIF-1α by inhibition of prolyl 4-hydroxylases. Mol Biol Cell. 2003; 14: 3470–81
- Berchner-Pfannschmidt U, Yamac H, Trinidad B, Fandrey J. Nitric oxide modulates oxygen sensing by hypoxia-inducible factor 1-dependent induction of prolyl hydroxylase 2. J Biol Chem. 2007; 282: 1788–96
- Gerald D, Berra E, Frapart YM, Chan DA, Giaccia AJ, Mansuy D, et al. JunD reduces tumor angiogenesis by protecting cells from oxidative stress. Cell. 2004; 118: 781–94
- Cash TP, Pan Y, Simon MC. Reactive oxygen species and cellular oxygen sensing. Free Radic Biol Med. 2007; 43: 1219–25
- Pan Y, Mansfield KD, Bertozzi CC, Rudenko V, Chan DA, Giaccia AJ, et al. Multiple factors affecting cellular redox status and energy metabolism modulate hypoxia-inducible factor prolyl hydroxylase activity in vivo and in vitro. Mol Cell Biol. 2007; 27: 912–25
- Mole DR, Schlemminger I, McNeill LA, Hewitson KS, Pugh CW, Ratcliffe PJ, et al. 2-oxoglutarate analogue inhibitors of HIF prolyl hydroxylase. Bioorg Med Chem Lett. 2003; 13: 2677–80
- Schlemminger I, Mole DR, McNeill LA, Dhanda A, Hewitson KS, Tian Y-M, et al. Analogues of dealanylalahopcin are inhibitors of human HIF prolyl hydroxylases. Bioorg Med Chem Lett. 2003; 13: 1451–4
- Warshakoon NC, Wu S, Boyer A, Kawamoto R, Sheville J, Renock S, et al. Structure-based design, synthesis, and SAR evaluation of a new series of 8-hydroxyquinolines as HIF-1alpha prolyl hydroxylase inhibitors. Bioorg Med Chem Lett. 2006; 16: 5517–22
- Warshakoon NC, Wu S, Boyer A, Kawamoto R, Sheville J, Renock S, et al. A novel series of imidazo[1,2-a]pyridine derivatives as HIF-1α prolyl hydroxylase inhibitors. Bioorg Med Chem Lett. 2006; 16: 5598–601
- Warshakoon NC, Wu S, Boyer A, Kawamoto R, Renock S, Xu K, et al. Design and synthesis of a series of novel pyrazolopyridines as HIF 1-α prolyl hydroxylase inhibitors. Bioorg Med Chem Lett. 2006; 16: 5687–90
- Warshakoon NC, Wu S, Boyer A, Kawamoto R, Sheville J, Bhatt RT, et al. Design and synthesis of substituted pyridine derivatives as HIF-1α prolyl hydroxylase inhibitors. Bioorg Med Chem Lett. 2006; 16: 5616–20
- Nangaku M, Izuhara Y, Takizawa S, Yamashita T, Fujii-Kuriyama Y, Ohneda O, et al. A novel class of prolyl hydroxylase inhibitors induces angiogenesis and exerts organ protection against ischemia. Arterioscler Thromb Vasc Biol. 2007; 27: 2548–54
- Isaacs JS, Jung YJ, Mole DR, Lee S, Torres-Cabala C, Chung Y-L, et al. HIF overexpression correlates with biallelic loss of fumarate hydratase in renal cancer: novel role of fumarate in regulation of HIF stability. Cancer Cell. 2005; 8: 143–53
- Lu H, Dalgard CL, Mohyeldin A, McFate T, Tait AS, Verma A. Reversible inactivation of HIF-1 prolyl hydroxylases allows cell metabolism to control basal HIF-1. J Biol Chem. 2005; 280: 41928–39
- Selak MA, Armour SM, MacKenzie ED, Boulahbel H, Watson DG, Mansfield KD, et al. Succinate links TCA cycle dysfunction to oncogenesis by inhibiting HIF-α prolyl hydroxylase. Cancer Cell. 2005; 7: 77–85
- Hewitson KS, Liénard BM, McDonough MA, Clifton IJ, Butler D, Soares AS, et al. Structural and mechanistic studies on the inhibition of the hypoxia-inducible transcription factor hydroxylases by tricarboxylic acid cycle intermediates. J Biol Chem. 2007; 282: 3293–301
- Pollard PJ, Brière JJ, Alam NA, Barwell J, Barclay E, Wortham NC, et al. Accumulation of Krebs cycle intermediates and over-expression of HIF1α in tumours which result from germline FH and SDH mutations. Hum Mol Genet. 2005; 14: 2231–9
- Franklin TJ. Therapeutic approaches to organ fibrosis. Int J Biochem Cell Biol. 1997; 29: 79–89
- Grimm C, Hermann DM, Bogdanova A, Hotop S, Kilic Ü, Wenzel A, et al. Neuroprotection by hypoxic preconditioning: HIF-1 and erythropoietin protect from retinal degeneration. Semin Cell Dev Biol. 2005; 16: 531–8
- Nangaku M, Eckardt K-U. Hypoxia and the HIF system in kidney disease. J Mol Med. 2007; 85: 1325–30
- Prass K, Scharff A, Ruscher K, Löwl D, Muselmann C, Victorov I, et al. Hypoxia-induced stroke tolerance in the mouse is mediated by erythropoietin. Stroke. 2003; 34: 1981–6
- Ratan RR, Siddiq A, Aminova L, Lange PS, Langley B, Ayoub I, et al. Translation of ischemic preconditioning to the patient: prolyl hydroxylase inhibition and hypoxia inducible factor-1 as novel targets for stroke therapy. Stroke. 2004; 35: 2687–9
- Baranova O, Miranda LF, Pichiule P, Dragatsis I, Johnson RS, Chavez JC. Neuron-specific inactivation of the hypoxia inducible factor 1α increases brain injury in a mouse model of transient focal cerebral ischemia. J Neurosci. 2007; 27: 6320–32
- Cai Z, Zhong H, Bosch-Marce M, Fox-Talbot K, Wang L, Wei C, et al. Complete loss of ischaemic preconditioning-induced cardioprotection in mice with partial deficiency of HIF-1α. Cardiovasc Res. 2008; 77: 463–70
- Ockaili R, Natarajan R, Salloum F, Fisher BJ, Jones D, Fowler III AA, et al. HIF-1 activation attenuates postischemic myocardial injury: role for heme oxygenase-1 in modulating microvascular chemokine generation. Am J Physiol Heart Circ Physiol. 2005; 289: H542–8
- Sridharan V, Guichard J, Bailey RM, Kasiganesan H, Beeson C, Wright GL. The prolyl hydroxylase oxygen-sensing pathway is cytoprotective and allows maintenance of mitochondrial membrane potential during metabolic inhibition. Am J Physiol Cell Physiol. 2007; 292: C719–28
- Hill P, Shukla D, Tran MGB, Aragones J, Cook HT, Carmeliet P, et al. Inhibition of hypoxia inducible factor hydroxylases protects against renal ischemia-reperfusion injury. J Am Soc Nephrol. 2008; 19: 39–46
- Siddiq A, Ayoub IA, Chavez JC, Aminova L, Shah S, LaManna JC, et al. Hypoxia-inducible factor prolyl 4-hydroxylase inhibition. A target for neuroprotection in the central nervous system. J Biol Chem. 2005; 280: 41732–43
- Philipp S, Jürgensen JS, Fielitz J, Bernhardt WM, Weidemann A, Schiche A, et al. Stabilization of hypoxia inducible factor rather than modulation of collagen metabolism improves cardiac function after acute myocardial infarction in rats. Eur J Heart Fail. 2006; 8: 347–54
- Bernhardt WM, Câmpean V, Kany S, Jürgensen J-S, Weidemann A, Warnecke C, et al. Preconditional activation of hypoxia-inducible factors ameliorates ischemic acute renal failure. J Am Soc Nephrol. 2006; 17: 1970–8
- Langsetmo I, Jacob C, Ho W-B, Stephenson R, Sirenko O, Sidhu P, et al. Inhibition of HIF prolyl hydroxylases with FG-4539 is neuroprotective in a mouse model of permanent focal ischemia. Stroke. 2006; 37: 731
- Wang Q, Guo R, Winmill R, Stephenson R, Pacleb J, Bin-Ho W, , et al. Renoprotective and therapeutic efficacy of the HIF prolyl hydroxylase inhibitor FG-4539 in experimental ischemia-reperfusion induced acute kidney injury. Am Soc Nephrol Renal Week 2006, Abstract TH-P01025.
- Macdougall IC, Eckardt K-U. Novel strategies for stimulating erythropoiesis and potential new treatments for anaemia. Lancet. 2006; 368: 947–53
- Bunn HF. New agents that stimulate erythropoiesis. Blood. 2007; 109: 868–73
- Klaus S, Langsetmo I, Flippin L, Arend M, Seeley T, Winmill R, , et al Induction of erythropoiesis in rodents by novel and distinct families of orally active HIF prolyl hydroxylase inhibitors. Keystone Symposia in Molecular, Cellular, Physiological, and Pathogenic Responses to Hypoxia; 15–20 Jan, 2008;, Vancouver, Canada. Abstract 301.
- Seeley TW, Sternlicht MD, Stephenson R, Arend M, Flippin L, Liu DY, , et al HIF prolyl hydroxylase inhibitors increase erythropoiesis without promotion of tumor progression in the presence or absence of concomitant chemotherapy. Keystone Symposia in Molecular, Cellular, Physiological, and Pathogenic Responses to Hypoxia; 15–20 Jan, 2008;, Vancouver, Canada. Abstract 364.
- Hsieh MM, Linde NS, Wynter A, Metzger M, Wong C, Langsetmo I, et al. HIF prolyl hydroxylase inhibition results in endogenous erythropoietin induction, erythrocytosis, and modest fetal hemoglobin expression in rhesus macaques. Blood. 2007; 110: 2140–7
- Wiecek A, Piecha G, Ignacy W, Schmidt R, Neumayer HH, Scigalla P, et al. Pharmacological stabilization of HIF increases hemoglobin concentration in anemic patients with chronic kidney disease. Nephrol Dial Transplant 2005; 20(suppl 5)v195
- Frohna PA, Milwee S, Pinkett J, Lee T, Moore-Perry K, Chou J, , et al. Results from a randomized, single-blind, placebo-controlled trial of FG-4592, a novel hypoxia inducible factor prolyl hydroxylase inhibitor, in CKD anemia. Am Soc Nephrol Renal Week 2007, Abstract SU-PO806.
- Bernhardt WM, Wiesener MS, Schmieder RE, Günzler V, Eckardt KU. The prolyl hydroxylase inhibitor FG-2216 stimulates EPO production in nephric and anephric dialysis patients—evidence for underutilized production capacity in liver and kidneys. Am Soc Nephrol Renal Week 2007, Abstract SA-PO784.
- Pugh CW, Ratcliffe PJ. Regulation of angiogenesis by hypoxia: role of the HIF system. Nat Med. 2003; 9: 677–84
- Vincent KA, Jiang C, Boltje I, Kelly RA. Gene therapy progress and prospects: therapeutic angiogenesis for ischemic cardiovascular disease. Gene Ther. 2007; 14: 781–9
- Pajusola K, Künnapuu J, Vuorikoski S, Soronen J, André H, Pereira T, et al. Stabilized HIF-1α is superior to VEGF for angiogenesis in skeletal muscle via adeno-associated virus gene transfer. FASEB J. 2005; 19: 1365–7
- Warnecke C, Griethe W, Weidemann A, Jürgensen JS, Willam C, Bachmann S, et al. Activation of the hypoxia-inducible factor-pathway and stimulation of angiogenesis by application of prolyl hydroxylase inhibitors. FASEB J. 2003; 17: 1186–8
- Asikainen TM, Schneider BK, Waleh NS, Clyman RI, Ho WB, Flippin LA, et al. Activation of hypoxia-inducible factors in hyperoxia through prolyl 4-hydroxylase blockade in cells and explants of primate lung. Proc Natl Acad Sci USA. 2005; 102: 10212–7
- Asikainen TM, Chang LY, Coalson JJ, Schneider BK, Waleh NS, et al. Improved lung growth and function through hypoxia-inducible factor in primate chronic lung disease of prematurity. FASEB J. 2006; 20: 1698–700