Abstract
Background
Hypoxia-reperfusion (HR) and inflammation are causes of renal allograft injury. Pathological evidence has indicated that ischemia followed by reperfusion leads to the proteolysis and destruction of the extracellular matrix (ECM) in renal tubular epithelial cells. Matrix metalloproteinases (MMPs), such as MMP-2 and MMP-9, play roles in cleaving and reshaping the ECM. Acute accumulation of MMP-9 secreted from neutrophils promotes the incidence of inflammation and exacerbates graft trauma. Our goal was to investigate the activities of MMP-9/MMP-2 and their correlation with HR injury and neutrophil-related inflammation in renal proximal tubular cells.
Methods
This model was established by placing HK-2 cells under hypoxic conditions (5% CO2, 1% O2) for 6 h and then exposing them to reperfusion (5% CO2, 21% O2) for 12 h in a tri-gas incubator. The cell culture medium was collected for culturing polymorphonuclear leukocytes (PMNs). BB-94 (MMP-9 inhibitor) was added to the culture medium in the inhibitor group.
Results
Flow cytometry showed a significant increase in reactive oxygen species (ROS) levels in HK-2 cells from the HR injury group. MMP-9 expression was significantly increased and MMP-2 expression was significantly decreased in HK-2 cells from the HR group. MMP-9 and MPO expression were significantly increased in the HR group, while MPO expression was significantly decreased in the PMN inhibitor group.
Conclusions
The outcomes indicated that MMP-9 and MMP-2 are important components of an underlying pathophysiological mechanism of injury following HR. MMP-9 inhibition may be a potential approach to mitigateHR injury.
Introduction
Due to the current shortage of organs for transplantation, the use of marginal kidneys has increased, and the incidence of delayed graft function (DGF) has gradually increased [Citation1]. Acute kidney injury is a risk factor for DGF [Citation2], and evidence has shown that hypoxia-reperfusion injury (HRI) plays an important role in this process [Citation3]. HRI occurs when organs experience a restriction of blood supply followed by a subsequent restoration of perfusion and concomitant reoxygenation [Citation4]. According to a previous study, oxidative stress caused by reactive oxygen species (ROS) is a potential mechanism of HRI [Citation5].
Bonventre [Citation6] first discovered that renal tubular cells undergo proteolysis during HRI. Regarding the mechanism underlying this phenomenon, several researchers have speculated that matrix metalloproteinases (MMPs) participate in remodeling the proximal and distal tubules [Citation7]. MMPs, including MMP-9 and MMP-2, are a family of zinc-dependent endopeptidases that degrade various proteins in the extracellular matrix (ECM) [Citation8]. MMP-9 regulates ECM remodeling by degrading many ECM proteins [Citation9,Citation10]. MMPs are secreted by many cells, including vascular smooth muscle (VSM) cells, fibroblasts, and polymorphonuclear leukocytes (PMNs). Some experimental results have indicated that MMPs are expressed at significantly different levels in rats with HRI [Citation11]. In addition, some clinical data suggest that the MMP-9 concentration in the early post-transplant period is a potential marker to evaluate the early and long-term function of transplanted kidneys [Citation12]. However, some conclusions drawn from animal experiments and clinical trials are contradictory, and to date, a consensus has not been established regarding the expression levels of MMPs during the HRI process. In addition, few studies have established a physical HRI model in vitro.
PMNs participate in inflammation during blood perfusion and secrete MMP-9 to digest the ECM and facilitate cell adhesion and chemotaxis. PMNs and platelets combine to form thrombi in the microcirculation after HRI [Citation13], which are not conducive to the recovery of graft function after kidney transplantation. Evidence indicates that PMNs are associated with the acute accumulation of MMP-9, which promotes the incidence of inflammation and exacerbates graft trauma after liver and lung transplantation [Citation14]. According to Christoffersson et al. [Citation15], the CD11b+/Gr-1+/CXCR4hi leukocyte subset is efficiently recruited from the circulation to hypoxic sites and delivers considerable amounts of MMP-9, which was detected during the revascularization of transplanted pancreatic islets. However, to date, no similar studies have focused on kidney transplantation.
Recently, we identified a reliable in vitro physical model of HR injury that was established with a tri-gas incubator [Citation16]. The tri-gas cell incubator is a device that uses ultrasonic sensors, electrochemical sensors, and conductor heat-sensitive integrated temperature sensors. It accurately detects the gas concentrations and temperatures of N2, CO2, and O2. Another function of this device is to adjust the gas concentration and temperature of each component in the box; thus, it is often used to establish a cell culture model with low oxygen levels. This in vitro model was first used by Sauvant et al. [Citation17,Citation18] to simulate the process of HR injury in a rat proximal tubular cell line. Cavdar et al. [Citation19] also used a similar model of human umbilical vein endothelial cells that was established with a hypoxia system that consisted of a hypoxic chamber and a gas-mixture inlet. In this study, our model is based on the system described in the study by Weng et al. [Citation16], which used a tri-gas incubator. The device used to establish the model is much more developed and reliable for effectively simulating and monitoring the process of HR.
Thus, in this study, our aims were to investigate the activities of MMP-9 and MMP-2 and their correlations with HRI, as well as to investigate the correlation between the activities of MMP-9 with PMN-related inflammation during the HR process.
Materials and methods
Cell culture
HK-2 cells (a human renal epithelial cell line with characteristics of proximal tubular cells) were purchased from the American Type Culture Collection, catalog number CRL-2190. HK-2 cells were cultured in RPMI 1640 medium (Gibco, Gaithersburg, MD) supplemented with 10% fetal calf serum (Biological Industries, Beit-Haemek, Israel), penicillin (100 U/mL), and streptomycin (100 µg/mL). The cells were cultured in 6-well plates in an atmosphere containing 5% CO2 and 21% O2 and maintained at pH 7.4 and 37 °C for 24 h prior to the experiments, as appropriate.
Isolation of polymorphonuclear neutrophils (PMNs)
Human PMNs were obtained from the peripheral blood of healthy laboratory members who volunteered to provide blood samples. The blood samples (10 mL each) were treated with the anticoagulant heparin sodium. PMNs were isolated using the Ficoll–Dextran method [Citation20] (P0940 kit, Solarbio Life Science, Beijing, China) and resuspended in RPMI 1640 medium.
In vitro model
Cells in culture dishes were randomly divided into three groups. Cells in the control group were cultured in complete medium under normal conditions. Cells in the hypoxia-reperfusion group (HR group) were cultured with serum-free medium under ischemic conditions (5% CO2, 1% O2) for 6 h in a tri-gas incubator and then cultured with complete medium for reperfusion (5% CO2, 21% O2) for 12 h in a normal incubator. In the MMP-9 inhibitor group, the MMP-9 inhibitor BB-94 (4 nmol; Selleck, Houston, TX) was added to the serum-free culture medium of HK-2 cells during the hypoxic phase, and then this medium was discarded and replaced with complete medium in the HK-2 cell reperfusion phase. The culture supernatants (without the MMP-9 inhibitor BB-94) were collected and centrifuged to remove the sediment for the next step of PMN culture.
PMN culture
The isolated PMNs were randomly divided into three groups, resuspended in HK-2 culture supernatant (control group, HR group, and MMP-9 inhibitor group), and incubated for 12 h. PMN activation was analyzed by detecting CD11b and CD18 expression using RT-PCR.
Cell viability
Cell Counting Kit-8 (Dojindo Laboratories, Kumamoto, Japan) was used to measure cell viability. HK-2 cells were grown in 96-well plates. The cells were washed twice with PBS and incubated for 1 h at 37 °C with 5 mg/mL of the CCK-8 stock solution in RPMI 1640 medium. The absorption was measured at 562 nm, and the viability was reported as the % absorbance (Multiskan FC, Thermo, Waltham, MA).
Analysis of ROS using immunofluorescence microscopy and flow cytometry
HK-2 cells were washed twice with 1 mL of PBS per well for 1 min. Then, HK-2 cells were cultured in RPMI 1640 medium containing a ROS-specific fluorescent probe (5 µmol, H2DCFDA, Abmole Bioscience, Houston, TX) in the dark for 30 min. HK-2 cells were washed twice with 1 mL of PBS and then cultured in RPMI 1640 medium as appropriate for immunofluorescence staining and microscopy. Images were captured with a digital inverted microscope (EVOS FL AMF-4302-AU, AMG, WA, USA) under a green excitation laser. Then, the cells were digested with 0.25% trypsin (Gibco, Gaithersburg, MD). The cells were resuspended in 1 mL of PBS per well as appropriate. Flow cytometry (CytoFLEX, Beckman Coulter, Brea, CA) was performed to analyze ROS levels with an excitation wavelength of 504 nm and an emission wavelength of 529 nm. The images were then processed and overlaid using Adobe Photoshop version 7.0 software (Adobe Systems, Mountain View, CA). Images were analyzed using ImageJ software (National Institutes of Health, Bethesda, MD).
RNA extraction and reverse transcription
Total RNA was extracted from HK-2 cells and PMNs with TRIzol (Solarbio Life Science, Beijing, China). Reverse transcription was performed using 5 µg of the total RNA in a first-strand cDNA synthesis reaction with SuperScript II RNaseH Reverse Transcriptase (AG Scientific, San Diego, CA). The RT-PCR conditions were 95 °C for 30 s, followed by 39 cycles of 95 °C for 3 s, 55 °C for 30 s, and 95 °C for 30 s. The cDNA products were amplified by RT-PCR using primers specific for MMP-9/2, TIMP-1, CD-11b/CD-18, and β-actin. All RT-PCR data were normalized to β-actin. The specific primers used in this study are listed in .
Table 1. The primer sequences used for RT-PCR.
Western blot analysis of MMP-2 and MMP-9
Levels of the MMP-2 and MMP-9 proteins were examined using Western blotting. Briefly, proteins were extracted from HK-2 cells and PMNs, separated on 10% SDS-PAGE gels, and transferred onto nitrocellulose membranes (Millipore, Burlington, MA). The membranes were blocked with 5% nonfat milk in a Tris-buffered saline solution (Solarbio Life Science, Beijing, China) and incubated with the following rabbit primary antibodies: MMP-9 (1:1,000; Affinity Biosciences, Zhenjiang, China) and MMP-2 (1:1,000; Affinity Biosciences, Zhenjiang, China). The membranes were then incubated with secondary horseradish peroxidase-conjugated goat anti-rabbit IgG antibodies (1:3,000; ZSGB-BIO, Wuhan, China). The specific bands were developed and visualized using an enhanced chemiluminescence detection kit (Solarbio Life Science, Beijing, China). Images were analyzed using ImageJ software.
Determination of MPO enzyme activity
The MPO activity in PMNs was determined with an MPO Detection Kit (Nanjing Jiancheng Bioengineering Institute, Nanjing, China). The MPO proteins were extracted from the PMNs, and 10 μL of the supernatant was added to PBS (pH 6.0) containing 0.17 mg/mL 3,3′-dimethoxybenzidine and 0.0005% H2O2. The H2O2-dependent oxidation of 3,3′-dimethoxybenzidine was used to characterize the activity of MPO, which was reported as units per gram of total protein (u/g) [Citation21]. The total protein content in the samples was analyzed using a bicinchoninic acid protein assay kit.
Statistical analysis
All experiments were repeated in triplicate. The data are presented as the means ± SD Student’s t-test was used to calculate statistical significance. p < .05 was considered to indicate a statistically significant difference. All statistical tests were performed using SPSS version 22.0 (IBM, Armonk, NY).
Results
Viability of HK-2 cells following HR treatment and the BB-94 intervention
The viability of HK-2 cells was assessed after the HR treatment and BB-94 intervention using the CCK-8 assay to detect changes in biological activities. HK-2 cells were treated with CCK-8 reagents after culture and BB-94 intervention. The viability of the HK-2 cells in the HR group was significantly decreased compared with that of the HK-2 cells in the control group (< .05). Furthermore, no difference was observed between the HR group and the inhibitor group.
ROS levels in HK-2 cells following HR treatment
HK-2 cells were subjected to serum starvation and treated in accordance with the group assignments described above. After 4 h of culture, HK-2 cells were collected, stained with a ROS-specific fluorescent probe, and immunofluorescence was analyzed. As shown in , the relative fluorescence intensity in the control group was significantly lower than that in the HR and inhibitor groups (p < .005). Compared with the HR group, the relative fluorescence intensity in the inhibitor group was significantly lower (p < .01) (). As shown in , the flow cytometry analyses revealed a significant increase in the mean fluorescence intensity (MFI) of the ROS in the HR group compared with that in the control group (p < .005). In addition, a difference between the HR and inhibitor groups (p < .01) was observed ().
Figure 2. Relative fluorescence intensity and ratio of ROS + HK-2 cells. (A) Fluorescence images of HK-2 cells captured using a fluorescence microscope. (B) Quantitative analysis of the relative fluorescence intensity. (C) Flow cytometry plots of HK-2 cells. (D) Quantitative flow cytometry data. All experiments were repeated three times, and the mean values are presented and compared (**p < .01 and ***p < .005).
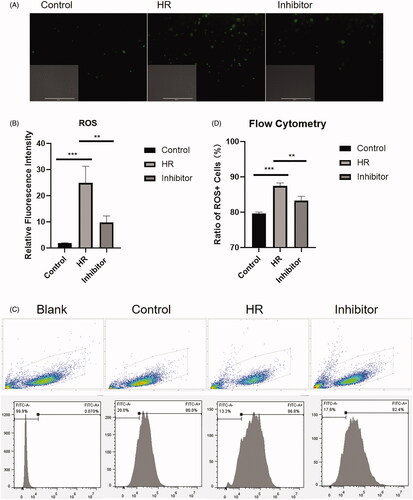
Levels of the MMP-2, MMP-9, and TIMP-1 mRNAs in HK-2 cells
RT-PCR experiments were performed to measure the expression levels of MMP-2, MMP-9, and TIMP-1 in HK-2 cells. MMP-2 mRNA expression was significantly downregulated in the HR group compared with the control group (p < .0001) (). In contrast to the MMP-2 mRNA expression pattern, MMP-9 mRNA expression was significantly upregulated in the HR group compared with the control group (p < .05) (). The mRNA encoding TIMP-1, a natural inhibitor of MMPs, was upregulated in the HR group (p < .005) ().
Figure 3. Levels of the MMP-2, MMP-9, and TIMP-1 mRNAs in HK-2 cells. (A) Real-time PCR analysis of levels of the MMP-2 mRNA in HK-2 cells. (B) Levels of the MMP-9 mRNA in HK-2 cells. (C) Levels of the TIMP-1 mRNA in HK-2 cells. All experiments were repeated three times, and the mean values are presented and compared (*p < .05; **p < .005; and ****p < .001).
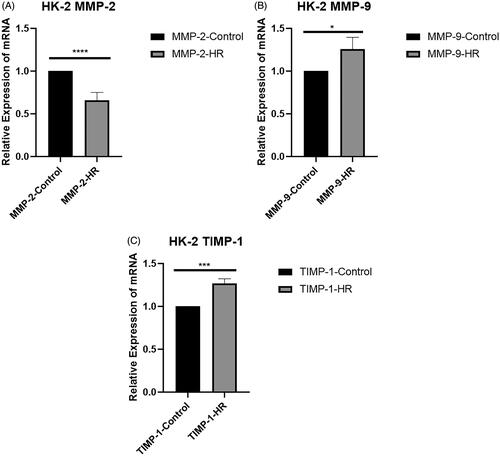
Levels of the MMP-9 and MMP-2 proteins in HK-2 cells exposed to HR
Levels of the MMP-9 and MMP-2 proteins were examined using Western blotting (). The expression of the MMP-9 protein was upregulated in the HR group compared with the control and inhibitor groups (p < .05) (), while the expression of the MMP-2 protein was downregulated in the HR group (p < .01) ().
Figure 4. Levels of the MMP-9 and MMP-2 proteins in HK-2 cells. (A) Western blot analysis of MMP-2 protein levels. (B) Quantitation of the Western blot analysis of MMP-2 protein levels. (C) Western blot analysis of MMP-9 protein levels. (D) Quantitation of the Western blot analysis of MMP-9 protein levels. All experiments were repeated three times, and the mean values are presented and compared (*p < .05).
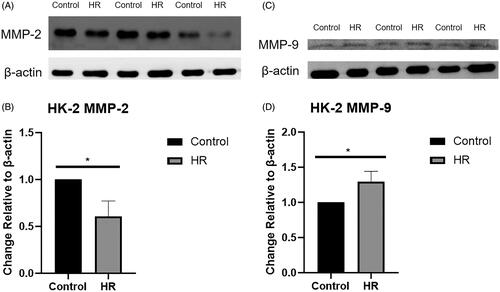
Levels of the CD-11b, CD-18, and MMP-9 mRNAs in PMNs
An RT-PCR experiment was also performed to measure the expression levels of CD-11b, CD-18, and MMP-9 in PMNs. CD11b and CD18 are typically analyzed to determine the degree of PMN activation. CD11b mRNA expression was upregulated in the HR group compared with the control group (p < .05) (). The expression levels of the CD-18 mRNA were significantly upregulated in the HR group compared with the control group (p < .05) (). Similar to CD-11b and CD-18, MMP-9 expression was significantly upregulated in the HR group (p < .01) ().
Figure 5. Levels of the CD-11b, CD-18, and MMP-9 mRNAs in PMNs. (A) Real-time PCR analysis of levels of the CD-11b mRNA in PMNs. (B) Levels of the CD-18 mRNA in PMNs. (C) Levels of the MMP-9 mRNA in PMNs. All experiments were repeated three times, and the mean values are presented and compared (*p < .05 and **p < .01).
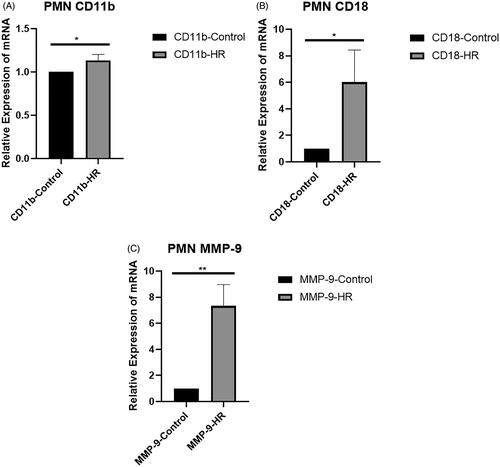
Level of the MMP-9 protein in PMNs treated with HK-2 cell supernatants
Levels of the MMP-9 protein in PMNs were examined using Western blotting (). The expression of the MMP-9 protein was upregulated in the HR group compared with the control and inhibitor groups (p < .001) ().
Detection of MPO enzyme activity in PMNs
PMNs were analyzed with the MPO Detection Kit. After treatment, the MPO enzyme activity was observed with an enzyme labeling instrument (). The MPO enzyme activity in the HR group was higher than that in the control group (p < .001). Compared with the HR group, the MPO enzyme activity in the inhibitor group was significantly reduced (p < .001), suggesting that BB-94 limited the injury caused by HR.
Discussion
Most previous studies have established in vivo HR models in animals, but the results are contradictory. We speculated that tissue samples always contain multiple types of cells, including epithelial cells, mesenchymal cells, and inflammatory cells. However, necrosis of renal epithelial cells is the main cause of acute kidney injury after renal transplantation. The in vitro model adequately circumvents these problems. Thus, in this study, we aimed to specifically examine expression patterns in epithelial cells. Due to the advantages of cell models, in which cell types can be identified, we decided to use an in vitro model in this experimental design, in which two cell types were cultured separately.
Although a few studies have established in vitro HR models, these studies mostly used chemical processes, which may affect the physiological conditions of the models. A few studies used a mineral oil overlay to simulate the ischemic environment; however, the accuracy of this simulation was not sufficient [Citation22,Citation23]. Thus, in our study, we established an in vitro HR model with a tri-gas incubator that generated conditions as close to the pathophysiological environment as possible.
In clinical practice, inhibitors can be added to the perfusion solution to protect the kidney during cold ischemia (the drug intervention is only administered to the donor kidney, not the recipient, to avoid a greater burden on the recipient). The accumulation of PMNs after HRI is not conducive to the functional recovery of the graft [Citation13]. Thus, our purpose is to establish a model of the effect of donor kidney tissue undergoing HR during kidney transplantation on neutrophils in the recipient. In our model, the inhibitor BB-94 was only added to the cell culture medium during the HR period. Afterwards, the cell supernatant was discarded and replaced with complete medium (without the MMP-9 inhibitor BB-94) in the HK-2 cell reperfusion phase, which simulates the microenvironment of PMN-related inflammation during reperfusion in the recipient. The model was capable of simulating the inflammatory response in the kidney undergoing HRI to the recipient’s neutrophils.
The isolation of PMNs and HK-2 cells allowed us to detect the expression patterns in each cell type. The tri-gas incubator provided a controlled physical environment that stimulated HR. In this study, we investigated cell viability to evaluate the feasibility of establishing the model () and used an ROS-specific fluorescent probe to evaluate HR injury (). As shown in , HR decreased cell viability, and no difference was observed between the HR group and inhibitor group, suggesting that the inhibitor dose was appropriate. shows that HR increased ROS-mediated injury, and an MMP inhibitor (BB-94) reduced HR damage. According to the results, we showed the feasibility of the model and intervention conditions in this experiment.
Regarding the phenomenon of proteolysis during HRI, several studies have suggested that MMPs are associated with proteolysis. However, a consensus has not been reached on the conclusions drawn from experiments examining the expression of MMPs. Based on the findings obtained from an in vivo rat model, MMP-2 and MMP-9 are upregulated in response to HRI [Citation24,Citation25], and tissue inhibitor of metalloproteinases-1 (TIMP-1), a naturally occurring protein, was downregulated [Citation26]. However, several clinical studies have suggested that the poor prognoses of some patients are related to MMP-9 and TIMP-1 upregulation and MMP-2 downregulation [Citation27–29]. Racca et al. [Citation28] suggested that among kidney transplant patients, patients with interstitial fibrosis and tubular atrophy (IF/TA) have higher plasma MMP-9 levels. According to the results of the study by Palomar et al. [Citation27], MMP-2 expression was lower in the chronic allograft nephropathy (CAN) patient group, and the changes were attributed to early transplant biopsies. Mazanowska et al. [Citation29] suggested that proteinuria and estimated daily proteinuria (uPr:uCr) were positively correlated with plasma TIMP-1 levels in kidney transplant recipients. In our study, the results obtained from HK-2 cells were similar to clinical evidence and indicated that MMP-9 and TIMP-1 were upregulated and MMP-2 was downregulated ( and ). We postulated that the results indirectly confirm the reliability of the clinical evidence mentioned above. Our result is similar to the findings reported by Covington MD et al. [Citation30], which suggested lower expression of the MMP-2 protein in mineral oil overlay models using NRK cells.
Although the evidence indicates that PMNs are associated with the acute accumulation of MMP-9, which promotes the incidence of inflammation and exacerbates graft trauma after liver and lung transplantation [Citation31,Citation32], few studies have focused on kidney transplantation. In our model, isolated PMNs were resuspended in HK-2 cell culture supernatants, allowing HK-2 cells and PMNs to be separated. This approach ensures that the expression patterns of each cell type would be determined without interference. The expression levels of the CD-11b and CD-18 mRNAs were significantly upregulated in the HR group (). We considered that HR-treated HK-2 cell culture supernatants increased the inflammatory activity of PMNs. As shown in , the expression level of MMP-9 was increased in the HR group, and shows increased MPO enzyme activity in the HR group. MPO is a marker commonly used to assess the inflammatory activities of PMNs. The data presented in both and support our hypothesis that HR-treated proximal tubular cells may also exert the same MMP-9-mediated inflammatory effects as those observed after liver and lung transplantation. Additionally, the MPO enzyme activity in the inhibitor group was significantly reduced (), suggesting that the inhibition of MMP-9 is a potential mechanism to reduce inflammatory injury after ischemia and reperfusion during kidney transplantation.
Some limitations of our study should be considered. The application of gene knockout technology may validate our findings on the role of MMP. However, our experimental design is based on clinical treatment, and pharmacological inhibitors have established applications. If we study the molecular mechanism in the future, we will consider designing a gene knockout model. We know that if the aforementioned techniques are adopted, the role of MMPs in this process can be clarified. Another limitation of our study is that ECM is difficult to characterize using standard methods. In view of the close relationship between the ECM and MMPs, we indirectly indicate changes in the ECM based on changes in MMPs. The purpose of the experiment is not to reveal the molecular mechanism, although the authors will explore it in future experiments, if possible.
In summary, HR exacerbated the oxidative stress caused by ROS, and MMP-9 regulated HR-related neutrophil inflammation in proximal tubular cells. Further research, including the histopathological and immunological examinations of effective animal models, is warranted to verify our conclusion.
Author contributions
Y.D. conceived and designed the experiments. Y.D. and H.Z. performed the experiments. H.Z. jointly analyzed the results. Y.D. and H.Z. drafted the manuscript. L.Y., J.M., and S.F. performed critical revisions. L.Y supervised and coordinated all the work.
Abbreviations | ||
HR | = | hypoxia-reperfusion |
ECM | = | extracellular matrix |
MMPs | = | matrix metalloproteinases |
PMNs | = | polymorphonuclear leukocytes |
DGF | = | delayed graft function |
HRI | = | hypoxia-reperfusion injury |
ROS | = | reactive oxygen species |
VSM | = | vascular smooth muscle |
MFI | = | mean fluorescence intensity |
TIMP-1 | = | tissue inhibitor of metalloproteinases-1 |
IF/TA | = | interstitial fibrosis and tubular atrophy |
CAN | = | chronic allograft nephropathy |
Supplemental Material
Download PDF (78.7 KB)Supplemental Material
Download PDF (132.8 KB)Acknowledgments
The authors thank the Gansu Nephro-Urological Clinical Center and Key Laboratory of Urological Disease of Gansu Province.
Disclosure statement
No potential conflicts of interest are reported by the author(s).
Data availability statement
The data used to support the findings of this study are included in the article.
Additional information
Funding
References
- Zheng J, Hu X, Ding, X et al. Comprehensive assessment of deceased donor kidneys with clinical characteristics, pre-implant biopsy histopathology and hypothermic mechanical perfusion parameters is highly predictive of delayed graft function. Ren Fail. 2020;42(1):369–376.
- Domagala P, Gorski L, Wszola M, et al. Successful transplantation of kidneys from deceased donors with terminal acute kidney injury. Ren Fail. 2019;41(1):167–174.
- Bahl D, Haddad Z, Datoo A, et al. Delayed graft function in kidney transplantation. Curr Opin Organ Transplant. 2019;24(1):82–86.
- Salvadori M, Rosso G, Bertoni E. Update on ischemia-reperfusion injury in kidney transplantation: pathogenesis and treatment. World J Transplant. 2015;5(2):52–67.
- Cadenas S. ROS and redox signaling in myocardial ischemia-reperfusion injury and cardioprotection. Free Radic Biol Med. 2018;117:76–89.
- Bonventre JV. Mechanisms of ischemic acute renal failure. Kidney Int. 1993;43(5):1160–1178.
- Romanic AM, Burns-Kurtis CL, Ao Z, et al. Upregulated expression of human membrane type-5 matrix metalloproteinase in kidneys from diabetic patients. Am J Physiol Renal Physiol. 2001;281(2):F309–F17.
- Wang X, Khalil RA. Matrix metalloproteinases, vascular remodeling, and vascular disease. Adv Pharmacol. 2018;81:241–330.
- Vandooren J, Van den Steen PE, Opdenakker G. Biochemistry and molecular biology of gelatinase B or matrix metalloproteinase-9 (MMP-9): the next decade. Crit Rev Biochem Mol Biol. 2013;48(3):222–272.
- Reinhard SM, Razak K, Ethell IM. A delicate balance: role of MMP-9 in brain development and pathophysiology of neurodevelopmental disorders. Front Cell Neurosci. 2015;9:280.
- Chang MW, Chen CH, Chen YC, et al. Sitagliptin protects rat kidneys from acute ischemia-reperfusion injury via upregulation of GLP-1 and GLP-1 receptors. Acta Pharmacol Sin. 2015;36(1):119–130.
- Kwiatkowska E, Domanski L, Bober J, et al. Urinary metalloproteinases-9 and -2 and their inhibitors TIMP-1 and TIMP-2 are markers of early and long-term graft function after renal transplantation. Kidney Blood Press Res. 2016;41(3):288–297.
- Kavanagh DPJ, Lokman AB, Neag G, et al. Imaging the injured beating heart intravitally and the vasculoprotection afforded by haematopoietic stem cells. Cardiovasc Res. 2019;115(13):1918–1932.
- Yao W, Han X, Guan Y, et al. Neutrophil elastase inhibitors suppress oxidative stress in lung during liver transplantation. Oxid Med Cell Longev. 2019;2019(2019):7323986.
- Christoffersson G, Vagesjo E, Vandooren J, et al. VEGF-A recruits a proangiogenic MMP-9-delivering neutrophil subset that induces angiogenesis in transplanted hypoxic tissue. Blood. 2012;120(23):4653–4662.
- Weng X, Wang L, Chen H, et al. Ischemic postconditioning inhibits apoptosis in an in vitro proximal tubular cell model. Mol Med Rep. 2015;12(1):99–104.
- Sauvant C, Schneider R, Holzinger H, et al. Implementation of an in vitro model system for investigation of reperfusion damage after renal ischemia. Cell Physiol Biochem. 2009;24(5–6):567–576.
- Sauvant C, Schneider R, Holzinger H, et al. Indomethacin corrects alterations associated with ischemia/reperfusion in an in vitro proximal tubular model. Am J Nephrol. 2010;32(1):57–65.
- Cavdar Z, Oktay G, Egrilmez MY, et al. In vitro reoxygenation following hypoxia increases MMP-2 and TIMP-2 secretion by human umbilical vein endothelial cells. Acta Biochim Pol. 2010;57(1):69–73.
- Wiedermann CJ, Niedermuhlbichler M, Braunsteiner H, et al. Priming of polymorphonuclear neutrophils by atrial natriuretic peptide in vitro. J Clin Invest. 1992;89(5):1580–1586.
- Liu SH, Ma K, Xu XR, et al. A single dose of carbon monoxide intraperitoneal administration protects rat intestine from injury induced by lipopolysaccharide. Cell Stress Chaperones. 2010;15(5):717–727.
- Witzgall R. The proximal tubule phenotype and its disruption in acute renal failure and polycystic kidney disease. Exp Nephrol. 1999;7(1):15–19.
- Meldrum KK, Meldrum DR, Hile KL, et al. A novel model of ischemia in renal tubular cells which closely parallels in vivo injury. J Surg Res. 2001;99(2):288–293.
- Caron A, Desrosiers RR, Beliveau R. Ischemia injury alters endothelial cell properties of kidney cortex: stimulation of MMP-9. Exp Cell Res. 2005;310(1):105–116.
- Inkinen KA, Soots AP, Krogerus LA, et al. Fibrosis and matrix metalloproteinases in rat renal allografts. Transpl Int. 2005;18(5):506–512.
- Caron A, Desrosiers RR, Langlois S, et al. Ischemia-reperfusion injury stimulates gelatinase expression and activity in kidney glomeruli. Can J Physiol Pharmacol. 2005;83(3):287–300.
- Palomar R, Mayorga M, Ruiz JC, et al. Markers of fibrosis in early biopsies of renal transplants. Transplant Proc. 2005;37(3):1468–1470.
- Racca MA, Novoa PA, Rodriguez I, et al. Renal dysfunction and intragraft proMMP9 activity in renal transplant recipients with interstitial fibrosis and tubular atrophy. Transpl Int. 2015;28(1):71–78.
- Mazanowska O, Żabińska M, Kościelska-Kasprzak K, et al. Increased plasma matrix metalloproteinase-2 (MMP-2), tissue inhibitor of proteinase-1 (TIMP-1), TIMP-2, and urine MMP-2 concentrations correlate with proteinuria in renal transplant recipients. Transplant Proc. 2014;46(8):2636–2639.
- Covington MD, Burghardt RC, Parrish AR. Ischemia-induced cleavage of cadherins in NRK cells requires MT1-MMP (MMP-14). Am J Physiol Renal Physiol. 2006;290(1):F43–F51. DOI:10.1152/ajprenal.00179.2005.
- Uchida Y, Freitas MC, Zhao D, et al. The protective function of neutrophil elastase inhibitor in liver ischemia/reperfusion injury. Transplantation. 2010;89(9):1050–1056.
- Hardison MT, Galin FS, Calderon CE, et al. The presence of a matrix-derived neutrophil chemoattractant in bronchiolitis obliterans syndrome after lung transplantation. J Immunol. 2009;182(7):4423–4431.