Abstract
Background. Visfatin/PBEF/Nampt is an adipose-derived hormone proposed to exert insulin-mimicking effects and play a positive role in attenuating insulin resistance. However, the precise mechanisms underlying the beneficial effects of visfatin/PBEF/Nampt on insulin sensitivity remain unknown.
Method. Euglycemic-hyperinsulinemic clamps were used in the same groups of rats to study the in vivo effect of visfatin/PBEF/Nampt on insulin sensitivity and glucose/lipid metabolism before and after the overexpression of visfatin/PBEF/Nampt protein, which was carried out by injection of pcDNA3.1-visfatin plasmid.
Results. On day 4 after plasmid injection, plasma visfatin/PBEF/Nampt protein levels were significantly increased and displayed a hypocholesterolemic effect in both normal-chow (NC) and high-fat diet (HT) animals with pcDNA3.1-visfatin treatment. A second glucose clamp also demonstrated increased insulin sensitivity in pcDNA3.1-visfatin animals. Consistent with the clamp data, the extent of insulin receptor substrate (IRS)-1 tyrosine phosphorylation in response to insulin was significantly enhanced in the liver and adipose tissues. In addition, the mRNA expression of peroxisome proliferator-activated receptor-γ (PPARγ) and sterol regulatory element-binding proteins 2 (SREBP-2) in the liver and adipose tissues was also significantly upregulated in these animals.
Conclusion. These results demonstrate that visfatin/PBEF/Nampt improves insulin sensitivity and exerts its hypocholesterolemic effects at least partially through upregulation of the tyrosine phosphorylation of IRS-1 protein and the mRNA levels of PPARγ and SREBP-2.
Introduction
Type 2 diabetes mellitus (T2DM) is a genetically heterogeneous disorder characterized by peripheral insulin resistance and reduced insulin secretion from pancreatic beta cells in the late stage of the disorder Citation[1–3]. Despite extensive studies, the underlying mechanism of these defects remains elusive. Several polypeptide hormones have been discovered that could represent functional links between energy balance and insulin resistance. These polypeptides include leptin, resistin, adiponectin, visfatin, apelin, and others Citation[4–9]. Among these polypeptide hormones, visfatin was recently identified as a novel adipocytokine implicated in the development of obesity-associated insulin resistance and diabetes mellitus Citation[10]. Visfatin is a nicotinamide phosphoribosyltransferase (Nampt). It was originally reported to be a cytokine that promotes β cell maturation and therefore named ‘pre-β cell colony-enhancing factor’ (PBEF) Citation[11]. Thus, visfatin, PBEF, and Nampt refer to an identical protein with multiple biological functions.
Key messages
Visfatin/PBEF/Nampt is an adipose-derived hormone proposed to exert insulin-mimicking effects and play a positive role in attenuating insulin resistance.
The precise mechanisms underlying the beneficial effects of visfatin/PBEF/Nampt on insulin sensitivity remain largely unknown.
Using twice EU clamps in animals serving as their own control, we investigated the in vivo effect of visfatin/PBEF/Nampt on insulin sensitivity and glucose-lipid metabolism by overexpressing visfatin/PBEF/Nampt protein in rats.
Visfatin/PBEF/Nampt is preferentially expressed in visceral fat of both humans and animals as compared with subcutaneous fat. In humans, plasma visfatin/PBEF/Nampt concentrations correlate strongly with the amount of visceral fat. Most interestingly, visfatin mimics insulin effects in various rodent models of insulin resistance and obesity in vivo. A study by Fukuhara et al. showed that visfatin/PBEF/Nampt directly bound to and stimulated the insulin receptor through a different binding site of insulin Citation[10]. However, the action of visfatin/PBEF/Nampt on the insulin receptor needs to be further confirmed by other groups because the original study by Fukuhara et al. was retracted later on by the authors Citation[12]. Another study by Beltowski et al. showed that acute intravenous administration of visfatin reduced plasma glucose levels without affecting plasma insulin concentrations, which suggests that the hypoglycemic effect of visfatin/PBEF/Nampt is not through an insulin-stimulating mechanism Citation[13]. In addition, visfatin/PBEF/Nampt stimulates the differentiation of preadipocytes to mature fat cells, induces triglyceride accumulation, accelerates triglyceride synthesis from glucose, and induces the expression of genes encoding the adipose tissue-specific markers peroxisome proliferator-activated receptor-γ (PPARγ), fatty acid synthase, diacylglycerol acyltransferase, and adiponectin Citation[14]. In humans, plasma visfatin/PBEF/Nampt levels correlate significantly with percent body fat, body mass index, and visfatin/PBEF/Nampt mRNA level in visceral adipose tissue, but not with visceral fat mass or waist-to-hip ratio (WHR) Citation[14]. In two recent studies, plasma visfatin/PBEF/Nampt was shown higher in patients with T2DM than in normoglycemic controls Citation[15], Citation[16]. These data suggest that endogenous visfatin/PBEF/Nampt is involved in the regulation of glucose and lipid homeostasis.
The role of visfatin/PBEF/Nampt in the regulation of glucose and lipid homeostasis is arguable because several studies reported that plasma visfatin/PBEF/Nampt was reduced in obese patients and was not related to insulin resistance Citation[17]. The circulating visfatin level was also shown to correlate with inflammation, but not with insulin resistance Citation[18]. Thus, the relationship between visfatin/PBEF/Nampt and insulin resistance remains inconclusive. To further characterize the physiological role of visfatin/PBEF/Nampt, we examined the effects of overexpression of visfatin/PBEF/Nampt on glucose/lipid metabolism and insulin sensitivity in rats. We also examined the effects of visfatin/PBEF/Nampt on insulin receptor substrate (IRS-1), fibroplast growth factor-21 (FGF-21), and gene expression of selected metabolic enzymes.
Materials and methods
Construction of recombinant plasmids
The full-length visfatin cDNA (1476 bp) was amplified from rat adipose tissue using the 5′-GCGAATTCG CCACCATGAA T G CTGCGGCAGAAGC-3′ sense and 5′-GCC TCGAGCTAGTGAG GCGCCACATCCT-3′ antisense primers by Reverse Transcription Polymerase Chain Reaction (RT-PCR). PCR products and pcDNA3.1(+) (Invitrogen, Carlsbad, CA) were digested with EcoR I and Xho I. The 1476 bp (visfatin cDNA) and 5428 bp (pcDNA3.1 + ) fragments were collected in low melting point agarose, then ligated using T4 ligase at 16°C overnight. Escherichia coli (DH-5α) was transformed using the ligation products. Recombinant plasmids (pcDNA3.1-visfatin) were purified from transformed E. coli, screened on ampicillin plates, and verified by restriction endonuclease digestion and DNA sequencing.
Animals
The animal studies presented here were conducted in accordance with National Institutes of Health (NIH) Guidelines for Animal Care and approved by Chongqing Medical University. Four-week-old male sprague-drawley rat (SD) rats (EA Center, Chongqing, China) with average body-weight of 120 g were used in this study. Rats were housed individually in controlled light/dark (12 h/12 h) and temperature conditions with free access to water and standard rat chow for 3 days. Rats were then randomly divided into three groups. Two groups (n=10 for each) were fed a normal-chow diet containing 60% carbohydrate, 21% protein, and 19% fat. The third group (n=10) was fed a high-fat diet containing 54% fat, 33% carbohydrate, and 13% protein. The content of fiber, vitamins, and minerals was the same in both diets.
Animal studies
The euglycemic-hyperinsulinemic clamps (EU clamp) were performed 16 weeks after the initiation of the special diet. Four days prior to the clamp study, animals were catheterized through the right jugular vein and the left carotid artery under pentobarbital sedation (50 mg/kg; Nembutal Abbott Laboratories, Abbott Park, IL). Catheters were ‘tunneled’ subcutaneously, exteriorized at the back of neck, and filled with heparinized saline. The jugular and carotid catheters were used for blood sampling and infusion, respectively.
On postoperative day 4, EU clamps were performed in awake and unrestrained rats as previously described Citation[19]. Briefly, insulin (4.8 mU kg−1 min−1) was infused through the carotid artery catheter from 0 to 120 min during the EU clamps. Glucose concentrations were clamped at euglycemic levels by a variable rate infusion of 25% glucose. Blood glucose levels were monitored with a portable glucometer, and glucose infusion rates were adjusted every 5–10 min as needed. Blood samples (400 µL) were obtained from the jugular vein at 0 and 180 min for determination of levels of insulin, visfatin/PBEF/Nampt, free fatty acids (FFA), triglyceride (TG), and total cholesterol (TC). Whole-body insulin sensitivity was determined by the rate of glucose infusion that maintains euglycemia (glucose infusion rate, GIR).
At the end of the clamp, one group of the normal-chow fed rats (NT group; n=10) was given pcDNA3.1-visfatin plasmid at a dose of 300 µg in 300 µL phosphate buffer system (PBS) Another group of the normal-chow fed rats (NC group; n=10) was given pcDNA3.1(+) at the same dose. High-fat-fed rats (HT group; n=10) were given pcDNA3.1-visfatin plasmid at a dose of 300 µg in 300 µL PBS. For all three groups, the DNA was injected into the rectus femoris muscle using a disposable insulin syringe with a sterile 29-gauge needle. On postinjection day 4, EU clamps were performed again in the animals as described above to access the insulin sensitivity. At the end of the studies, rats were euthanized with overdosing of pentobarbital (180 mg/kg) after terminal blood sampling at 120 min. Tissues were harvested, immediately frozen in liquid nitrogen and stored at -80°C until analysis.
Western blot analysis
Plasma visfatin/PBEF/Nampt levels were quantified by Western blot analysis. One microliter of plasma was subjected to 12% sodium dodecyl sulfate polyacrylamide gel electrophoresis (SDS-PAGE), transferred, blotted with primary goat anti-rat antibody for visfatin/PBEF/Nampt and FGF-21 (Santa Cruz Biotechnology, Inc., CA, USA) (1:5000 dilution). Secondary antibodies consisted of goat anti-rabbit Horseradish Peroxidase (HRP)-conjugated antibodies (Santa Cruz Biotechnology, Inc., CA, USA) used with enhanced chemiluminescent (ECL) reagents (Perkin Elmer, MA, USA). Binding was imaged on Kodak Maximum Sensitivity (MS) films and quantified with a Bio Imaging System Densitometer (Bio-Rad, CA, USA). The plasma of normal rat was used as the positive control and water as the negative control (data not shown).
RT-PCR analysis of gene expression
Total RNA was isolated from adipose tissue, liver, or muscle using Trizol reagent (Invitrogen, Carlsbad, CA, USA). RNA was quantified by A260, and its integrity verified by agarose gel electrophoresis using ethidium bromide for visualization. Reverse transcription was performed on 1 µg total RNA using RTase Moloney Murine Leukemia Virus Reverse Transcriptase (M-MLV) (RNase H, TaKaRa Bio Co. Ltd, Dalian) and Oligo deoxy-thymine (dT) 18 Primer (TaKaRa Bio Bio Co. Ltd, Dalian). The PCR reaction mixture contained in a final volume of 2 µL of the first strand cDNA, 2 µL of 10 pmol specific primes, 4 µL of 2.5 mM dNTP, 5 µL 10×buffer, 2.5 U Taq DNA polymerase, 3 µL of 2.5 mM MgCl2, dH2O upto 50 µL. Samples were incubated for an initial denaturation at 94°C for 3 min followed by 1 cycle consisting of 94°C for 30 s for degeneration, 55°C for 30 s for annealing, and 72°C for 40 s for extension. The final cycle included a prolonged extension phase performed at 72°C for 5 min for more intense extension. The intensities of PCR bands were quantified with a Bio Imaging System Densitometer (Bio-Rad, CA, USA). The sequences, product lengths, and annealing temperatures of the primers are shown in .
Table I. Characteristics of the specific primers used for Reverse Transcription Polymerase Chain Reaction (RT-PCR) analysis.
IRS-1 tyrosine phosphorylation assays
Dot-blot assay was used to study the effects of visfatin/PBEF/Nampt overexpression on insulin-stimulated tyrosine phosphorylation. To maximally stimulate protein tyrosine phosphorylation in adipose and liver tissues, insulin was injected via cardiac puncture at the dose of 0.5 U/kg body-weight 4 days after plasmid treatment. Epididymal adipose tissue and liver were harvested after 5 min and snap-frozen in liquid N2.
A total of 100 mg of liver or adipose tissue was homogenized in liquid nitrogen and treated with lysis buffer (150 mmol/L NaCl, 1% Nonidet P40 Lysis Buffer (NP-40), 0.5% deoxycholic acid sodium, and 50 mmol/L Tris, pH 8.0). The lysates were clarified by centrifugation (20,000 g at 4°C for 3 min). A total of 100 µL of supernatant was blotted onto a polyvinylidene fluoride (PVDF) membrane (0.22 µm) by vacuum using a Bio-Dot blotting apparatus (Bio-Rad, CA, USA). The membrane was blocked with buffer A (Tris Buffered Saline with Tween 20 (TBST) containing 1% blot-qualified bovine serum albumin (BSA)) at 30°C for 120 min. The membrane was incubated for 2 h at 30°C in buffer A containing a rabbit anti-IRS-1 phosphotyrosine antibody (IRS-1 tyrosine-632; Santa Cruz Biotechnology, Inc., CA). The membrane was then washed three times with TBST for 10 min before being incubated for 30 min at 30°C with horseradish peroxidase-labeled sheep anti-rabbit Immunoglobulin G (IgG) antibody (Santa Cruz Biotechnology, Inc., CA) diluted 1:5000 in TBST. Washing in TBST was repeated as described above, and the reactive dots were identified after a 5-min incubation in ECL, prepared according to the manufacturer's instructions. The intensity of the dots was quantified with a Bio Imaging System Densitometer (Bio-Rad, CA, USA).
Analytical procedures
Plasma insulin was measured in deproteinized serum by radioimmunoassay using rat insulin as standard (Linco, St. Charles, Missouri). Free-fatty acid (FFA) was measured in plasma with a kit from Wako Pure Chemicals, Richmond, VA. Plasma glucose was measured using the glucose oxidase method. Plasma triglyceride, total cholesterol, low-density lipoprotein cholesterol (LDL-C), and high-density lipoprotein cholesterol (HDL-C) concentrations were determined enzymatically.
Statistical analysis
Data are presented as mean±SE. A repeated measures analysis of variance (repeated-measures ANOVA) was used to assess the results measured at consecutive multiple time points. A two-way design was used to incorporate additional effects of different experimental groups followed by a post hoc least significant difference (PLSD) test to compare two individual groups. Within-group comparisons were made using the paired Student's t test. Differences were considered statistically significant at P<0.05. All analyses were performed using SPSS (SPSS graduate pack; SPSS, Chicago, IL).
Results
Metabolic parameters
Compared to rats fed a regular diet (NC and NT), rats fed a high fat diet (HT) had significantly increased body-weight and plasma concentrations of FFA, triglycerides (TG), total cholesterol (TC), high-density lipoprotein cholesterol (HDL-C), and low-density lipoprotein cholesterol (LDL-C) (). On day 3 following plasmid injection, however, plasma levels of TC, HDL-C, LDL-C, and insulin were significantly decreased in the HT group (P < 0.05). A decrease in TC and HDL-C levels was also observed in the NT group (P < 0.05).
Table II. Laboratory data in rats injected with pcDNA3.1-visfatin versus control pcDNA3.1.
Plasma levels of visfatin/PBEF/Nampt
To study the effects of visfatin/PBEF/Nampt in vivo, we overexpressed the visfatin protein by injecting male Sprague-Dawley rats with pcDNA3.1-visfatin or control pcDNA3.1. The overexpression was carried out by a recombinant plasmid expressing the rat visfatin/PBEF/Nampt cDNA from a the cytomegalovirus (CMV) promoter as described under the heading ‘Materials and methods’. To confirm the high level of visfatin/PBEF/Nampt protein expression in vivo, we performed Western blotting on the plasma samples obtained 4 days after the injection. As shown in , injection of pcDNA3.1-visfatin resulted in an increase in plasma levels of visfatin/PBEF/Nampt in the NT group (from 0.99±0.08 to 1.54±0.06 arbitrary units, P <0.01) and in the HT group (from 0.99±0.16 to 1.92±0.18 arbitrary units, P < 0.01). This effect was not observed in the NC group injected with pcDNA3.1(+) (0.99±0.05 versus 0.99±0.04 arbitrary units, P > 0.05).
Figure 1. Plasma visfatin/PBEF/Nampt protein levels in normal controls treated with pcDNA3.1(+) (NC; n=5), normal-chow rats treated with pcDNA3.1-visfatin (NT; n=5), and high-fat-fed rats treated with pcDNA3.1-visfatin (HT; n=5) for 4 days. Each experiment was performed in triplicate. Plasma samples were taken under following experimental conditions: Lane 1, basal values; Lane 2, at the end of first clamp; Lane 3, day 4 after plasmid injection; and Lane 4, at the end of second clamp.
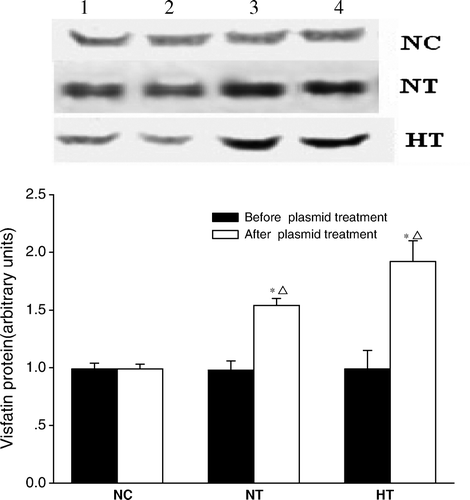
The expression of FGF-21 mRNA and its plasma protein levels
Both plasma FGF-21 levels and hepatic expression of FGF-21 mRNA were significantly decreased in the NT group compared with the NC group (0.50±0.02 versus 0.66±0.04, P < 0.05; and 1.88±0.11 versus 3.04±0.11, P < 0.01, respectively; all data are expressed in arbitrary units). These differences were not detected between the HT and the NC groups ().
Figure 2. Effects of visfatin/PBEF/Nampt overexpression on fibroplast growth factor-21 (FGF-21) mRNA expression and its plasma protein levels. A: Plasma FGF-21 protein levels (n=5 in each group, and each experiment was performed in triplicate); B: FGF-21 mRNA expression in liver tissue (n=5 in normal-chow diet with pcDNA3.1(+) plasmid infusion (NC) group; n=6 in normal-chow diet with pcDNA3.1-visfatin plasmid infusion (NT) and High-fat diet with pcDNA3.1-visfatin plasmid infusion (HT) group; each experiment was performed in triplicate).
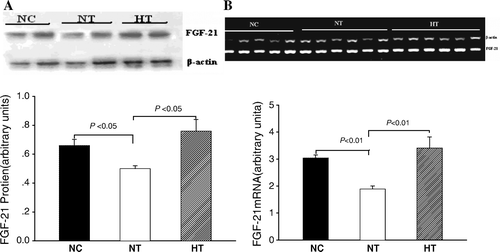
Euglycemic-hyperinsulinemic clamp
To directly examine the quantitative effect of visfatin overexpression on insulin sensitivity, we performed euglycemic-hyperinsulinemic clamps on the same group of rats before and after the plasmid injection. Before the plasmid injection, there was no statistic difference in glucose infusion rate (GIR) required to maintain euglycemia among the three groups of rats. Injection of pcDNA3.1-visfatin resulted in a significant increase in GIR in the NT rats (by 28%) and in the HT rats (by 36%). The GIR did not change in the NC rats injected with pcDNA3.1(+) ( and ).
Figure 3. Glucose infusion rates (GIR) during EU clamping in normal controls treated with pcDNA3.1(+) (NC; n=10), normal-chow rats treated with pcDNA3.1-visfatin (NT; n=10), and high-fat-fed rats treated with pcDNA3.1-visfatin (HT; n=10) for 3 days. ▪ Before plasmid treatment; □ After plasmid treatment; * indicates P < 0.01 before and after injection of plasmid.
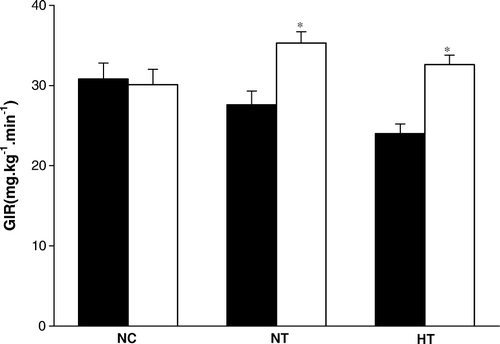
RT-PCR analysis of gene expression
Compared to NC (0.68±0.09 arbitrary units), hepatic expression of PPARγ mRNA was significantly upregulated in NT (0.98±0.08 arbitrary units, P < 0.05) and HT (1.59±0.15 arbitrary units, P < 0.01). PPARγ mRNA expression in adipose tissues was significantly upregulated in the NT compared to NC (0.56±0.04 versus 0.27±0.07 arbitrary units, P < 0.01). No difference was detected between the HT and the NC groups. Hepatic expression of SREBP-2 mRNA was significantly increased in both NT and HT groups compared to the NC (0.37±0.06 and 0.36±0.07 versus 0.21±0.04 arbitrary units, both P < 0.05). Expression of hepatic 3-hydroxy-3-methyl-glutaryl-CoA reductase (HMG CoA) mRNA was higher in the NT than in the NC (0.89±0.12 versus 0.37±0.07 arbitrary units, P < 0.01). There was no difference in the hepatic mRNA levels of SREBP-2 and HMG CoA between the HT and the NC groups (). The mRNA expression of PEPCK (phosphoenolypyruvate carboxykinase), SCD-1 (stearoylcoenzyme A desaturase-1), ATGL (adipose triglyceride lipase), and HSL (hormone-sensitive lipase) in the liver and adipose tissue was not different among the three groups (data not shown).
Figure 4. Effects of visfatin/PBEF/Nampt overexpression on peroxisome proliferator-activated receptor-gamma (PPARγ) and sterol regulatory element binding proteins 2 (SREBP-2) mRNA expressions. A: PPARγ mRNA expression in the liver; B: PPARγ mRNA expression in adipose tissues; C: SREBP-2 mRNA expression in the liver; D: 3-hydroxy-3-methyl-glutaryl-CoA reductase (HMG CoA) mRNA expression in the liver. NC: normal-chow diet with pcDNA3.1(+) plasmid infusion; NT: normal-chow diet with pcDNA3.1-visfatin plasmid infusion; HT: High-fat diet with pcDNA3.1-visfatin plasmid infusion.
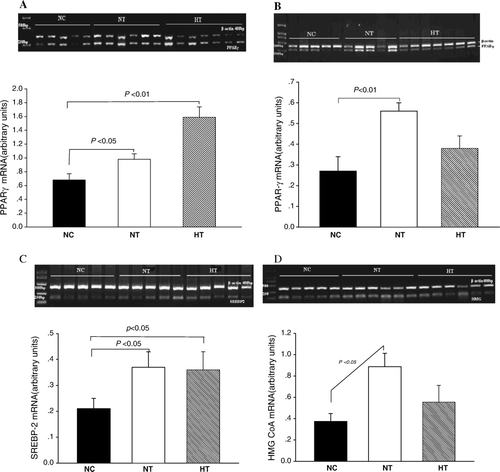
Insulin signaling studies
To explore the cellular mechanisms involved in the visfatin-induced insulin sensitivity, we obtained muscle and liver samples at the end of the euglycemic-hyperinsulinemic clamping study. These samples represented the fully ‘insulinized’ state at the termination of the glucose clamp study. Hypervisfatinemia increased IRS-1 tyrosine phosphorylation in response to insulin in the liver of the NT (1.41±0.11, P < 0.01) and the HT (1.20±0.10, P < 0.05) rats compared to the NC group (0.92±0.08). A similar increase was seen in muscles of NT (2.18±0.16, P < 0.01) and HT (1.66±0.06, P < 0.05) groups compared to NC (1.34±0.04) ().
Figure 5. Effects of visfatin/PBEF/Nampt overexpression on the extent of insulin receptor susbstrate-1 (IRS-1) tyrosine phosphorylation. A: IRS-1 tyrosine phosphorylation in the liver (n=6 in each group and each experiment was performed in triplicate); B: IRS-1 tyrosine phosphorylation in muscle tissues (n=8 in each group and each experiment was performed in triplicate). NC: normal-chow diet with pcDNA3.1(+) plasmid infusion; NT: normal-chow diet with pcDNA3.1-visfatin plasmid infusion; HT: High-fat diet with pcDNA3.1-visfatin plasmid infusion.
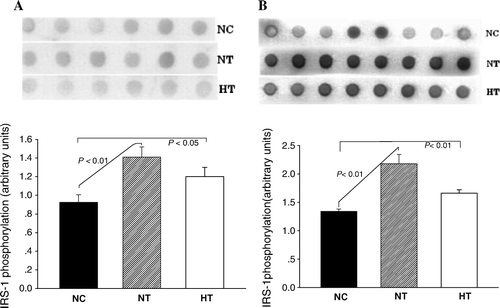
Discussion
Recent work in obesity research has revealed that adipose tissue functions as an endocrine organ, producing a variety of adipocytokines that play important roles in metabolic homeostasis Citation[20]. The newly identified adipocytokine visfatin/PBEF/Nampt has piqued interest among researchers. Assessment of the biological function of visfatin/PBEF/Nampt revealed that it has a glucose-lowering effect and enhances glucose uptake invivo and in vitro, similar to that induced by insulin. It is also reported that visfatin/PBEF/Nampt can directly activate insulin receptor (IR) in a manner distinct from insulin. In the adipocyte, visfatin/PBEF/Nampt exerts its functions through autocrine and paracrine pathways Citation[10]. These data suggest that visfatin/PBEF/Nampt is an important molecule linking adipocyte and blood glucose metabolism. To date, it has not been elucidated how visfatin/PBEF/Nampt modulates glucose and lipid metabolism. It is not known whether visfatin/PBEF/Nampt improves glucose and lipid metabolism by the activation of upstream insulin signaling components and/or by the regulation of expression of related genes. We present in this study that overexpression of visfatin/PBEF/Nampt in rats increases whole-body insulin sensitivity, probably through the activation of the insulin signaling pathway in the liver and adipose tissue. Overexpression of visfatin/PBEF/Nampt also results in the upregulation of PPARγ and SREBP-2 mRNA levels.
Visfatin/PBEF/Nampt-overexpressing rats were generated by intramuscular injection of a plasmid vector (pcDNA3.1-visfatin) containing a mouse visfatin/PBEF/Nampt cDNA. A significant increase in the plasma levels of visfatin/PBEF/Nampt protein was detected on postinjection day 4 in rats injected with pcDNA3.1-visfatin, but not observed in rats injected with a control plasmid vector pcDNA3.1(+). The expression of visfatin via plasmid pcDNA3.1-visfatin injection provided a good in vivo model for generating hypervisfatinemia and for the study of physiologic functions of visfatin/PBEF/Nampt.
We found that transgenic expression of visfatin/PBEF/Nampt led to decreased plasma levels of TC, HDL-C, and LDL-C in high-fat-fed rats. These findings suggest that visfatin/PBEF/Nampt plays an important role in cholesterol metabolism in vivo. In parallel, overexpression of visfatin/PBEF/Nampt also resulted in a significant improvement of insulin sensitivity as accessed by euglycemic-hyperinsulinemic clamps before and after the plasmid injection. As shown in , before the plasmid injection the GIR required to maintain euglycemia was significantly lower in rats fed a high-fat diet (the HT group) than in rats fed a normal diet, indicating a high-fat-induced decrease in insulin sensitivity. Four days after the plasmid injection, a second euglycemic-hyperinsulinemic clamp was done in the same groups of rats. Injection of pcDNA3.1-visfatin increased significantly the GIR not only in the HT group but also in the NT group, indicating the improvement of insulin sensitivity. Injection of control vector pcDNA3.1(+) did not alter the insulin sensitivity in the NC group. These results demonstrate the hypoglycemic effect of visfatin/PBEF/Nampt, which is not associated with diet conditions. Further studies need to be done to evaluate the effect of visfatin on lipid metabolism under physiologic conditions.
Previous studies of the relationship between visfatin/PBEF/Nampt and insulin signaling yield controversial results. To explore the potential mechanisms underlying our observed effects of visfatin/PBEF/Nampt, we determined the activity of the upstream insulin-signaling pathway by measuring IRS-1 phosphorylation in skeletal muscle and in the liver. The levels of IRS-1 phosphorylation in the liver and muscle were increased in both NT and HT rats injected pcDNA3.1-visfatin, suggesting that the improvement of insulin sensitivity by visfatin/PBEF/Nampt is probably through the activation of insulin signaling at posttranscriptional stage. However, injection of pcDNA3.1-visfatin in the present study failed to alter the plasma triglyceride levels and the mRNA expression of downstream targets of insulin signaling, including PEPCK, ATGL, HSL, and SCD-1 in the liver and muscle (data not shown). These are key enzymes involved in hepatic gluconeogenesis, lipogenesis, and lipolysis Citation[21–23]. An explanation for these observations is that visfatin improves insulin sensitivity through its anti-inflammatory effect in addition to activation of the IRS-1, since insulin resistance is known to associate with the inflammatory process.
Despite its cholesterol-lowering effect, visfatin/PBEF/Nampt appears to have no effect on lipogenesis. Administration of pcDNA3.1-visfatin failed to alter the plasma levels of triglycerides and the mRNA expression of enzymes responsible for triglyceride synthesis and hydrolysis. On day 3 following pcDNA3.1-visfatin plasmid injection the plasma levels of TC, LDL-C, and HDL-C were significantly decreased, while SREBP-2 and HMG CoA mRNA expression was significantly increased in normal-chow-fed rats. In HT rats injected with the pcDNA3.1-visfatin plasmid, only SREBP-2 mRNA expression was increased. SREBP-2 is best known to regulate HMG CoA reductase, LDL receptor, and genes directly involved in cholesterol homeostasis Citation[24], Citation[25]. These results suggest that the increase in mRNA expressions of SREBP-2 and HMG CoA may be a secondary effect of hypervisfatinemia in normal-chow rats, probably due to diminished plasma cholesterol levels. However, pcDNA3.1-visfatin treatment in HT rats did not significantly increase HMG CoA mRNA expression, suggesting a suppressive effect of excess cholesterol uptake in high-fat diet rats. Taken together, visfatin/PBEF/Nampt may exert a negative feedback through HMG CoA action in regulation of cholesterol homeostasis.
PPARγ is expressed primarily in adipose tissue and is an adipogenic factor that regulates the expression of genes associated with lipid metabolism Citation[26], Citation[27]. It is well known that PPARγ activation in adipose tissue is associated with an increase in the number of differentiated adipocytes with enhanced capacity to take up and store lipids leading to improved insulin sensitivity in other tissues Citation[28], Citation[29]. In our study, it was noteworthy that in normal-chow-fed rats with pcDNA3.1-visfatin injection, hypervisfatinemia increased PPARγ mRNA expression in the liver and adipose tissues, increased whole-body insulin sensitivity, and decreased circulating cholesterol levels. Therefore, it is possible that visfatin/PBEF/Nampt reduces insulin sensitivity and lipid profile by modifying PPARγ activities. However, PPARγ mRNA expression in adipose tissues was not significantly increased in the HT-fed pcDNA3.1-visfatin animals compared with normal controls. We speculate that the increasing insulin resistance in adipose tissues secondary to excess fat accumulation in the HF rats may lead to a downregulation of PPARγ mRNA.
To further explore the effects of elevated circulating visfatin/PBEF/Nampt levels on other adipokines, we measured the FGF-21 mRNA expression in the liver and its plasma protein levels. Both hepatic mRNA expression and plasma protein levels of FGF-21 were significantly reduced in pcDNA3.1-visfatin-injected NT but not HT rats. FGF-21 is a recently identified adipokine associated with energy homeostasis and insulin sensitivity Citation[30], Citation[31]. Our findings suggest a negative effect of visfatin/PBEF/Nampt on FGF-21 mRNA, and protein expressions may attribute to the visfatin-induced insulin sensitivity. In contrast, in HT rats, increasing insulin resistance may lead to a secondary downregulating of FGF-21 mRNA and protein expression.
In conclusion, we studied the effect of visfatin on insulin sensitivity using euglycemic-hyperinsulinemic clamps in the same group of hypervisfatinemic rats we created. We show for the first time in vivo that hypervisfatinemia leads to enhanced whole-body insulin sensitivity. Hypervisfatinemia also leads to reduced plasma concentrations of cholesterol and increased PPARγ mRNA expression in the liver and adipose tissue. Our study presents strong evidence suggesting that visfatin/PBEF/Nampt improves insulin sensitivity and has hypocholesterolemic effects, at least partially through upregulation of PPARγ activity. We speculate that visfatin/PBEF/Nampt may be a potential therapeutic target for the treatment of insulin resistance and atherosclerosis.
Acknowledgements
This work was supported by research grants from the National Natural Science Foundation of China (30771037, 30871199), Chongqing Municipal Education Commission (JK 050304), and Chongqing Medical University (XBZD200704).
Qin Sun and Ling Li contributed equally to this project.
Declaration of interest: The authors report no conflicts of interest. The authors alone are responsible for the content and writing of the paper.
References
- Kahn CR. Insulin action, diabetogenes, and the cause of type II diabetes. Diabetes. 1994; 43: 1066–84
- Polonsky KS, Sturis J, Bell GI. Non-insulin-dependent diabetes mellitus––a genetically programmed failure of the beta cell to compensate for insulin resistance. N Engl J Med. 1996; 334: 777–83
- Taylor SI. Insulin resistance or insulin deficiency: which is the primary cause of NIDDM?. Diabetes. 1994; 43: 735–40
- Staiger H, Haring HU. Adipocytokines: fat-derived humoral mediators of metabolic homeostasis. Exp Clin Endocrinol Diab. 2005; 113: 67–79
- Zhang Y, Proenca R, Maffei M, Barone M, Leopold L, Friedmen JM. Positional cloning of the mouse obese gene and its human homologue. Nature. 1994; 372: 425–32
- Steppen CM, Bailey ST, Bhat S, Brown EJ, Banerjee RR, Wright CM, et al. The hormone resistin links obesity to diabetes. Nature. 2001; 409: 307–12
- Yamauchi T, Kamon J, Waki H, Terauchi Y, Kubota N, Hara K, et al. The fat-derived hormone adiponectin reverses insulin resistance associated with both lipoatrophy and obesity. Nature Med. 2001; 7: 941–6
- Hug C, Lodish HF. Visfatin: A new adipokine. Science. 2005; 307: 366–7
- Boucher J, Masri B, Daviaud D, Gesta S, Guigne C, Mazzucotelli A, et al. Apelin, a newly identified adipokine up-regulated by insulin and obesity. Endocrinology. 2005; 146: 1764–71
- Fukuhara A, Matsuda M, Nishizawa M, Segawa K, Tanaka M, Kishimoto K, et al. Visfatin: a protein secreted by visceral fat that mimics the effects of insulin. Science. 2005; 307: 426–30
- Samal B, Sun Y, Stearns G, Xie C, Suggs S, McNiece I. Cloning and characterization of the cDNA encoding a novel human pre-B-cell colony-enhancing factor. Mol Cell Biol. 1994; 14: 1431–7
- Fukuhara, A, Matsuda, M, Nishizawa, M, Segawa, K, Tanaka, M, Kishimoto, K, , et al. Science. 2007;318:565. Retraction of: Fukuhara A, Matsuda M, Nishizawa M, Segawa K, Tanaka M, Kishimoto K, et al. Science. 2005;307:426–30.
- Beltowski J. Apelin and visfatin: Unique ‘beneficial’ adipokines upregulated in obesity?. Med Sci Monit. 2006; 12: RA112–9
- Berndt J, Kloting N, Kralisch S. Plasma visfatin concentrations and fat depot-specific mRNA expression in humans. Diabetes. 2005; 54: 2911–16
- Hammarstedt A, Pihlajamaki J, Sopasakis VR, Gogg S, Jansson PA, Laakso M, et al. Visfatin is an adipokine but it is not regulated by thiazolidinediones. J Clin Endocrinol Metab. 2006; 91: 1181–4
- Chen MP, Chung FM, Chang DM, Tsai JR, Huang HF, Shin SJ, et al. Elevated plasma level of visfatin/pre-B cell colony-enhancing factor in patients with type 2 diabetes mellitus. J Clin Endocrinol Metab. 2006; 91: 295–9
- Pagano C, Pilon C, Olivieri M, Mason P, Fabris R, Serra R, et al. Reduced plasma visfatin/pre-B cell colony- enhancing factor in obesity is not related to insulin resistance in humans. J Clin Endocrinol Metab. 2006; 91: 3165–70
- Oki K, Yamane K, Kamei N, Nojima H, Kohno N. Circulating visfatin level is correlated with inflammation, but not with insulin resistance. Clin Endocrinol (Oxf) 2007; 67: 796–800
- Yang G, Li L, Tang Y, Boden G. Short-term pioglitazone treatment prevents free fatty acid-induced hepatic insulin resistance in normal rats: Possible role of the resistin and adiponectin. Biochem Biophys Res Commun. 2006; 339: 1190–6
- Hua Z, Xi Y, Naili W, Yaou Z, Guoping C. Macrostemonoside A promotes visfatin expression in 3T3-L1 cells. Biol Pharm Bull. 2007; 30: 279–83
- Scribner KB, McGrane MM. RNA Polymerase II association with the phosphoenolpyruvate carboxykinase (PEPCK) promoter is reduced in vitamin A-deficient mice. J Nutr. 2003; 33: 4112–7
- Miyazaki M, Kim YC, Ntambi JM. A lipogenic diet in mice with a disruption of the stearoyl-CoA desaturase 1 gene reveals a stringent requirement of endogenous monounsaturated fatty acids for triglyceride synthesis. J Lipid Res. 2001; 42: 1018–24
- Schweiger M, Schreiber R, Haemmerle G, Lass A, Fledelius C, Jacobsen P, et al. Adipose triglyceride lipase and hormone-sensitive lipase are the major enzymes in adipose tissue triacylglycerol catabolism. J Bio Chem. 2006; 286: 40236–41
- Shin DJ, Osborne TF. Thyroid hormone regulation and cholesterol metabolism are connected through sterol regulatory element-binding protein-2 (SREBP-2). J Bio Chem. 2003; 278: 34114–8
- Zeng L, Liao H, Liu Y, Lee TS, Zhu M, Wang X, et al. Sterol-responsive element-binding protein (SREBP) 2 down-regulates ATP-binding cassette transporter A1 in vascular endothelial cells. J Bio Chem. 2004; 279: 48801–7
- Wahli W, Braissant O, Desvergne B. Peroxisome proliferators activated receptors: transcriptional regulation of adipogenesis, lipid metabolism and more. Chem Biol. 1995; 2: 261–6
- Schoonjans K, Peinado Onsurbe J, Lefebvre AM, Heyman RA, Briggs M, Deeb S, et al. PPARalpha and PPARgamma activators direct a distinct tissue-specific transcriptional response via a PPRE in the lipoprotein lipase gene. EMBO J. 1996; 15: 5336–48
- Yamauchi T, Kamon J, Waki H, Murakami K, Motojima K, Komeda K, et al. The mechanisms by which both heterozygous peroxisome proliferator-activated receptor γ (PPARγ) deficiency and PPARγ agonist improve insulin resistance. J Biol Chem. 2001; 276: 41245–54
- Berthiaume M, Sell H, Lalonde J, Gelinas Y, Tchernof A, Richard D, et al. Actions of PPARγ agonism on adipose tissue remodeling, insulin sensitivity, and lipemia in absence of glucocorticoids. Am J Physiol Regul Integr Comp Physiol. 2004; 287: R1116–23
- Kharitonenkov A, Shiyanova TL, Koester A, Ford AM, Micanovic R, Galbreath EJ, et al. FGF-21 as a novel metabolic regulator. J Clin Invest. 2005; 115: 1627–35
- Wente W, Efanov AM, Brenner M, Kharitonenkov M, Köster A, Sandusky GE, et al. Fibroblast growth factor-21 improves pancreatic ß-cell function and survival by activation of extracellular signal-regulated kinase 1/2 and Akt signaling pathways. Diabetes. 2006; 55: 2470–8