Abstract
Purpose
To investigate the effects of canagliflozin (20 mg/kg) on Dahl salt-sensitive (DSS) rat gut microbiota and salt-sensitive hypertension-induced kidney injury and further explore its possible mechanism.
Methods
Rats were fed a high-salt diet to induce hypertension and kidney injury, and physical and physiological indicators were measured afterwards. This study employed 16S rRNA sequencing technology and liquid chromatography–tandem mass spectrometry (LC–MS/MS)-based metabolic profiling combined with advanced differential and association analyses to investigate the correlation between the microbiome and the metabolome in male DSS rats.
Results
A high-salt diet disrupted the balance of the intestinal flora and increased toxic metabolites (methyhistidines, creatinine, homocitrulline, and indoxyl sulfate), resulting in severe kidney damage. Canagliflozin contributed to reconstructing the intestinal flora of DSS rats by significantly increasing the abundance of Corynebacterium spp., Bifidobacterium spp., Facklamia spp., Lactobacillus spp., Ruminococcus spp., Blautia spp., Coprococcus spp., and Allobaculum spp. Moreover, the reconstruction of the intestinal microbiota led to significant changes in host amino acid metabolite concentrations. The concentration of uremic toxins, such as methyhistidines, creatinine, and homocitrulline, in the serum of rats was decreased by canagliflozin, which resulted in oxidative stress and renal injury alleviation.
Conclusion
Canagliflozin may change the production of metabolites and reduce the level of uremic toxins in the blood circulation by reconstructing the intestinal flora of DSS rats fed a high-salt diet, ultimately alleviating oxidative stress and renal injury.
Introduction
Hypertension poses a major threat to cardiovascular health, and existing data show that dietary salt intake is directly related to blood pressure (BP) and hypertension [Citation1,Citation2]. The vast majority of hypertensive patients have essential hypertension (i.e. hypertension for which there is no known medical cause); in these patients, dietary salt intake is one of the common causes of hypertension [Citation3].
A high-salt diet (HSD) significantly contributes to essential hypertension in clinical populations [Citation4]. Microbiota-related research has become an important mechanism linking diet to host health and disease. There is now much evidence to support that the gut microbiota is associated with essential hypertension [Citation5,Citation6]. Studies have shown that hypertensive patients and model animals show intestinal flora imbalance and reduced microbial diversity compared with normal BP controls [Citation7,Citation8]. Short-chain fatty acids (SCFAs), vasoactive hormones, and uremic toxins are a few of the metabolites that the bacteria in the intestines can release that may be important in the control of BP [Citation9,Citation10]. It is primarily the pro- or anti-inflammatory activity of these metabolites that have a role in managing BP [11]. The results presented above all strongly suggest that the gut microbiota has a role in hypertension.
The treatment tool currently available for preventing the progression of chronic kidney disease (CKD), which is primarily brought on by hypertension, is BP monitoring. Recent studies have mostly concentrated on lowering fibrosis and inflammation at different stages, but this has not been able to stop CKD progression, especially regarding end-stage renal disease [Citation12]. Prebiotics enhanced renal function and reduced fibrosis in a rat model of CKD by bringing the gut microbiota closer to that of control animals [Citation13]. Considering that the gut microbiota and the renal system interact, preventative measures, including modifying the microbiome inducers of uremic toxins implicated in the pathogenesis of CKD, seem promising.
A widely recognized animal model of salt-induced high BP and kidney damage, the Dahl salt-sensitive (DSS) rat model, replicates many of the signs and symptoms frequently seen among individuals with salt-sensitive hypertension, including albuminuria and severe glomerulosclerosis [Citation14,Citation15]. In DSS rats, HSD-induced hypertension and kidney damage are linked to alterations in microbial communities [Citation16]. A sodium-glucose cotransporter 2 (SGLT2) inhibitor, canagliflozin, also has a modest inhibitory impact on SGLT1 [17]. The gastrointestinal environment may change if intestinal SGLT1 is inhibited [Citation18]. Canagliflozin has effects in the intestine that prevent the buildup of uremic toxins, such as p-cresyl sulfate [Citation19]. To further examine the potential mechanism, we looked at the effects of canagliflozin on the gut microbiota and kidney damage caused by salt-sensitive hypertension in DSS rats.
Materials and methods
Animal experiment
Male DSS rats were purchased from Beijing Vital River Laboratory Animal Technology Co., Ltd. When they were seven weeks old, they were kept in a rodent facility with a 12-h/12-h light/dark cycle. Water and rat food were freely available to the rats. Following adaptation, 21 rats were split into three groups: the control group (normal salt diet [NSD] group; AIN-76A + 0.3% NaCl with irradiation), the hypertension and renal injury group (HSD group; AIN-76A + 8% NaCl with irradiation), and the HSD combined with 20 mg/kg/d canagliflozin treatment group (HSD + Cana group; n = 7). Approximately 0.5% hydroxypropyl methylcellulose was administered orally and daily for 12 weeks to DSS rats in the HSD and NSD groups. The rats’ body weight and food intake were measured. A tail sphygmomanometer (BP-2000, Visitech Systems, Inc., United States) was used to measure the BP in the tails of DSS rats. Weekly BP readings were taken, and the average of six actual readings was determined. The rats were housed in a metabolic cage (SA104, Jiangsu SANS Biological Technology Co. Ltd., China) one week before the experiment’s conclusion, and 24-h urine collection was performed. Twenty-four hours following the final intragastric injection, DSS rats were sacrificed. As soon as possible, serum and cecal contents were taken and kept at −80 °C for examination. Weighed left kidneys were immediately preserved in 4% paraformaldehyde. The experiment was authorized by the Hebei General Hospital’s Animal Ethics Committee and adhered to international laboratory animal management standards.
Serum analysis and urinalysis
Blood was drawn after 12 weeks of a HSD, with the rats in a completely fasted state. First, intraperitoneal injections of 3% sodium pentobarbital (30 mg/kg) were used to anesthetize the rats. The serum was then extracted and kept at −80 °C after a whole-blood sample was drawn from the abdominal aorta. Rats in the metabolic cage were allowed to eat and drink ad libitum, and urine was collected within 24 h. Serum creatinine levels, serum superoxide dismutase (SOD) activity, and urine protein levels were all measured using an automated biochemical analyzer (DS-261, SINNOWA Medical Science and Technology Co., Ltd., China).
Histological analysis
After DSS rats were anesthetized, the left kidney was quickly removed and weighed. A slice of the left kidney was fixed in 4% formaldehyde and embedded in paraffin. Masson trichrome staining was performed to determine the extent of fibrosis.
Ileum content microbiota analysis
Total microbial DNA was extracted from cecum contents. The V3-V4 hypervariable region of 16S rRNA genes was chosen as the PCR amplified region. The bacterial forward primer was 5′-ACTCCTACGGGAGGCAGCA-3′, and the reverse primer was 5′-TCGGACTACHVGGGTWTCTAAT-3′. High-throughput pyrosequencing of the PCR products was performed on an Illumina MiSeq platform at Shanghai Personal Biotechnology Co., Ltd. (Shanghai, China). The 16S rRNA raw sequencing data were processed using the open-source software QIIME 2 (version 2019.4) [Citation20]. DATA2 was used to obtain quality filtered, denoised, chimaera-free, and merged amplicon sequence variants (ASVs) [Citation21]. Sequences with any ambiguous bases were excluded. The taxonomic notation of ASVs was obtained from the Greengenes database [Citation22]. ASVs with richness in less than 0.001% of total reads or with zero values in more than 80% of samples were removed. Alpha-diversity metrics (Chao1 and Simpson) and beta-diversity metrics were estimated using the diversity plugin. Beta-diversity analysis was performed using an unsupervised principal coordinates analysis (PCoA) of Bray–Curtis dissimilarity to illustrate differences in microbiome composition profiles at the ASV level. Then, beta diversity metrics were subjected to pairwise permutational multivariate analysis of variance (PERMANOVA) (P values < 0.05) correction to determine whether the microbial communities differed statistically. To determine significantly enriched bacteria per group, we used functional difference analysis (linear discriminant analysis effect size [LEfSe]) analysis (Z scores > 2.0 and p < 0.05) and balanced analysis with the GNEISS plugin in QIIME2.
Targeted metabolomic analysis
Serum metabolomic pretreatment and measurement were performed according to the procedures established in our laboratory [Citation23]. Briefly, rat serum samples and extracts were added to an Eppendorf (EP) tube, and the supernatant was collected after centrifugation. The resulting supernatant was transferred to a fresh glass vial for analysis. Quality control (QC) samples were prepared by pooling all plasma samples and used for all analytical methods. Liquid chromatography–tandem mass spectrometry (LC–MS/MS) analyses were performed using an ultra-high-performance liquid chromatography (UHPLC) system (1290 Infinity LC, Agilent Technologies) with the HILIC and C18 phase column coupled to a mass spectrometer (6500 QTRAP, AB Sciex Pte. Ltd.). The raw data were analyzed using the MultiQuant or Analyst program for peak detection. The ratio of each substance’s peak area to the internal standard’s peak area was obtained, and the concentration was calculated according to the standard curve. This method can be used for the targeted quantitative determination of 650 specific metabolites, of which 275 metabolites were identified and quantified in this study. We screened out differentially abundant metabolites for subsequent analysis.
Statistical analysis
IBM SPSS 25.0 (IBM Corp., Armonk, United States) was used for the statistical analysis. Comparisons between groups were performed by one-way analysis of variance (ANOVA). p < 0.05 was considered to indicate a statistically significant difference. For sequencing data, alpha- and beta-diversity metrics were calculated using the q2-diversity plugin in QIIME2.
Results
Effects of canagliflozin on BP, oxidative stress, and renal fibrosis
We analyzed the trend of changes in BP within 12 weeks. As shown in , after 6 weeks of canagliflozin treatment, the BP of the HSD + Cana group was lower than that of the HSD group, but the difference was not significant. However, after 12 weeks of canagliflozin treatment, systolic and diastolic BP in the HSD + Cana group was significantly lower than that in the HSD group (). At week 12, HSD + Cana group rats had significantly lower serum creatinine (p < 0.01) and proteinuria (p < 0.05) than HSD group rats after the HSD challenge (). In , we observed that the HSD resulted in renal fibrosis. After 12 weeks of canagliflozin treatment, the degree of fibrosis was significantly reduced ().
Figure 1. Effects of canagliflozin on blood pressure, oxidative stress, and renal fibrosis. (A) Blood pressure; (B) Serum creatinine; (C) Urinary protein excretion; (D) Serum SOD activity; (E) Semi-quantification of fibrosis in the left kidney from the Masson trichrome-stained section; (F) Representative left renal sections stained by Masson trichrome staining; HSD, high-salt diet. *p < 0.05, **p < 0.01 vs. NSD. #p < 0.05, ##p < 0.01 vs. HSD.
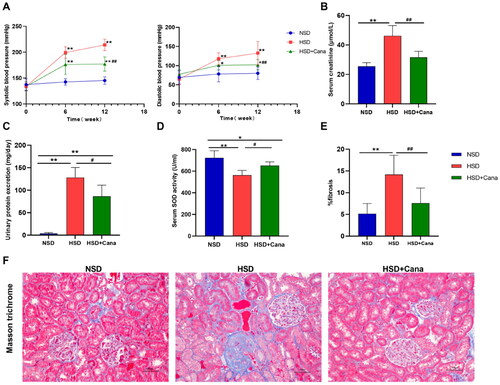
As oxidative stress is closely related to renal fibrosis, the influence of canagliflozin on oxidative stress was determined. In , we found that the HSD reduced the serum activity of SOD, whereas SOD serum activity was significantly increased (p < 0.05) after canagliflozin. These data demonstrate the substantial protection of the canagliflozin-fed DSS rats against salt-induced renal injury and oxidative stress compared to the disease-prone HSD group rats.
Effects of canagliflozin on gut microbiota community structure
There was no significant difference in alpha diversity among the three groups, as indicated by the Simpson and Chao1 indices (). In contrast, the beta diversity analysis showed significant differences in the composition and abundance of the gut microbiota (p < 0.01, ). The overall difference in the gut microbial community structure resulting from HSD and canagliflozin was evaluated using unsupervised PCoA of Bray–Curtis dissimilarity (). The principal coordinates PCo1 and PCo2 explained 37.9% and 15.8% of the variation in Bray–Curtis dissimilarity, respectively ().
Canagliflozin induces core changes in the composition and diversity of the gut microbiota
At the phylum level, the results indicated that the dominant bacteria in all groups were Firmicutes, Actinobacteria, Verrucomicrobia, Proteobacteria, Bacteroidetes, Cyanobacteria, Chloroflexi, Tenericutes, Thermi and TM7 (). Among them, the abundance of Firmicutes and Actinobacteria was highest in the three groups. Canagliflozin increased the abundance of Actinobacteria and decreased the abundance of Firmicutes. At the genus level, the results indicated that the dominant bacteria in all groups were Enterococcus spp., Allobaculum spp., Blautia spp., Lactobacillus spp., Corynebacterium spp., Aerococcus spp., Akkermansia spp., Clostridium spp., Dorea spp., and Jeotgalicoccus spp. (). LEfSe () analysis showed that these taxa were significantly more abundant than the others. Based on the plots, the families Clostridiaceae and Peptostreptococcaceae were enriched in the NSD group; the phylum Firmicutes and family Lachnospiraceae were more abundant in the HSD group; and the genus Allobaculum spp., the family Erysipelotrichales, and the phylum Actinobacteria and its uncultured genus were enriched in the HSD + Cana group, including Corynebacterium spp. and Bifidobacterium spp.(). In the metagenomeSeq analysis, at the genus level, the abundance of Corynebacterium spp., Bifidobacterium spp., Facklamia spp., Lactobacillus spp, Ruminococcus spp., Blautia spp., Coprococcus spp., and Allobaculum spp. was significantly different in the HSD and HSD + Cana groups, with the abundance being significantly increased after canagliflozin treatment ().
Figure 3. Canagliflozin-mediated changes of gut microbiota in HSD-fed DSS rats. (A) Average relative percentage abundance of top 10 phylume; (B) Average relative percentage abundance of top 10 genus; (C) Taxonomic cladogram generated from LEfSe analysis of 16S rRNA gene sequences. Each circles size is proportional to the taxon’s abundance. LEfSe analysis showed that these taxa were significantly more abundant in comparison to the other groups; (D) Genus with significant difference between the HSD and HSD + Cana group in metagenome Seq analysis. HSD, high-salt diet.
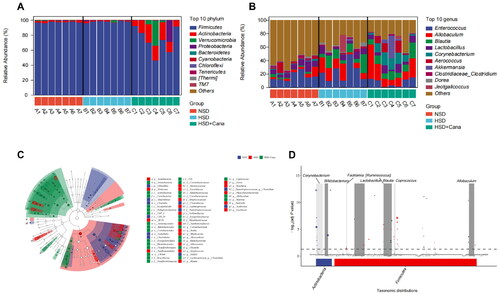
Canagliflozin relieves kidney injury by reducing uremic toxins
A total of 275 metabolites were identified by LC/MS, including 81 carboxylic acids and derivatives, 42 fatty acids, 32 organooxygen compounds, 26 steroids and steroid derivatives, and 94 other small molecules (). Carboxylic acids and derivatives were the predominant metabolites, accounting for 29.5% of all metabolites (). Most of the detected carboxylic acids and derivatives were amino acids. To understand how canagliflozin affects serum metabolites in DSS rats, differentially abundant metabolites between the NSD and HSD groups and differentially abundant metabolites between the HSD and HSD + Cana groups were screened. A total of 77 differentially abundant metabolites were screened out between the HSD and NSD groups (). There were 24 kinds of amino acids among these differentially abundant metabolites, and related metabolites made up a significant proportion (). After the canagliflozin treatment, compared with the HSD group, a total of 56 metabolites were significantly changed. These differentially abundant metabolites contained 26 kinds of amino acids and related metabolites ().
Figure 4. Canagliflozin alleviates kidney injury by regulating intestinal microorganisms to reduce urinary toxin concentration. (A) The proportion of all metabolites detected in three groups according to chemical classification; (B) Heatmaps of serum metabolites correlations in three groups; (C) Clustering heat map of metabolites with partial significant differences between NSD and HSD groups; (D) Clustering heat map of metabolites with partial significant differences between HSD and HSD + Cana groups; (E) Serum metabolites, gut microbiota, and their correlations. The heatmaps with gradient colors of blue, white, and red illustrate the Spearman correlations of the relative abundance of microbes and the concentrations of serum metabolites. The r values are represented by gradient colors, where red and blue cells indicate positive and negative correlations, respectively. *p < 0.05, **p < 0.01. HSD, high-salt diet.
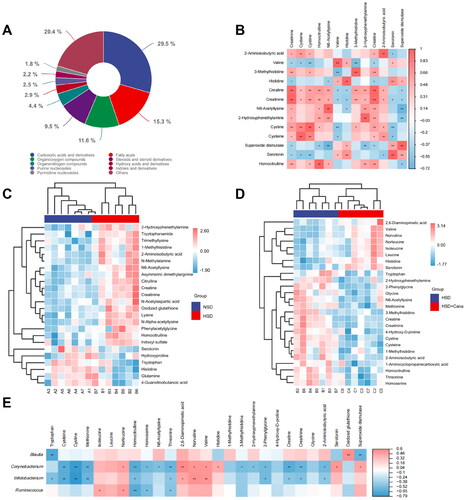
The HSD increased serum levels of known toxic metabolites (methyhistidines, creatinine, homocitrulline, and indoxyl sulfate) in serum (). After canagliflozin treatment, amino acid metabolites, including 2,6-diaminopimelic acid, valine, norvaline, norleucine, isoleucine, leucine, histidine, and serotonin, were increased significantly compared to those in the HSD group (). In contrast, tryptophan, 2-hydroxyphenethylamine, 2-phenylglycine, glycine, N6-acetyllysine, methionine, 3-methylhistidine, creatine, creatinine 4-hydroxy-D-proline, cystine, cysteine, 1-methylhistidine, 2-aminoisobutyric acid, 1-aminocyclopropanecarboxylic acid, homocitrulline, threonine, and homoserine were decreased significantly after the intervention of canagliflozin (). Canagliflozin reduced uremic toxins. In addition, we found that compared with the NSD group, oxidized glutathione was significantly increased in the HSD group and decreased after canagliflozin treatment (). Findings for serum biochemical indices (e.g. creatinine and SOD) also supported the abovementioned results ().
Canagliflozin alleviates kidney injury by regulating intestinal microorganisms to reduce the concentrations of urinary toxins
Serum creatinine was reduced in the HSD + Cana group compared to the HSD group, and the difference was statistically significant (). To further study the correlation between differentially abundant metabolites and renal function in the HSD and HSD + Cana groups, we performed a Spearman correlation between creatinine and the other differentially abundant metabolites (). These results showed that serum creatinine was significantly correlated with cysteine, cystine, homocitrulline, N6-acetyllysine, valine, histidine, 3-methylhistidine, 2-hydroxyphenethylamine, creatine, 2-aminoisobutyric acid, and serotonin. Serum creatinine was also negatively correlated with SOD activity ().
We then performed a Spearman correlation between the amino acid metabolites and the microbes that significantly differed between the HSD and HSD + Cana groups. We observed that Corynebacterium spp., Blautia spp., Bifidobacterium spp., and Ruminococcus spp. were significantly correlated with specific amino acids (). Notably, the relative abundance of Bifidobacterium spp. was negatively correlated with creatinine and homocitrulline and the relative abundance of Corynebacterium spp. was negatively correlated with creatinine, 3-methylhistidine, and homocitrulline (). The relative abundance of Blautia spp. was negatively correlated with SOD activity. However, the relative abundance of Ruminococcus spp. was negatively correlated with homocitrulline (). Canagliflozin treatment reconstructed the intestinal flora and resulted in metabolite changes, alleviating the metabolic disorders caused by HSD.
Discussion
Recent studies in patients with moderate to severe CKD have shown that SGLT2 inhibitors (canagliflozin and dapagliflozin) have renoprotective effects [Citation24,Citation25]. The underlying mechanism of the renal protection induced by SGLT2 inhibitors likely involves multiple factors, including tubuloglomerular feedback activation, the promotion of diuresis and natriuresis, a reduction in renal interstitial inflammation, an increase in hematocrit and the mitigation of hypoxia in tubular cells, among others [Citation26,Citation27]. The present study demonstrated that canagliflozin treatment attenuated the salt-sensitive hypertension, renal damage, and oxidative stress caused by an HSD; moreover, canagliflozin induced significant shifts in the gut microbiota composition of HSD + Cana group rats. Supportive evidence from other animal models of hypertension has also demonstrated pathogenic factors derived directly from the hypertensive gut microbiota. Compared with their respective normotensive strains and models, most experimental hypertension model animals studied to date, including spontaneously hypertensive rat (SHR), DSS, angiotensin II, and deoxycorticosterone acetate (DOCA)-salt model animals, have altered gut microbiota [Citation28–30]. Similarly, a single transplantation of feces from hypertensive humans results in elevated BP in germ-free mice [Citation7]. An animal study demonstrated that an HSD directly contributes to the development of DSS hypertension and renal injury, and dietary modifications and nutritional interventions can reduce kidney injury through the gut microbiota [Citation31]. Another study investigated whether doxycycline (a broad-spectrum tetracycline antibiotic) improves dysbiosis, prevents cardiovascular pathology, and attenuates hypertension in DOCA-salt rats, a renin-independent rat model of hypertension [Citation32]. Our work highlights the potential power of gut microbiota modulation through canagliflozin intervention in regulating BP and kidney disease, similar to the above findings.
It is increasingly recognized that the microbiome may affect the health and disease of the host [Citation33,Citation34]. Animal and clinical studies have shown that canagliflozin inhibits intestinal SGLT1, thereby altering the composition of the intestinal microbiota [Citation17–19,Citation35,Citation36]. Our study also found canagliflozin-mediated changes in the gut microbiota in HSD-fed DSS rats. Upon closer examination of the statistically significant ASVs, we can gain insight into potential bacterial functions and gut microbiota-derived metabolites that may be responsible for the ultimate pathological effects. LEfSe analysis showed that the phylum Firmicutes was more abundant in the HSD group, and its abundance decreased in the HSD + Cana group after canagliflozin treatment. The increase in Firmicutes in hypertension may be due to Firmicutes being the main phylum that produces TMA [Citation37], which exacerbates hypertension. Compared with the HSD group, EtagenomeSeq analysis showed the abundance of Corynebacterium spp., Bifidobacterium spp., Facklamia spp., Lactobacillus spp., Ruminococcus spp., Blautia spp., Coprococcus spp., and Allobaculum spp. was significantly increased in the HSD + Cana group. Lactobacillus [Citation38], Coprococcus [Citation39], Bifidobacterium [Citation40], and Allobaculum [Citation41] were enriched in healthy or treated groups with lower BP and were negatively correlated with BP. By providing probiotic supplements to DSS rats fed with HSD, the relative abundance of Lactobacillus and Bifidobacterium increased, which changed the production of metabolites and reduced the level of uremic toxins, ultimately leading to reduced renal injury [Citation42]. Similarly, in DOCA-salt hypertension rats, supplementation with probiotic Bifidobacterium breve can also induce changes in the gut microbiota linked with the attenuation of endothelial dysfunction and an increase in BP in this low-renin form of hypertension, and these beneficial effects seem to be mediated by increased acetate and reduced TMA production by gut microbiota [Citation43]. Blautia is a genus of anaerobic bacteria with probiotic characteristics that are widely present in the feces and intestines of mammals [Citation44]. A longitudinal study of Japanese subjects showed that changes in the abundance ratios of two Blautia species, Blautia hansenii and Blautia producta, were significantly and negatively associated with visceral fat area changes [Citation45]. This may partially explain the mechanism by which SGLT2 inhibitors (including canagliflozin) can reduce the abdominal visceral fat area and may influence renal function in patients with type 2 diabetes [Citation46]. These results support our research. After 12 weeks of treatment with canagliflozin, the relative abundance of Lactobacillus spp., Bifidobacterium spp., Allobaculum spp., and Coprococcus spp. increased and hypertension and renal injury were alleviated.
Uremic toxins associated with renal function can be classified based on their origin: endogenous (mammalian metabolism), exogenous (diet) or microbial [Citation47,Citation48]. Several circulating metabolites derived from bacterial protein fermentation have been found to be inversely associated with renal function [Citation49]. A deeper understanding of the gut-microbe-metabolite axis is a prerequisite to further clarify the mechanism of canagliflozin in alleviating renal injury. Therefore, we examined the serum metabolome and analyzed the correlation between intestinal microecology and metabolites. In our study, HSD treatment destroyed the intestinal flora and increased serum levels of known toxic metabolites (methyhistidines, creatinine, homocitrulline, and indoxyl sulfate). After intestinal microflora reconstruction by canagliflozin, these toxic metabolites (methyhistidines, creatinine, and homocitrulline) were significantly reduced in serum, thus alleviating renal injury caused by the HSD. Similar clinical studies have shown that these measured metabolites, including tryptophan-derived uremic toxins (indoxyl sulfate) and metabolites involved in histamine metabolism (methyhistidines), and azotemia (homocitrulline), are associated with CKD progression [Citation50,Citation51]. The main functions of the activity appear to be altered monocyte activation, intensified inflammatory processes, and augmented oxidative stress by these uremic toxins [Citation52]. Serum biochemical index (e.g. creatinine and SOD) findings also support the abovementioned results. Serum creatinine is a gauge of kidney function, and SOD is a classic indicator of oxidative stress. Compared with the HSD group, the serum creatinine concentration was significantly decreased and SOD activity was significantly increased in the HSD + Cana group. This research found that serum creatinine was positively related to homocitrulline and 3-methylhistidine. In addition, our study showed that three metabolites related to tryptophan metabolism changed significantly among the three groups, including tryptophan, indoxyl sulfate, and serotonin. Tryptophan catabolites also modulate neural activity and are active in the systemic inflammatory cascade [Citation53]. Changing the proportion of the plasma tryptophan concentration in patients with CKD is related to improving oxidative stress [Citation54]. In summary, our study found that canagliflozin reduced these toxic metabolites and oxidative stress, thus alleviating HSD-induced renal injury.
Recent studies have revealed a link between intestinal microorganisms and circulatory metabolites in CKD. The shift toward a predominantly proteolytic fermentation pattern in CKD promotes increased microbiota-derived toxins and systemic inflammation [Citation55]. We observed that Corynebacterium spp., Blautia spp., Bifidobacterium spp., and Ruminococcus spp. were significantly correlated with specific amino acids. Ruminococcaceae families are inversely associated with inflammatory bowel disease and are considered butyrate producers [Citation56,Citation57]. In a rat model of diabetic nephropathy, the increased abundance of Ruminococcaceae, Blautia and Bifidobacterium was associated with reduced inflammation and oxidative stress [Citation58]. A clinical study has shown that synbiotic therapy significantly modified the gut microbiome with Bifidobacteria and Lactobacillus enrichment and consequently reduced the serum level of indoxyl sulfate, improved the estimated glomerular filtration rate, and decreased the level of high-sensitivity C-reactive protein in treated patients [Citation59]. Previous studies showed that the relative abundance of Lactobacillus and Bifidobacterium increased by supplementing DSS rats with L. plantarum ZDY2013, which changed the production of metabolites, reduced the level of uremic toxins in the blood circulation and alleviated renal injury [Citation42]. Bifidobacterium is generally known as a beneficial bacterium that produces SCFAs. SGLT2 inhibitor (luseogliflozin) treatment was found to increase the abundance of intestinal bacteria involved in the synthesis of SCFAs, leading to improved amino acid metabolism [Citation60]. There may be some controversy about Corynebacterium. Our study showed that Corynebacterium presented a significant positive correlation with SOD activity and a negative correlation with creatinine concentration. Corynebacterium is generally believed to be part of the human microbiome but can cause the development of various localization-related inflammatory diseases in various locations [Citation61]. However, Corynebacterium glutamicum is an exception. Corynebacterium glutamicum is a bacterium with a powerful role in amino acid production, and this may explain the positive correlation between Corynebacterium and multiple amino acids [Citation62]. Unfortunately, our study could not distinguish C. glutamicum from other Corynebacterium species; however, this did not affect the conclusion of our study. Another study showed that canagliflozin could exert intestinal effects that reduced the accumulation of uremic toxins [Citation19]. Our findings add to the body of knowledge on the gut-renal axis in the pathophysiology of CKD.
Conclusions
This study used an integrated approach of 16S rRNA gene sequencing combined with LC–MS-based metabolomics to assess the changes and variations in the gut microbiota in hypertensive kidney injury rats treated with canagliflozin. After 12 weeks of treatment with canagliflozin, the intestinal flora of the model rats was reconstructed, which changed the production of metabolites and reduced the level of uremic toxins in the blood circulation, ultimately alleviating oxidative stress and renal injury. Our results provide a theoretical basis for the treatment of salt-sensitive hypertension-induced kidney injury with canagliflozin. This study has some limitations. First, the present study is a correlative study, and further research is needed to investigate the effects of canagliflozin on the intestinal flora of DSS rats and the mechanisms related to protecting renal function. Second, the effect of canagliflozin on renal haemodynamics was not evaluated in this study.
Funding statement
The study was supported by The 2019 Hebei Science and Technology Project (No. 19277787D), The 2019 Hebei Innovation Capability Promotion Project (No. 199776249D), the 2020 medical science research project of Hebei Provincial Health Commission (No. 20200724).
Disclosure statement
All authors declare that they have no conflict of interest.
Data availability
The data used to support the findings of this study are available from the corresponding author upon request.
References
- Wang J, Wang Z, Guo F, et al. Individual and combined cardiometabolic morbidities and the subsequent risk of cardiovascular events in Chinese adults. J Clin Endocrinol Metab. 2022;107(1):1–10. doi: 10.1210/clinem/dgab609.
- Hirohama D, Fujita T. Evaluation of the pathophysiological mechanisms of salt-sensitive hypertension. Hypertens Res. 2019;42(12):1848–1857. doi: 10.1038/s41440-019-0332-5.
- Iatrino R, Manunta P, Zagato L. Salt sensitivity: challenging and controversial phenotype of primary hypertension. Curr Hypertens Rep. 2016;18(9):70. doi: 10.1007/s11906-016-0677-y.
- Drenjančević-Perić I, Jelaković B, Lombard JH, et al. High-salt diet and hypertension: focus on the renin-angiotensin system. Kidney Blood Press Res. 2011;34(1):1–11. doi: 10.1159/000320387.
- Jama HA, Beale A, Shihata WA, et al. The effect of diet on hypertensive pathology: is there a link via gut microbiota-driven immunometabolism? Cardiovasc Res. 2019;115(9):1435–1447. doi: 10.1093/cvr/cvz091.
- Marques FZ, Mackay CR, Kaye DM. Beyond gut feelings: how the gut microbiota regulates blood pressure. Nat Rev Cardiol. 2018;15(1):20–32. doi: 10.1038/nrcardio.2017.120.
- Li J, Zhao F, Wang Y, et al. Gut microbiota dysbiosis contributes to the development of hypertension. Microbiome. 2017;5(1):14. doi: 10.1186/s40168-016-0222-x.
- de Araújo Henriques Ferreira G, Magnani M, Cabral L, et al. Potentially probiotic Limosilactobacillus fermentum fruit-derived strains alleviate cardiometabolic disorders and gut microbiota impairment in male rats fed a high-fat diet. Probiotics Antimicrob Proteins. 2022;14(2):349–359. doi: 10.1007/s12602-021-09889-y.
- Poll BG, Cheema MU, Pluznick JL. Gut microbial metabolites and blood pressure regulation: focus on SCFAs and TMAO. Physiology (Bethesda). 2020;35(4):275–284. doi: 10.1152/physiol.00004.2020.
- Cosola C, Rocchetti MT, Cupisti A, et al. Microbiota metabolites: pivotal players of cardiovascular damage in chronic kidney disease. Pharmacol Res. 2018;130:132–142. doi: 10.1016/j.phrs.2018.03.003.
- Tokarek J, Budny E, Saar M, et al. Does the composition of gut microbiota affect hypertension? Molecular mechanisms involved in increasing blood pressure. Int J Mol Sci. 2023;24(2):1377. doi: 10.3390/ijms24021377.
- Rayego-Mateos S, Valdivielso JM. New therapeutic targets in chronic kidney disease progression and renal fibrosis. Expert Opin Ther Targets. 2020;24(7):655–670. doi: 10.1080/14728222.2020.1762173.
- Sueyoshi M, Fukunaga M, Mei M, et al. Effects of lactulose on renal function and gut microbiota in adenine-induced chronic kidney disease rats. Clin Exp Nephrol. 2019;23(7):908–919. doi: 10.1007/s10157-019-01727-4.
- Tanada Y, Okuda J, Kato T, et al. The metabolic profile of a rat model of chronic kidney disease. PeerJ. 2017;5:e3352. doi: 10.7717/peerj.3352.
- Bigazzi R, Bianchi S, Baldari D, et al. Microalbuminuria in salt-sensitive patients. A marker for renal and cardiovascular risk factors. Hypertension. 1994;23(2):195–199. doi: 10.1161/01.hyp.23.2.195.
- Chakraborty S, Mandal J, Cheng X, et al. Diurnal timing dependent alterations in gut microbial composition are synchronously linked to salt-sensitive hypertension and renal damage. Hypertension. 2020;76(1):59–72. doi: 10.1161/HYPERTENSIONAHA.120.14830.
- Sokolov V, Yakovleva T, Chu L, et al. Differentiating the sodium-glucose cotransporter 1 inhibition capacity of canagliflozin vs. Dapagliflozin and Empagliflozin using quantitative systems pharmacology modeling. CPT Pharmacometrics Syst Pharmacol. 2020;9(4):222–229. doi: 10.1002/psp4.12498.
- Ho H-J, Kikuchi K, Oikawa D, et al. SGLT-1-specific inhibition ameliorates renal failure and alters the gut microbial community in mice with adenine-induced renal failure. Physiol Rep. 2021;9(24):e15092. doi: 10.14814/phy2.15092.
- Mishima E, Fukuda S, Kanemitsu Y, et al. Canagliflozin reduces plasma uremic toxins and alters the intestinal microbiota composition in a chronic kidney disease mouse model. Am J Physiol Renal Physiol. 2018;315(4):F824–F833. doi: 10.1152/ajprenal.00314.2017.
- Bolyen E, Rideout JR, Dillon MR, et al. Reproducible, interactive, scalable and extensible microbiome data science using QIIME 2. Nat Biotechnol. 2019;37(8):852–857. doi: 10.1038/s41587-019-0209-9.
- Callahan BJ, McMurdie PJ, Rosen MJ, et al. DADA2: high-resolution sample inference from Illumina amplicon data. Nat Methods. 2016;13(7):581–583. doi: 10.1038/nmeth.3869.
- DeSantis TZ, Hugenholtz P, Larsen N, et al. Greengenes, a chimera-checked 16S rRNA gene database and workbench compatible with ARB. Appl Environ Microbiol. 2006;72(7):5069–5072. doi: 10.1128/AEM.03006-05.
- Zhang Z, Wang X, Wang J, et al. Metabonomics approach to assessing the metabolism variation and endoexogenous metabolic interaction of ginsenosides in cold stress rats. J Proteome Res. 2016;15(6):1842–1852. doi: 10.1021/acs.jproteome.6b00015.
- Perkovic V, Jardine MJ, Neal B, et al. Canagliflozin and renal outcomes in type 2 diabetes and nephropathy. N Engl J Med. 2019;380(24):2295–2306. doi: 10.1056/NEJMoa1811744.
- Heerspink HJL, Stefánsson BV, Correa-Rotter R, et al. Dapagliflozin in patients with chronic kidney disease. N Engl J Med. 2020;383(15):1436–1446. doi: 10.1056/NEJMoa2024816.
- Leoncini G, Russo E, Bussalino E, et al. SGLT2is and renal protection: from biological mechanisms to real-world clinical benefits. Int J Mol Sci. 2021;22(9):4441. doi: 10.3390/ijms22094441.
- Isidto R, Danguilan R, Naidas O, et al. Emerging role of sodium-glucose co-transporter 2 inhibitors for the treatment of chronic kidney disease. Int J Nephrol Renovasc Dis. 2023;16:43–57. doi: 10.2147/IJNRD.S387262.
- Mell B, Jala VR, Mathew AV, et al. Evidence for a link between gut microbiota and hypertension in the Dahl rat. Physiol Genomics. 2015;47(6):187–197. doi: 10.1152/physiolgenomics.00136.2014.
- Adnan S, Nelson JW, Ajami NJ, et al. Alterations in the gut microbiota can elicit hypertension in rats. Physiol Genomics. 2017;49(2):96–104. doi: 10.1152/physiolgenomics.00081.2016.
- Marques FZ, Nelson E, Chu PY, et al. High-fiber diet and acetate supplementation change the gut microbiota and prevent the development of hypertension and heart failure in hypertensive mice. CIRCULATION. 2017;135(10):964–977. doi: 10.1161/CIRCULATIONAHA.116.024545.
- Abais-Battad JM, Saravia FL, Lund H, et al. Dietary influences on the Dahl SS rat gut microbiota and its effects on salt-sensitive hypertension and renal damage. Acta Physiol (Oxf). 2021;232(4):e13662. doi: 10.1111/apha.13662.
- Robles-Vera I, de la Visitación N, Toral M, et al. Changes in gut microbiota induced by doxycycline influence in vascular function and development of hypertension in DOCA-salt rats. Nutrients. 2021;13(9):2971. doi: 10.3390/nu13092971.
- Hollister Emily B, Gao C, Versalovic J. Compositional and functional features of the gastrointestinal microbiome and their effects on human health. GASTROENTEROLOGY. 2014;146(6):1449–1458. doi: 10.1053/j.gastro.2014.01.052.
- Takagi T, Naito Y, Kashiwagi S, et al. Changes in the gut microbiota are associated with hypertension, hyperlipidemia, and type 2 diabetes mellitus in Japanese subjects. Nutrients. 2020;12(10):2996. doi: 10.3390/nu12102996.
- Polidori D, Sha S, Mudaliar S, et al. Canagliflozin lowers postprandial glucose and insulin by delaying intestinal glucose absorption in addition to increasing urinary glucose excretion: results of a randomized, placebo controlled study. Diabetes Care. 2013;36(8):2154–2161. doi: 10.2337/dc12-2391.
- Wang L, Liang C, Song X, et al. Canagliflozin alters the gut, oral, and ocular surface microbiota of patients with type 2 diabetes mellitus. Front Endocrinol (Lausanne). 2023;14:1256292. doi: 10.3389/fendo.2023.1256292.
- Fennema D, Phillips IR, Shephard EA. Trimethylamine and trimethylamine N‐oxide, a flavin‐containing monooxygenase 3 (FMO3)‐mediated host‐microbiome metabolic axis implicated in health and disease. Drug Metab Dispos. 2016;44(11):1839–1850. doi: 10.1124/dmd.116.070615.
- Wiest R, Chen F, Cadelina G, et al. Effect of lactobacillus-fermented diets on bacterial translocation and intestinal flora in experimental prehepatic portal hypertension. Dig Dis Sci. 2003;48(6):1136–1141. doi: 10.1023/a:1023729115659.
- Vijay A, Astbury S, Panayiotis L, et al. Dietary interventions reduce traditional and novel cardiovascular risk markers by altering the gut microbiome and their metabolites. Front Cardiovasc Med. 2021;8:691564. doi: 10.3389/fcvm.2021.691564.
- Liu J, An N, Ma C, et al. Correlation analysis of intestinal flora with hypertension. Exp Ther Med. 2018;16(3):2325–2330. doi: 10.3892/etm.2018.6500.
- Guo H, Hao Y, Fan X, et al. Administration with quinoa protein reduces the blood pressure in spontaneously hypertensive rats and modifies the fecal microbiota. Nutrients. 2021;13(7):2446. doi: 10.3390/nu13072446.
- Wan C, Chen S, Zhao K, et al. Serum untargeted metabolism reveals the mechanism of L. plantarum ZDY2013 in alleviating kidney injury induced by high-salt diet. Nutrients. 2021;13(11):3920. doi: 10.3390/nu13113920.
- Robles-Vera I, de la Visitación N, Toral M, et al. Probiotic Bifidobacterium breve prevents DOCA-salt hypertension. Faseb J. 2020;34(10):13626–13640. doi: 10.1096/fj.202001532R.
- Liu X, Mao B, Gu J, et al. Blautia-a new functional genus with potential probiotic properties? Gut Microbes. 2021;13(1):1–21. doi: 10.1080/19490976.2021.1875796.
- Ozato N, Yamaguchi T, Mori K, et al. Two Blautia species associated with visceral fat accumulation: a one-year longitudinal study. Biology (Basel). 2022;11(2):318. doi: 10.3390/biology11020318.
- Tosaki T, Kamiya H, Himeno T, et al. Sodium-glucose co-transporter 2 inhibitors reduce the abdominal visceral fat area and may influence the renal function in patients with type 2 diabetes. Intern Med. 2017;56(6):597–604. doi: 10.2169/internalmedicine.56.7196.
- Koppe L, Fouque D, Soulage CO. The role of gut microbiota and diet on uremic retention solutes production in the context of chronic kidney disease. Toxins (Basel). 2018;10(4):155. doi: 10.3390/toxins10040155.
- Rukavina Mikusic NL, Kouyoumdzian NM, Choi MR. Gut microbiota and chronic kidney disease: evidences and mechanisms that mediate a new communication in the gastrointestinal-renal axis. Pflugers Arch. 2020;472(3):303–320. doi: 10.1007/s00424-020-02352-x.
- Barrios C, Beaumont M, Pallister T, et al. Gut-microbiota-metabolite axis in early renal function decline. PLoS One. 2015;10(8):e0134311. doi: 10.1371/journal.pone.0134311.
- Wen D, Zheng Z, Surapaneni A, et al. Metabolite profiling of CKD progression in the chronic renal insufficiency cohort study. JCI Insight. 2022;7(20):e161696. doi: 10.1172/jci.insight.161696.
- Uehara T, Horinouchi A, Morikawa Y, et al. Identification of metabolomic biomarkers for drug-induced acute kidney injury in rats. J Appl Toxicol. 2014;34(10):1087–1095. doi: 10.1002/jat.2933.
- Kamiński TW, Pawlak K, Karbowska M, et al. Indoxyl sulfate – the uremic toxin linking hemostatic system disturbances with the prevalence of cardiovascular disease in patients with chronic kidney disease. BMC Nephrol. 2017;18(1):35. doi: 10.1186/s12882-017-0457-1.
- Roth W, Zadeh K, Vekariya R, et al. Tryptophan metabolism and gut-brain homeostasis. Int J Mol Sci. 2021;22(6):2973. doi: 10.3390/ijms22062973.
- Zinellu A, Sotgia S, Mangoni AA, et al. Impact of cholesterol lowering treatment on plasma kynurenine and tryptophan concentrations in chronic kidney disease: relationship with oxidative stress improvement. Nutr Metab Cardiovasc Dis. 2015;25(2):153–159. doi: 10.1016/j.numecd.2014.11.004.
- Kim SM, Song IH. The clinical impact of gut microbiota in chronic kidney disease. Korean J Intern Med. 2020;35(6):1305–1316. doi: 10.3904/kjim.2020.411.
- Vital M, Howe AC, Tiedje JM. Revealing the bacterial butyrate synthesis pathways by analyzing (meta)genomic data. mBio. 2014;5(2):e00889. doi: 10.1128/mBio.00889-14.
- Kostic AD, Xavier RJ, Gevers D. The microbiome in inflammatory bowel disease: current status and the future ahead. Gastroenterology. 2014;146(6):1489–1499. doi: 10.1053/j.gastro.2014.02.009.
- Zhang Z, Li Q, Liu F, et al. Lycoperoside H protects against diabetic nephropathy via alteration of gut microbiota and inflammation. J Biochem Mol Toxicol. 2022;36(12):e23216. doi: 10.1002/jbt.23216.
- Mitrović M, Stanković-Popović V, Tolinački M, et al. The impact of synbiotic treatment on the levels of gut-derived uremic toxins, inflammation, and gut microbiome of chronic kidney disease patients-a randomized trial. J Ren Nutr. 2022;33(2):278–288. doi: 10.1053/j.jrn.2022.07.008.
- Hata S, Okamura T, Kobayashi A, et al. Gut microbiota changes by an SGLT2 inhibitor, Luseogliflozin, alters metabolites compared with those in a low carbohydrate diet in db/db mice. Nutrients. 2022;14(17):3531. doi: 10.3390/nu14173531.
- Mangutov EO, Alieva AA, Kharseeva GG, et al. Corynebacterium spp.: relationship of pathogenic properties and antimicrobial resistance. Klin Lab Diagn. 2022;67(9):519–524. doi: 10.51620/0869-2084-2022-67-9-519-524.
- Tsuge Y, Matsuzawa H. Recent progress in production of amino acid-derived chemicals using Corynebacterium glutamicum. World J Microbiol Biotechnol. 2021;37(3):49. doi: 10.1007/s11274-021-03007-4.