Abstract
Increasing evidence suggests that peritoneal fibrosis induced by peritoneal dialysis (PD) is linked to oxidative stress. However, there are currently no effective interventions for peritoneal fibrosis. In the present study, we explored whether adding caffeic acid phenethyl ester (CAPE) to peritoneal dialysis fluid (PDF) improved peritoneal fibrosis caused by PD and explored the molecular mechanism. We established a peritoneal fibrosis model in Sprague–Dawley rats through intraperitoneal injection of PDF and lipopolysaccharide (LPS). Rats in the PD group showed increased peritoneal thickness, submesothelial collagen deposition, and the expression of TGFβ1 and α-SMA. Adding CAPE to PDF significantly inhibited PD-induced submesothelial thickening, reduced TGFβ1 and α-SMA expression, alleviated peritoneal fibrosis, and improved the peritoneal ultrafiltration function. In vitro, peritoneal mesothelial cells (PMCs) treated with PDF showed inhibition of the AMPK/SIRT1 pathway, mitochondrial membrane potential depolarization, overproduction of mitochondrial reactive oxygen species (ROS), decreased ATP synthesis, and induction of mesothelial-mesenchymal transition (MMT). CAPE activated the AMPK/SIRT1 pathway, thereby inhibiting mitochondrial membrane potential depolarization, reducing mitochondrial ROS generation, and maintaining ATP synthesis. However, the beneficial effects of CAPE were counteracted by an AMPK inhibitor and siSIRT1. Our results suggest that CAPE maintains mitochondrial homeostasis by upregulating the AMPK/SIRT1 pathway, which alleviates oxidative stress and MMT, thereby mitigating the damage to the peritoneal structure and function caused by PD. These findings suggest that adding CAPE to PDF may prevent and treat peritoneal fibrosis.
Introduction
Peritoneal dialysis (PD) is a viable and efficient approach for end-stage renal disease (ESRD) [Citation1,Citation2]. However, extended utilization of biologically incompatible peritoneal dialysis fluid (PDF) and peritoneal infections during PD treatment may result in peritoneal fibrosis [Citation3–7] and ultrafiltration failure [Citation8].
In response to PD, peritoneal mesothelial cells (PMCs) undergo a mesothelial-to-mesenchymal transition (MMT) in the early stages of peritoneal fibrosis [Citation9,Citation10]. Oxidative stress plays a driving part in the progression of MMT [Citation11–17]. Mitochondria are the primary source of reactive oxygen species (ROS) [Citation18–21]. Studies have shown that clearing mitochondrial ROS can improve TGF-β1-induced MMT in PMCs [Citation22]. Therefore, we hypothesize that mitochondrial homeostasis may be a target for preventing and treating MMT during PD.
Up to now, there are no effective interventions for PD-induced peritoneal fibrosis. Some researchers attempted to add molecular hydrogen (H2) to PDF and found that it can reduce oxidative stress and alleviate peritoneal fibrosis [Citation23–25]. Caffeic acid phenethyl ester (CAPE), which is a dietary drug and a potent antioxidant [Citation26,Citation27], has been shown to alleviate neuronal damage induced by various harmful factors via adjusting the AMPK/SIRT1 pathway [Citation28,Citation29]. Additionally, multiple other studies suggest that the beneficial impacts of CAPE are achieved through AMPK activation [Citation30–32]. Therefore, we hypothesized that CAPE may regulate mitochondrial homeostasis by adjusting the AMPK/SIRT1 pathway, thereby mitigating the damage to the peritoneum caused by PD.
In this study, we explored the impacts of adding CAPE to PDF on PD-related peritoneal fibrosis in vivo and investigated its impact on mitochondrial homeostasis and the AMPK/SIRT1 pathway in vitro. The results of this study offer fresh perspectives on the treatment approaches for PD-related peritoneal fibrosis.
Materials and methods
Animals
Male 7-week-old Sprague-Dawley rats weighing 200–220 g were acquired from the Experimental Animal Center of Soochow University. They were kept in a regulated environment at a temperature of 22 ± 1 °C, following a 12-h light-dark cycle. The rats were provided with unrestricted access to food and water. The study followed the principles outlined in the Declaration of Helsinki and was approved by the Ethics Committee for Experimental Animal Care and Use at Soochow University (Approval No JD-LK-2021-003-01).
Animal model
An animal model was conducted as previously described [Citation33]. Briefly, rats were intraperitoneally injected with 20 mL PDF containing 4.25% glucose (Baxter Health care, USA) daily and intraperitoneally injected with 0.6 mg/kg of lipopolysaccharide (LPS) (Sigma–Aldrich, L2012) on days 1, 3, 5, and 7. Rats were euthanized on days 0, 7, 14, and 28, and peritoneal tissues were collected.
In vivo experimental design
Rats were randomly divided into four groups with 8 rats in each group as follows: (i) intraperitoneal injection of 20 mL/day of normal saline and defined as the control group; (ii) intraperitoneal injection of 20 mL/day of normal saline containing CAPE (Selleck, S7414) at a daily dose of 4 mg/kg and defined as the CAPE group; (iii) intraperitoneal injection of 20 mL/day of 4.25% PDF and intraperitoneal injection of 0.6 mg/kg of LPS on days 1, 3, 5, and 7 and defined as the PD group; and (iv) addition of CAPE at a daily dose of 4 mg/kg to the PDF in the PD group and defined as the PD + CAPE group. All the rats were in good condition with stable weight gain throughout the entire experiment. On the 28th day of the experiment, rats were euthanized after the peritoneal equilibrium test and blood, peritoneal dialysate, and parietal peritoneum were collected.
Assessment of the peritoneal transport function by peritoneal equilibration test (PET)
PET was performed to assess the peritoneal permeability in rats as previously described [Citation34]. PDF containing 2.5% PDF was injected into the rat peritoneal cavity at a volume of 20 mL. Dialysate and blood samples (1 mL) were collected immediately (0 h) and at 2 h after infusion. The ultrafiltration volume (UV) and peritoneal solute transport were calculated as previously described [Citation34].
Histological and immunohistochemical examination
Hematoxylin and eosin (H&E) and Masson’s trichrome were used to observe peritoneal thickness and the collagen-positive area. Peritoneal thickness was defined as previously described [Citation35]. After routine deparaffinization, the slices were treated with 3% H2O2 to eliminate endogenous peroxidase activity and followed by antigen retrieval using proteinase K. The slides were blocked with 10% donkey serum. Primary antibodies against α-SMA (Abcam, ab5694), CD31 (Servicebio, GB11063-2), Collagen-I (Col-I) (Abcam, ab260043) and TGFβ1 (Abcam, ab215715) were added to the slides at a dilution of 1:100 and incubated overnight at 4 °C. At least 5 fields of view were examined for each slide using a microscope (Zeiss Axio Scope A1, Germany). Image J 1.8.0 was used to analysis and the results were expressed as the average of 5 independent measurements per slide.
Primary rat peritoneal mesothelial cell (PMC) culture and grouping
As previously described, PMCs were isolated from rat peritoneum using enzymatic digestion. Cells were incubated in the M199 culture medium (Gibco,11150059) supplemented with 10% fetal bovine serum (Gibco,10099141C). The cells were divided into four groups as follows: the control group (cells cultured in complete medium), CAPE group (complete medium containing 4 μM CAPE), PDF group (2.5% PDF: complete medium [V/V] = 1:1), and PDF + CAPE group (addition of 4 μM CAPE to the PDF group). Compound C (5 μM), an AMPK inhibitor, was used to inhibit AMPK pharmacologically.
Western blot analysis
Total proteins were extracted from PMCs. A total of 20 μg protein was separated by SDS–PAGE. The following primary antibodies were used: α-SMA, E-cadherin and TGFβ1 (Abcam, ab215715), AMPK (Cell Signaling Technology, 5831 T), p-AMPK (Cell Signaling Technology, 2535 T) and SIRT1 (Cell Signaling Technology, 9475 T), (all diluted 1:1000). The expression levels of the target proteins were analyzed using Image J 1.8.0 software.
Cell viability determination
The CCK-8 assay kit (Meilunbio) was utilized to assess cell viability. In brief, cells were cultured under the specified conditions as follows. In each well, 10 microliters of CCK-8 solution were added, and the cells were then incubated at a temperature of 37 °C for a duration of 2 h. Using a multifunctional microplate reader (TECAN Infinite 200 Pro, Switzerland), the measurement of absorbance was taken at 450 nm.
MDA, SOD activity and GSH detection
MDA levels and SOD activity in peritoneal tissue and cells were measured using the MDA assay kit (Beyotime, S0131) and the SOD activity assay kit (Beyotime, S0101S) separately, following the manufacturer’s instructions. The levels of glutathione (GSH) in the peritoneal tissues and cells were evaluated using the GSH assay kit (Sigma-Aldrich, MAK440), according to the instructions manual.
Adenosine triphosphate (ATP) assay
According to the manufacturer’s instructions, the ATP in the omentum tissue and PMCs was measured by utilizing the enhanced ATP assay kit (Beyotime, S0027). The relative light units (RLU) were measured using a multifunctional microplate reader (TECAN Infinite 200 Pro, Switzerland).
ROS detection
The total ROS level in the cells was assessed by employing the fluorescent probe DCFH-DA (Beyotime, S0033). Cells were incubated in a light-protected 37 °C 5% CO2 incubator for 20 min after adding a final concentration of 10 μmol/L DCFH-DA. After washing the cells, the fluorescence intensity was measured using a flow cytometer (FCM, NovoCyte 2060 R). We detected the generation of mitochondrial ROS by utilizing the MitoSOX Green probe (Invitrogen, M36005). The images were acquired with a Zeiss LSM (Zeiss LSM, Germany).
Mitochondrial membrane potential detection
The JC-1 probe (Beyotime, C2003S) was utilized to assess the mitochondrial membrane potential (MMP). Following particular interventions, the cells were rinsed once with PBS. Next, cell culture medium and JC-1 staining working solution were introduced (1:1). 20 min later, the liquid above was removed, and the cells were rinsed two times using JC-1 staining solution. Next, culture medium was introduced, and the cells were examined using a confocal laser scanning microscope (Zeiss LSM, Germany). The software ImageJ 1.8.0 was used to analyze the intensity of fluorescence.
Transmission electron microscopy
Cell aggregates were immersed in 2.5% glutaraldehyde and fixed at 4 °C followed by washing with PBS. Subsequently, samples were dehydrated in alcohol solutions and then embedded in resin and kept overnight at 37 °C. Then, the resin blocks were sliced into 60–80 nm sections using an ultramicrotome, stained on 150-mesh copper grids. Finally, the mitochondria were observed under a transmission electron microscope.
Transient transfection
According to the manufacturer’s protocol, siRNA was transfected into peritoneal mesothelial cells using LipofectamineTM 2000 (Invitrogen, 11668019). Briefly, 100 pmol of siRNA was diluted in 250 µl of Opti-MEM (Gibco, 11058021). Then, 5 µl of Lipofectamine™2000 was diluted in 250 µl of Opti-MEM and incubated at room temperature for 5 min. After 5 min, the diluted oligomer was mixed with diluted Lipofectamine™2000. After a 20-min incubation, the complexes were added to each well to replace the culture medium.
Statistical analysis
The quantitative data are presented as the mean ± standard deviation (SD). Multiple group comparison was done using one-way analysis of variance followed by the Bonferroni test. GraphPad Prism 8 software was used for the data analysis.
Results
Establishment of the peritoneal fibrosis model
A peritoneal fibrosis model was established by intraperitoneal injection of PDF and LPS. To observe the progression of fibrosis, we obtained the parietal peritoneum on days 0, 7, 14, and 28 after drug administration. Peritoneal pathological changes were evaluated using H&E and Masson’s staining. As shown in , on day 0, the rat peritoneum had a smooth surface with a layer of flat PMCs, and the submesothelial matrix was thin. On day 7, there was submesothelial matrix edema and increased infiltration of inflammatory cells. From day 14 to 28, in addition to infiltrating inflammatory cells, collagen deposition became more severe in the submesothelial layer.
Figure 1. Establishment of PD-induced peritoneal fibrosis in vivo. Rats were euthanized on days 0, 7, 14, and 28, and peritoneal tissues were collected. (A) The parietal peritoneal tissues were assessed by H&E and Masson’s staining. Magnification, ×400. (B) The bar graphs represent the thickness of the peritoneum and Masson-positive areas at different time points. Data were expressed as the mean ± SD. ns, no significance, *p < 0.05, ***p < 0.001.
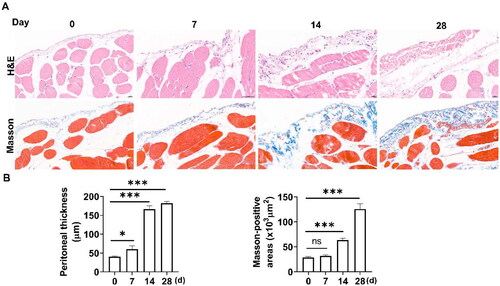
CAPE alleviates PD-induced peritoneal fibrosis
First, we observed the peritoneal changes in different groups using H&E and Masson’s staining. As shown in , the peritoneum of the PD rats exhibited thickening and significant collagen deposition. However, the addition of CAPE to the PDF significantly alleviated the peritoneal damage in PD rats including alleviated peritoneal thickening, decreased collagen fiber deposition and reduced inflammatory cell infiltration. Additionally, immunohistochemical staining of CD 31 in peritoneum indicated that CAPE decreased neovascularization (). Next, we evaluated the peritoneal transport function using PET. As expected, compared to the control rats, the PD rats showed a significant decrease in UV, which was accompanied by an increased D2h creatinine/P2h creatinine ratio and a decreased D2h glucose/D0h glucose ratio, indicating impaired peritoneal ultrafiltration function and increased peritoneal permeability. In comparison, the addition of CAPE to PDF reversed the impairment of the ultrafiltration function and the increased peritoneal permeability induced by PD (). These results suggest that the addition of CAPE to PDF alleviates peritoneal damage in PD rats.
Figure 2. CAPE alleviates PD-induced peritoneal fibrosis. (A) The structural changes of the rat peritoneum in different groups were observed using H&E and Masson’s staining. Magnification, ×400. (B) The bar graphs represent the thickness of the peritoneum and Masson-positive areas. (C) Immunohistochemical staining of CD31 in the rat peritoneum of different groups. Magnification, ×400. (D) The bar graph represents CD31-positive vessels in peritoneum. (E) The results of the peritoneal equilibration test. Data were expressed as the mean ± SD. ns, no significance, **p < 0.01, ***p < 0.001.
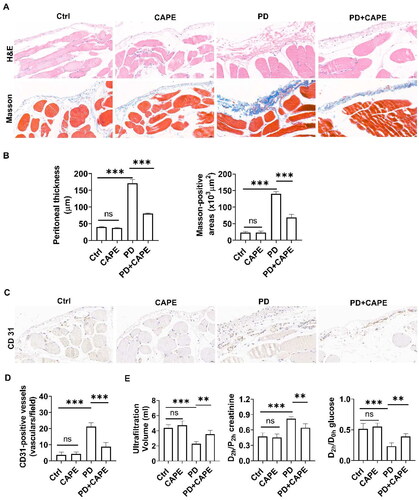
CAPE alleviates PD-induced MMT and oxidative stress in vivo and in vitro
TGF β1 is an important factor mediating MMT. α-SMA is a marker of myofibroblasts, and collagen-I (Col-I) is a major component of the extracellular matrix. Therefore, we evaluated the expression of TGF β1, α-SMA, and Col-I using immunohistochemistry. As shown in , compared to control rats, TGF β1- and α-SMA-positive cells of PD rats significantly increased. However, the addition of CAPE to PDF reduced the number of TGF-β1- and α-SMA-positive cells. Additionally, Col-I in the submesothelial layer was significantly increased in PD rats, while CAPE significantly reduced the expression of Col-I.
Figure 3. CAPE alleviates PD-induced MMT and oxidative stress in vivo and in vitro. (A) Immunohistochemical staining of α-SMA, TGF β1, and Col-I in the rat peritoneum of different groups. Magnification, ×400. (B) The bar graphs represent α-SMA-, TGF β1-positive cells and Col-I-positive areas in peritoneum. (C) Cell viability was determined using the CCK-8 assay kit, and the results showed that CAPE exhibited cytotoxicity on PMCs when the concentration reached above 8 μM. (D) After pretreatment with 4 μM CAPE, cells were stimulated with 2.5% PDF for 24 h, and the protein levels of E-cadherin, α-SMA, and TGF β1 in PMCs from different groups were detected. (E-G) MDA, GSH and SOD levels in the peritoneal tissue of each group of rats. (H-J) Intracellular ROS, GSH and SOD levels from different groups. ns, no significance, *p < 0.05, **p < 0.01, ***p < 0.001.
Next, we attempted to examine the impact of CAPE on the PDF-induced MMT of PMCs in vitro. We first examined whether CAPE exerted cytotoxicity on PMCs under normal culture conditions. As shown in , CAPE exhibited cytotoxicity on PMCs when the concentration reached above 8 μM. Therefore, we chose a concentration of 4 μM CAPE for further experiments. As shown in , PDF upregulated TGF β1 and α-SMA and downregulated E-cadherin. CAPE effectively reversed the PDF-induced upregulation of TGF β1 and α-SMA and the downregulation of E-cadherin. The findings suggest that CAPE inhibits PDF-induced MMT of PMCs in vitro.
Oxidative stress is a significant catalyst of MMT and leads to peritoneal fibrosis [Citation36]. The impact of CAPE on oxidative stress in the peritoneum was assessed during PD conditions. As shown in , compared to the control rats, the PD rats exhibited a significant increase in MDA levels and a decrease in GSH levels and SOD activity in the peritoneum. The addition of CAPE to PDF partially reversed these changes. In vitro, PDF promoted ROS generation and decreased GSH levels and SOD activity. Treatment with CAPE significantly inhibited ROS generation and increased GSH levels and SOD activity (). The findings indicate that the addition of CAPE to PDF alleviates oxidative stress in peritoneal tissues under PD conditions.
CAPE rescues mitochondrial homeostasis in the PD state
We further investigated the mechanism by which CAPE improves oxidative stress. Mitochondria are the main sources of ROS. We found that PDF increased mitochondrial ROS, while CAPE significantly reversed this change (). Significant alterations in mitochondrial structure were observed in PDF-stimulated PMCs after 12 h, as evidenced by transmission electron microscopy. These changes included shortened length, mitochondrial swelling, and a loss of cristae, while CAPE partially restored mitochondrial morphology (). In addition, the stimulation of PMCs with PDF resulted in a decreased ATP, while treatment with 4 µM CAPE restored ATP levels (). Consistent with the results obtained in vitro, compared to the control rats, PD rats exhibited a significant decreased ATP in the omentum, while CAPE alleviated the inhibition of ATP ().
Figure 4. CAPE rescues mitochondrial homeostasis in the PD state. (A) Confocal microscopic images revealed increased mitochondrial ROS in PDF-induced PMCs, which was significantly reversed by CAPE. Magnification, ×200. (B) Transmission electron microscopy images of mitochondria in PMCs, with a scale bar of 500 nm, indicate the disappearance of mitochondrial cristae (arrow) and mitochondrial swelling and cristae reduction (star). (C) ATP levels in PMCs in the indicated groups. (D) ATP levels in the omentum. *p < 0.05, **p < 0.01, ***p < 0.001.
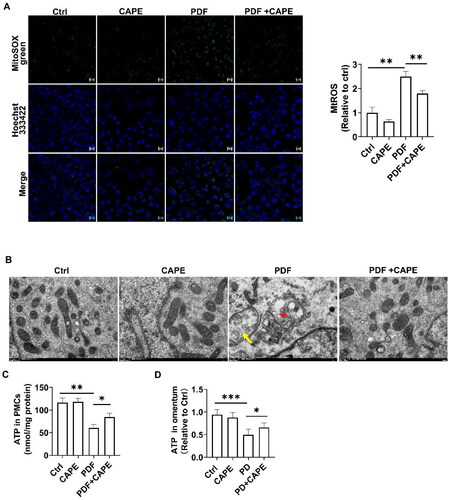
CAPE activates the AMPK/SIRT1 pathway in PDF-treated PMCs
The AMPK/SIRT1 pathway is vital for regulating intracellular signals, which are essential for maintaining mitochondrial homeostasis [Citation37]. Consequently, we explored the impact of CAPE on the AMPK/SIRT1 pathway. Immunoblotting results showed that exposure of PMCs to PDF for 12 h resulted in a decrease in AMPK phosphorylation and SIRT1 expression (). CAPE reversed the decrease in AMPK phosphorylation but SIRT1 expression (). PMCs exposed to PDF for 24 h still significantly inhibited AMPK phosphorylation and SIRT1 expression (). At this point, CAPE reversed the decrease in AMPK phosphorylation and the downregulation of SIRT1 in PDF-treated PMCs (). The findings indicate that CAPE could potentially enhance mitochondrial homeostasis through the AMPK/SIRT1 pathway.
Figure 5. CAPE activates the AMPK/SIRT1 pathway in PDF-treated PMCs. (A) Immunoblotting results showed that exposure of PMCs to PDF for 12 h resulted in a decrease in AMPK phosphorylation and SIRT1 expression. CAPE reversed the decrease in AMPK phosphorylation but SIRT1 expression. (B) PMCs exposed to PDF for 24 h still significantly inhibited AMPK phosphorylation and SIRT1 expression. At this point, CAPE reversed the decrease in AMPK phosphorylation and the downregulation of SIRT1 in PDF-treated PMCs. *p < 0.05, **p < 0.01, ***p < 0.001.
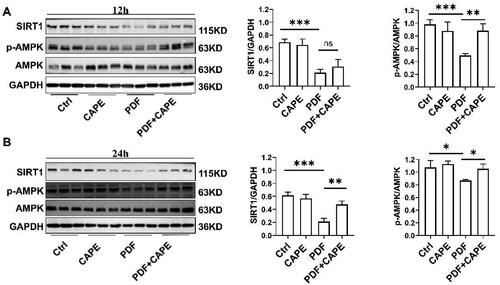
CAPE maintains mitochondrial homeostasis and alleviates MMT via the AMPK/SIRT1 pathway
We validated whether the AMPK/SIRT1 pathway is involved in the beneficial effects mediated by CAPE using an AMPK inhibitor (Compound C) and siRNA targeting SIRT1. The findings indicated that positive impacts of CAPE on restoring MMP () and reducing mitochondrial ROS production () were neutralized by Compound C or the SIRT1 knockdown. Further exploration revealed that the pharmacological inhibition of AMPK or the knockdown of SIRT1 partially abolished the beneficial effects of CAPE on reducing total intracellular ROS production () and meanwhile neutralized the effects of CAPE on restoring GSH, SOD and ATP synthesis (). CAPE effectively reversed the PDF-induced upregulation of TGF β1 and α-SMA and the downregulation of E-cadherin (). The beneficial effect of CAPE on MMT were partially abolished by Compound C or the SIRT1 knockdown (). These results indicate that CAPE maintains mitochondrial homeostasis by activating the AMPK/SIRT1 pathway, and thereby inhibit MMT of PMCs.
Figure 6. CAPE maintains mitochondrial homeostasis via the AMPK/SIRT1 pathway. The beneficial effects of CAPE on restoring MMP (A) and reducing mitochondrial ROS (B) were neutralized by Compound C or the SIRT1 knockdown. Magnification, ×400. (C) The beneficial effect of CAPE on inhibiting total intracellular ROS depended on AMPK and SIRT1. (D) GSH levels in PMCs in the indicated groups. (E) GSH levels in PMCs in the indicated groups. (F) ATP levels in PMCs in the indicated groups. (G) The protein levels of E-cadherin, α-SMA, and TGF β1 in PMCs from different groups were detected. *p < 0.05, **p < 0.01, ***p < 0.001.
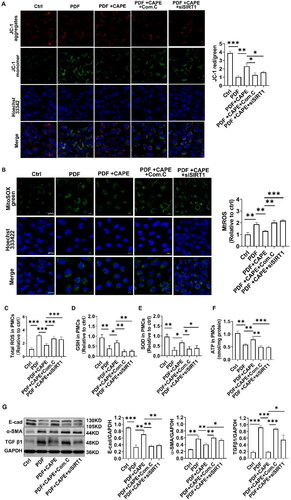
Discussion
In this study, PD rats exhibited peritoneal thickening accompanied by increased vascularization and collagen deposition. The addition of CAPE to PDF significantly inhibited PD-induced MMT, alleviated peritoneal fibrosis, and improved the ultrafiltration function. In vitro, PDF downregulated the AMPK/SIRT1 pathway, increased oxidative stress, and induced MMT of PMCs. CAPE reversed the inhibition of AMPK phosphorylation, activated the AMPK/SIRT1 pathway, inhibited mitochondrial membrane depolarization, restored ATP synthesis and reduced mitochondrial ROS generation. The effects of CAPE were neutralized by an AMPK inhibitor and siSIRT1. Our findings suggest that CAPE maintains mitochondrial homeostasis by upregulating the AMPK/SIRT1 pathway, alleviating oxidative stress, inhibiting MMT, and thereby mitigating the damage to peritoneal structure and function induced by PD.
PMCs play a critical role in the integrity of the peritoneal structure and function as the first line of defense against PDF exposure. Previous studies have found that long-term PD can increase PMC apoptosis and induce MMT [Citation36,Citation38,Citation39]. In this study, we found that the peritoneum of the model rats exhibited thickening and increased collagen deposition with significant upregulation of TGFβ1, α-SMA, and Col-I in the peritoneum. Similar results were observed in PMCs cultured in vitro with upregulation of the fibroblast markers TGFβ1 and α-SMA and downregulation of the epithelial marker E-cadherin. Therefore, consistent with previous studies [Citation36,Citation40], our results confirm that PD can induce MMT, which leads to peritoneal fibrosis.
Increasing studies have found that oxidative stress is an important event in PD-related peritoneal fibrosis [Citation36,Citation40–42]. Glucose is an effective osmotic agent that is widely used in PD due to its low cost and easy metabolism. For example, in the 2.5% glucose-based PDF, the glucose concentration is 138 mmol/L. Cells exposed to a high glucose environment release a large amount of ROS [Citation36,Citation41]. Our previous research has shown that high glucose can cause oxidative stress damage to PMCs and induce apoptosis and MMT, which leads to peritoneal fibrosis and ultrafiltration failure [Citation36]. In this study, we found that MDA in the peritoneum of PD rats was significantly increased, while GSH and SOD activity was decreased. Consistent with the in vivo study, PDF promoted ROS generation and reduced GSH and SOD activity in cultured PMCs.
Caffeic acid phenethyl ester (CAPE) is the main active component of propolis and has been shown to possess effective antioxidant and antifibrosis properties [Citation26,Citation27]. CAPE inhibits liver fibrosis in rats by suppressing the TGF-β1/Smad3 and NF-κB pathways, while inducing the Nrf2 and autophagy pathways [Citation43–45]. Additionally, CAPE alleviates airway remodeling in chronic asthma through modulation of the ROS-responsive MAPK/Akt pathway and the NF-κB/TGF-β1 pathway [Citation46,Citation47]. There is also evidence to suggest that CAPE mitigates cardiac hypertrophy by regulating the MEK/ERK and TGF-β1/Galectin-3 pathways [Citation48,Citation49]. In terms of attenuating renal fibrosis, studies have shown that KS370G, a synthetic CAPE derivative, reduces obstructive nephropathy [Citation50], and mitigates renal ischemia-reperfusion injury by inhibiting the TGF-β/Smad3 pathway. Another derivative of CAPE, CAPE-pNO2, ameliorates diabetic nephropathy by suppressing oxidative stress, inflammation, and fibrosis via the Akt/NF-κB/iNOS pathway [Citation51]. According to previous research, in vivo, the maximum single dose of CAPE administered via intraperitoneal injection can reach up to 34 mg/kg [Citation27], while multiple dosing regimens range from 10 μmol/kg*d (equivalent to 2.84 mg/kg*d) to 10 mg/kg*d [Citation51–53]. In our preliminary research, CAPE was administered orally by gavage at a daily dose of 10 mg/kg. Despite the significant alleviation in peritoneal fibrosis [Citation40], the rats receiving CAPE suffered reduced food intake and weight loss. In this study, CAPE was added into the PDF and administered it via intraperitoneal injection, significantly reducing the effective dose. We found that CAPE (4 mg/kg) added to the PDF was effective in alleviating peritoneal fibrosis. Further research revealed that CAPE significantly reduced oxidative stress and inhibited MMT under PD conditions in vivo and in vitro. Mitochondria serve as the primary sources of ROS and are also the target of these molecules [Citation54]. Damaged mitochondria release mitochondrial ROS, triggering inflammation and immune responses, which in turn leads to mitochondrial damage [Citation55]. Under PD conditions, glucose-rich PDF causes mitochondrial dysfunction in human PMCs, resulting in increased mitochondrial ROS generation, cell apoptosis, and mtDNA damage [Citation17,Citation56]. In this study, we found that PDF induced mitochondrial morphological changes, depolarization of the MMP, increased mitochondrial ROS production, and decreased ATP synthesis in PMCs. Consistent with previous studies, our research also confirmed that mitochondrial dysfunction contributed to PD-induced peritoneal damage. We also demonstrated that the addition of CAPE to the PDF can reverse the above events. However, the mechanisms by which CAPE maintains mitochondrial function remain unclear.
Therefore, we proceeded to explore the mechanisms of the safeguarding impact of CAPE on mitochondria. AMP-activated protein kinase (AMPK) is a conserved serine/threonine kinase that regulates energy homeostasis and is responsible for monitoring cellular energy input and output [Citation57,Citation58]. It has been demonstrated that impaired AMPK is associated with metabolic diseases [Citation59]. Additionally, AMPK is a key protein involved in multiple signaling pathways. Phosphorylated AMPK activates silent mating-type information regulation 2 homolog 1 (SIRT1), which plays a crucial regulatory role in mitochondrial homeostasis and oxidative stress [Citation60–63]. Previous studies have shown that CAPE has a protective effect on various neurological disorders by activating the AMPK/SIRT1 pathway. For example, it reverses cell death and subsequent cognitive impairment in the hippocampus and cortex of mice [Citation64], protects PC12 (neuroendocrine) cells from cisplatin-induced neurotoxicity [Citation28], accelerates functional recovery in mice with traumatic brain injury [Citation65], and alleviates neuroinflammation in microglial cells [Citation66]. In this study, we found that PDF inhibited AMPK phosphorylation, downregulated SIRT1, induced mitochondrial membrane potential depolarization and mitochondrial ROS generation, and suppressed ATP synthesis. This suggests that PDF downregulates the AMPK/SIRT1 pathway, disrupting mitochondrial homeostasis and energy metabolism. However, adding CAPE to the PDF activates the AMPK/SIRT1 pathway and restores mitochondrial membrane potential and energy metabolism. The protective effect of CAPE on mitochondrial homeostasis was abolished by an AMPK inhibitor or silencing SIRT1 with siRNA, which also abolished the alleviation of oxidative stress. Therefore, we speculate that the protective effect of CAPE on the peritoneum partially depends on activating the AMPK/SIRT1 pathway and thereby exerts beneficial effects on maintaining mitochondrial homeostasis.
In summary, our study is the first to demonstrate that adding CAPE to the PDF regulates mitochondrial homeostasis by activating the AMPK/SIRT1 pathway, thereby alleviating PD-induced oxidative stress, reversing the process of MMT, and ultimately ameliorating peritoneal fibrosis caused by PD (). This study provides a theoretical basis for the application of CAPE in the prevention and treatment of PD-related peritoneal fibrosis.
Figure 7. Schematic diagram depicting the possible molecular mechanisms by which CAPE prevents peritoneal fibrosis by maintaining mitochondrial integrity under PD conditions. Under PD conditions, AMPK/SIRT1 pathway are downregulated, which disturbs mitochondrial homeostasis and then leads to oxidative stress. This upregulated oxidative stress results in MMT, which eventually leads to peritoneal fibrosis. Interestingly, CAPE treatment activates the AMPK/SIRT1 pathway and restores mitochondrial homeostasis, which alleviating oxidative stress, thereby attenuating PD-induced MMT and peritoneal fibrosis.
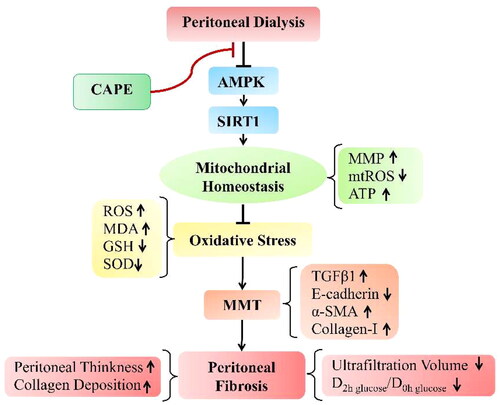
Author contributions
KS and JH performed study concept and design; YL and LG performed development of methodology and writing; YL, LG, WZ and YZ provided acquisition, analysis and interpretation of data, and statistical analysis; KS and JH provided technical and material support. All authors read and approved the final paper.
Acknowledgements
The authors wish to thank Ye Zhu and Lingling Liu for assistance in pathological evaluation.
Disclosure statement
No potential conflict of interest was reported by the author(s).
Additional information
Funding
References
- Javaid M, Khan B, Subramanian S. Peritoneal dialysis as initial dialysis modality: a viable option for late-presenting end-stage renal disease. J Nephrol. 2019;32(1):51–56. doi:10.1007/s40620-018-0485-3.
- Yeates K, Zhu N, Vonesh E, et al. Hemodialysis and peritoneal dialysis are associated with similar outcomes for end-stage renal disease treatment in Canada. Nephrol Dial Transplant. 2012;27(9):3568–3575. doi:10.1093/ndt/gfr674.
- del Peso G, Jiménez-Heffernan J, Selgas R, et al. Biocompatible dialysis solutions preserve peritoneal mesothelial cell and vessel wall integrity. A case-control study on human biopsies. Perit Dial Int. 2016;36(2):129–134. doi:10.3747/pdi.2014.00038.
- Zhou Q, Bajo M, Del Peso G, et al. Preventing peritoneal membrane fibrosis in peritoneal dialysis patients. Kidney Int. 2016;90(3):515–524. doi:10.1016/j.kint.2016.03.040.
- Mateijsen M, van der Wal A, Hendriks P, et al. Vascular and interstitial changes in the peritoneum of CAPD patients with peritoneal sclerosis. Perit Dial Int. 1999;19(6):517–525. doi:10.1177/089686089901900605.
- Krediet R, Struijk D. Peritoneal changes in patients on long-term peritoneal dialysis. Nat Rev Nephrol. 2013;9(7):419–429. doi:10.1038/nrneph.2013.99.
- Williams J, Craig K, Topley N, et al. Morphologic changes in the peritoneal membrane of patients with renal disease. J Am Soc Nephrol. 2002;13(2):470–479. doi:10.1681/asn.V132470.
- Devuyst O, Margetts P, Topley N. The pathophysiology of the peritoneal membrane. J Am Soc Nephrol. 2010;21(7):1077–1085. doi:10.1681/asn.2009070694.
- Yáñez-Mó M, Lara-Pezzi E, Selgas R, et al. Peritoneal dialysis and epithelial-to-mesenchymal transition of mesothelial cells. N Engl J Med. 2003;348(5):403–413. doi:10.1056/NEJMoa020809.
- Aroeira L, Aguilera A, Sánchez-Tomero J, et al. Epithelial to mesenchymal transition and peritoneal membrane failure in peritoneal dialysis patients: pathologic significance and potential therapeutic interventions. J Am Soc Nephrol. 2007;18(7):2004–2013. doi:10.1681/asn.2006111292.
- Fawzy M, Beshay O, Bekhit A, et al. Nephroprotective effect of at-MSCs against cisplatin-induced EMT is improved by azilsartan via attenuating oxidative stress and TGF-β/Smad signaling. Biomed Pharmacother. 2023;158:114097. doi:10.1016/j.biopha.2022.114097.
- Kong D, Zhang Z, Chen L, et al. Curcumin blunts epithelial-mesenchymal transition of hepatocytes to alleviate hepatic fibrosis through regulating oxidative stress and autophagy. Redox Biol. 2020;36:101600. doi:10.1016/j.redox.2020.101600.
- Bai Y, Wang W, Yin P, et al. Ruxolitinib alleviates renal interstitial fibrosis in UUO mice. Int J Biol Sci. 2020;16(2):194–203. doi:10.7150/ijbs.39024.
- Kang M, Kim S, Oh S, et al. Tangeretin ameliorates glucose-induced podocyte injury through blocking epithelial to mesenchymal transition caused by oxidative stress and hypoxia. Int J Mol Sci. 2020;21(22):8577. doi:10.3390/ijms21228577.
- Hyttinen J, Kannan R, Felszeghy S, et al. The regulation of NFE2L2 (NRF2) signalling and epithelial-to-Mesenchymal transition in Age-Related macular degeneration pathology. Int J Mol Sci. 2019;20(22):5800. doi:10.3390/ijms20225800.
- Santamaría B, Ucero A, Benito-Martin A, et al. Biocompatibility reduces inflammation-induced apoptosis in mesothelial cells exposed to peritoneal dialysis fluid. Blood Purif. 2015;39(1–3):200–209. doi:10.1159/000374103.
- Hung K, Liu S, Yang T, et al. High-dialysate-glucose-induced oxidative stress and mitochondrial-mediated apoptosis in human peritoneal mesothelial cells. Oxid Med Cell Longev. 2014;2014:642793–642712. doi:10.1155/2014/642793.
- Braga P, Alves M, Rodrigues A, et al. Mitochondrial pathophysiology on chronic kidney disease. Int J Mol Sci. 2022;23(3):1776. doi:10.3390/ijms23031776.
- Liu S, Zhao M, Zhou Y, et al. Resveratrol exerts dose-dependent anti-fibrotic or pro-fibrotic effects in kidneys: a potential risk to individuals with impaired kidney function. Phytomedicine. 2019;98:153855–153235. doi:10.1016/j.phymed.2018.12.024.
- Zhang S, Tan X, Chen Y, et al. Postconditioning protects renal fibrosis by attenuating oxidative stress-induced mitochondrial injury. Nephrol Dial Transplant. 2017;32(10):1628–1636. doi:10.1093/ndt/gfw469.
- Li Y, Du Z, Li T, et al. MitoQ ameliorates PM-induced pulmonary fibrosis through regulating the mitochondria DNA homeostasis. Chemosphere. 2023;330:138745. doi:10.1016/j.chemosphere.2023.138745.
- Ramil-Gómez O, Rodríguez-Carmona A, Fernández-Rodríguez J, et al. Mitochondrial dysfunction plays a relevant role in pathophysiology of peritoneal membrane damage induced by peritoneal dialysis. Antioxidants . 2021;10(3):447. doi:10.3390/antiox10030447.
- Lu H, Chen W, Liu W, et al. Molecular hydrogen regulates PTEN-AKT-mTOR signaling via ROS to alleviate peritoneal dialysis-related peritoneal fibrosis. Faseb J. 2020;34(3):4134–4146. doi:10.1096/fj.201901981R.
- Terawaki H, Hayashi Y, Zhu W, et al. Transperitoneal administration of dissolved hydrogen for peritoneal dialysis patients: a novel approach to suppress oxidative stress in the peritoneal cavity. Med Gas Res. 2013;3(1):14. doi:10.1186/2045-9912-3-14.
- Ohsawa I, Ishikawa M, Takahashi K, et al. Hydrogen acts as a therapeutic antioxidant by selectively reducing cytotoxic oxygen radicals. Nat Med. 2007;13(6):688–694. J doi:10.1038/nm1577.
- Göçer H, Gülçin I. Caffeic acid phenethyl ester (CAPE): correlation of structure and antioxidant properties[J]. Int J Food Sci Nutr. 2011;62(8):821–825. doi:10.3109/09637486.2011.585963.
- Trumbeckaite S, Pauziene N, Trumbeckas D, et al. Caffeic acid phenethyl ester reduces Ischemia-induced kidney mitochondrial injury in rats. Oxid Med Cell Longev. 2017;2017:1697018–1697011. doi:10.1155/2017/1697018.
- Ferreira R, Dos Santos N, Bernardes C, et al. Caffeic acid phenethyl ester (CAPE) protects PC12 cells against Cisplatin-induced neurotoxicity by activating the AMPK/SIRT1, MAPK/Erk, and PI3k/Akt signaling pathways. Neurotox Res. 2019;36(1):175–192. J doi:10.1007/s12640-019-00042-w.
- Huang Y, Jin M, Pi R, et al. Protective effects of caffeic acid and caffeic acid phenethyl ester against acrolein-induced neurotoxicity in HT22 mouse hippocampal cells. Neurosci Lett. 2013;535:146–151. doi:10.1016/j.neulet.2012.12.051.
- Tsuda S, Egawa T, Ma X, et al. Coffee polyphenol caffeic acid but not chlorogenic acid increases 5'AMP-activated protein kinase and insulin-independent glucose transport in rat skeletal muscle. J Nutr Biochem. 2012;23(11):1403–1409. doi:10.1016/j.jnutbio.2011.09.001.
- Liao C, Ou T, Huang H, et al. The inhibition of oleic acid induced hepatic lipogenesis and the promotion of lipolysis by caffeic acid via up-regulation of AMP-activated kinase. J Sci Food Agric. 2014;94(6):1154–1162. doi:10.1002/jsfa.6386.
- Singh J, Khan M, Singh I. Caffeic acid phenethyl ester induces adrenoleukodystrophy (Abcd2) gene in human X-ALD fibroblasts and inhibits the proinflammatory response in Abcd1/2 silenced mouse primary astrocytes. Biochim Biophys Acta. 2013;1831(4):747–758. doi:10.1016/j.bbalip.2013.01.004.
- Xu T, Xie JY, Wang WM, et al. Impact of rapamycin on peritoneal fibrosis and transport function. Blood Purif. 2012;34(1):48–57. doi:10.1159/000339814.
- Ni J, Verbavatz J, Rippe A, et al. Aquaporin-1 plays an essential role in water permeability and ultrafiltration during peritoneal dialysis. Kidney Int. 2006;69(9):1518–1525. doi:10.1038/sj.ki.5000285.
- Musi B, Braide M, Carlsson O, et al. Biocompatibility of peritoneal dialysis fluids: long-term exposure of nonuremic rats. Perit Dial Int. 2004;24(1):37–47.
- Shi P, Zhan Z, Ye X, et al. The antioxidative effects of empagliflozin on high glucose‑induced epithelial-mesenchymal transition in peritoneal mesothelial cells via the Nrf2/HO-1 signaling. Ren Fail. 2022;44(1):1528–1542. doi:10.1080/0886022x.2022.2118066.
- Ou H, Chu P, Huang Y, et al. Low-level laser prevents doxorubicin-induced skeletal muscle atrophy by modulating AMPK/SIRT1/PCG-1α-mediated mitochondrial function, apoptosis and up-regulation of pro-inflammatory responses. Cell Biosci. 2021;11(1):200. doi:10.1186/s13578-021-00719-w.
- Xie S, Xu F, Lu Y, et al. Elabela attenuates the TGF-β1-induced epithelial-mesenchymal transition of peritoneal mesothelial cells in patients receiving peritoneal dialysis. Front Pharmacol. 2022;13:890881. doi:10.3389/fphar.2022.890881.
- Lee Y, Lee J, Park M, et al. Inflammatory chemokine (C-C motif) ligand 8 inhibition ameliorates peritoneal fibrosis. Faseb J. 2023;37(1):e22632. doi:10.1096/fj.202200784R.
- Lu Ying SH-Y, Lu-Yan G, Zhi W, et al. Caffeic acid phenethyl ester improves peritoneal dialysis-associated peritoneal fibrosis by alleviating oxidative stress injury through activating nuclear factor erythroid-2-related factor 2/heme oxygenase-1 pathway. Chin J Nephrol. 2023;39(6):446–455.
- Lu Y, Shen H, Shi X, et al. Hydrogen sulfide ameliorates high-glucose toxicity in rat peritoneal mesothelial cells by attenuating oxidative stress. Nephron Exp Nephrol. 2014;126(3):157–165. doi:10.1159/000358436.
- Roumeliotis S, Dounousi E, Salmas M, et al. Unfavorable effects of peritoneal dialysis solutions on the peritoneal membrane: the role of oxidative stress. Biomolecules. 2020;10(5):768. doi:10.3390/biom10050768.
- Yang N, Shi J, Wu F, et al. viaCaffeic acid phenethyl ester up-regulates antioxidant levels in hepatic stellate cell line T6 an Nrf2-mediated mitogen activated protein kinases pathway. World J Gastroenterol. 2017;23(7):1203–1214. doi:10.3748/wjg.v23.i7.1203.
- Li M, Wang X, Shi J, et al. Caffeic acid phenethyl ester inhibits liver fibrosis in rats. World J Gastroenterol. 2015;21(13):3893–3903. doi:10.3748/wjg.v21.i13.3893.
- Yang N, Dang S, Shi J, et al. Caffeic acid phenethyl ester attenuates liver fibrosis via inhibition of TGF-β1/Smad3 pathway and induction of autophagy pathway. Biochem Biophys Res Commun. 2017;486(1):22–28. doi:10.1016/j.bbrc.2017.02.057.
- Ma Y, Zhang J, Liu Y, et al. Caffeic acid phenethyl ester alleviates asthma by regulating the airway microenvironment via the ROS-responsive MAPK/Akt pathway. Free Radic Biol Med. 2016;101:163–175. doi:10.1016/j.freeradbiomed.2016.09.012.
- Chen P, Wang X, Li Y, et al. An inhibitor of nuclear Factor-Kappa B pathway attenuates the release of TGF-β1 and inhibits the fibrogenic progress in a model of airway remodeling induced by acrolein. Comput Math Methods Med. 2022;2022:4984634. doi:10.1155/2022/4984634.
- Ren J, Zhang N, Liao H, et al. Caffeic acid phenethyl ester attenuates pathological cardiac hypertrophy by regulation of MEK/ERK signaling pathway in vivo and vitro. Life Sci. 2017;181:53–61. doi:10.1016/j.lfs.2017.04.016.
- Wan Q, Zhang L, Zhou Q, et al. Protection of CAPE-pNO(2) against chronic myocardial ischemia by the TGF-B1/galectin-3 pathway in vivo and in vitro. Inflammation. 2022;45(3):1039–1058. doi:10.1007/s10753-021-01600-1.
- Chuang ST, Kuo YH, Su MJ. KS370G, a caffeamide derivative, attenuates unilateral ureteral obstruction-induced renal fibrosis by the reduction of inflammation and oxidative stress in mice. Eur J Pharmacol. 2015;750:1–7. doi:10.1016/j.ejphar.2015.01.020.
- Wang X, Li D, Fan L, et al. CAPE-pNO(2) ameliorated diabetic nephropathy through regulating the Akt/NF-κB/iNOS pathway in STZ-induced diabetic mice. Oncotarget. 2017;8(70):114506–114525. doi:10.18632/oncotarget.23016.
- Cheng C, Chi P, Shen M, et al. Caffeic acid phenethyl ester rescues pulmonary arterial hypertension through the inhibition of AKT/ERK-Dependent PDGF/HIF-1α in vitro and in vivo. Int J Mol Sci. 2019;20(6):1468. doi:10.3390/ijms20061468.
- Li S, Huang Q, Zhang L, et al. Effect of CAPE-pNO2 against type 2 diabetes mellitus via the AMPK/GLUT4/GSK3β/PPARα pathway in HFD/STZ-induced diabetic mice. Eur J Pharmacol. 2019;853:1–10. doi:10.1016/j.ejphar.2019.03.027.
- López-Armada M, Riveiro-Naveira R, Vaamonde-García C, et al. Mitochondrial dysfunction and the inflammatory response. Mitochondrion. 2013;13(2):106–118. doi:10.1016/j.mito.2013.01.003.
- Mills E, Kelly B, O'Neill L. Mitochondria are the powerhouses of immunity. Nat Immunol. 2017;18(5):488–498. doi:10.1038/ni.3704.
- Xie X, Wang J, Xiang S, et al. Dialysate cell-free mitochondrial DNA fragments as a marker of intraperitoneal inflammation and peritoneal solute transport rate in peritoneal dialysis. BMC Nephrol. 2019;20(1):128. doi:10.1186/s12882-019-1284-3.
- Rabinovitch R, Samborska B, Faubert B, et al. AMPK maintains cellular metabolic homeostasis through regulation of mitochondrial reactive oxygen species. Cell Rep. 2017;21(1):1–9. doi:10.1016/j.celrep.2017.09.026.
- Hardie D, Ross F, Hawley S. AMPK: a nutrient and energy sensor that maintains energy homeostasis. Nat Rev Mol Cell Biol. 2012;13(4):251–262. doi:10.1038/nrm3311.
- Lage R, Diéguez C, Vidal-Puig A, et al. AMPK: a metabolic gauge regulating whole-body energy homeostasis. Trends Mol Med. 2008;14(12):539–549. doi:10.1016/j.molmed.2008.09.007.
- Cantó C, Auwerx J. PGC-1alpha, SIRT1 and AMPK, an energy sensing network that controls energy expenditure. Curr Opin Lipidol. 2009;20(2):98–105. doi:10.1097/MOL.0b013e328328d0a4.
- Andris F, Leo O. AMPK in lymphocyte metabolism and function. Int Rev Immunol. 2015;34(1):67–81. J doi:10.3109/08830185.2014.969422.
- Chen X, Li X, Zhang W, et al. Activation of AMPK inhibits inflammatory response during hypoxia and reoxygenation through modulating JNK-mediated NF-κB pathway. Metabolism. 2018;83:256–270. doi:10.1016/j.metabol.2018.03.004.
- Teng L, Fan L, Peng Y, et al. Carnosic acid mitigates early brain injury after subarachnoid hemorrhage: possible involvement of the SIRT1/p66shc signaling pathway. Front Neurosci. 2019;13:26. doi:10.3389/fnins.2019.00026.
- Hao R, Song X, Li F, et al. Caffeic acid phenethyl ester reversed cadmium-induced cell death in hippocampus and cortex and subsequent cognitive disorders in mice: involvements of AMPK/SIRT1 pathway and amyloid-tau-neuroinflammation axis. Food Chem Toxicol. 2020;144:111636. doi:10.1016/j.fct.2020.111636.
- Khan M, Shunmugavel A, Dhammu T, et al. Combined treatment with GSNO and CAPE accelerates functional recovery via additive antioxidant activities in a mouse model of TBI. J Neurosci Res. 2018;96(12):1900–1913. doi:10.1002/jnr.24279.
- Tsai C, Kuo Y, Yeh W, et al. Regulatory effects of caffeic acid phenethyl ester on neuroinflammation in microglial cells. Int J Mol Sci. 2015;16(3):5572–5589. doi:10.3390/ijms16035572.