Abstract
Background
In order to better understand the interplay between ferroptosis and autophagy, enhance the interpretation of the crosstalk between these two forms of regulated cell death, develop the effective pharmacological mechanisms for cancer treatment, discover novel biomarkers for better diagnostic, and envisage the future hotspots of the research on ferroptosis and autophagy, we harnessed bibliometric tools to study the articles published from 2012 to 2022 on the relationship between ferroptosis and autophagy.
Methods
Web of Science Core Collection (WOSCC) database was used to conduct a comprehensive search and analysis of articles in this field from January 1, 2012, to September 1, 2022. The Citespace 6.1.R2 software and VOS viewer 6.1.8 software were utilized to analyze the overall structure of the network, network clusters, links between clusters, key nodes or pivot points, and pathways.
Results
A total of 756 articles associated with the crosstalk between ferroptosis and autophagy were published in 512 journals by 4183 authors in 980 organizations from 55 countries or regions. The distribution of countries and organizations was demonstrated using CiteSpace and VOS viewer. The top three countries with the most articles were China (n = 511), United States (n = 166), and Germany (n = 37). The most productive institutions were Guangzhou Medical University and Central South University (n = 42), but their centralities were relatively low, which values were respective 0.04 and 0.03. Kang and Tang published the most articles related to ferroptosis and autophagy (n = 49), followed by Jiao Liu (n = 22), Guido Kroemer (n = 20), and Daniel Klionsky (n = 12). Published studies on ferroptosis and asthma have the most cited counts. The top three keywords with the highest frequencies were autophagy (n = 283), cell death (n = 243), and oxidative stress (n = 165).
Conclusion
Our results provide insights into the development of recognition related to the crosstalk between ferroptosis and autophagy, and the current molecular crosslinked mechanisms in the context of common signal transduction pathways or affecting cellular environment to induce the adaptive stress response and to activate the particular form of regulated cell death (RCD), and the development of cancer treatment based on novel targets and signaling regulatory networks provided by ferroptosis and autophagy.
Keywords:
1. Introduction
Different types of cell death are defined by distinct genetic, functional, biochemical, and morphological mechanisms. In general, two main types of cell death, accidental cell death (ACD) and regulated cell death (RCD), affect cell survival. In contrast to ACD, which is characterized by catastrophic and instantaneous cellular demise in response to chemical (such as dramatic pH variations), physical (such as osmotic forces, temperatures, or high pressures), or mechanical (such as shear forces) insults, RCD refers to the orderly and autonomous death of cells controlled by genes to maintain internal stability. RCD can be delayed or accelerated by pharmacological or genetic interventions [Citation1]. The establishment of signal amplification complexes can modulate their execution and induction, which are necessary for immune and developmental responses [Citation2]. RCD is involved not only in regulating the physiological mechanisms of tissue development or regeneration as programmed cell death but also occurs due to disturbances in the extracellular or intracellular microenvironment that are too prolonged or intense for adaptive responses to reinstate cellular homeostasis [Citation3–5]. When faced with perturbations in intracellular or extracellular homeostasis, eukaryotic cells can activate rapid adaptation mechanisms through post-translational modifications, transcription-dependent systems, or preexisting components to support adaptation over time. Additionally, stress sensors can dispatch signals that inhibit RCD. If adaptation fails, the signals dispatched by stress sensors become lethal, and the cells undergo RCD. Following primary cell death, a secondary wave of RCD can be promoted directly or indirectly by molecules released from cells succumbing to the primary insult, as well as by danger-associated molecular pattern (DAMP) signaling. Understanding this process can provide critical targets for pharmacological intervention.
Various types of RCD are known in greater detail, including apoptosis, necroptosis, ferroptosis, pyroptosis, autophagy-dependent cell death, NETosis, entosis, oxeiptosis, lysosome-dependent cell death (LCD), parthanatos, and alkaliptosis. Molecular mechanisms that can initiate and transmit RCD patterns exhibit a remarkable level of interconnection. Moreover, these forms of RCD can display a spectrum of morphological characteristics from complete apoptosis to complete necrosis, as well as immunoregulatory features from promoting inflammation and immunogenicity to anti-inflammatory responses and tolerance. This highlights the need to modulate multiple signal transduction modules to achieve robust cytoprotection, rather than regulating a single subroutine of RCD.
Exploring the effects of multiple signaling modules involved in the initiation, propagation, and execution of specific types of RCD may have significant implications for various disease outcomes. With an increasing understanding of the precise mechanisms of origin, intricate signal transduction modules, and effector mechanisms, the interplay between the pathophysiological relevance of each type of RCD and the pathogenesis of multiple diseases can be better understood. This perspective can lead to the discovery of novel targets related to modulating the program of RCD, controlling the signals emitted by affected cells associated with modulation of RCD, and integrating the modulation of different types of RCD.
Treatment strategies are increasingly focused on simultaneous modulation of two or more RCD subroutines, and researchers are exploring the crosstalk between multiple RCD pathways. The interplay between ferroptosis and autophagy has received particular attention due to their significant pathophysiological effects on multiple diseases and the tight molecular and regulatory networks connecting them.
Ferroptosis was first reported in 2003 when erastin was used to induce cellular death via RAS mutations [Citation6]. The term “ferroptosis” was introduced in 2012 [Citation7] to distinguish this type of RCD from others and to explore its involvement in drug regulation and disease pathogenesis. Ferroptosis is directly caused by unrestricted and lethal lipid peroxidation, leading to the rupture of the plasma membrane through iron catalysis, involving enzymatic (lipoxygenases) and non-enzymatic (Fenton’s reaction) mechanisms. The core regulatory mechanisms of ferroptosis are based on lipid peroxidation and iron accumulation. For example, the ACSL4-LPCAT3-ALOXs pathway can produce PLOOH with the engagement of RAB7A-dependent lipophagy to activate lipid peroxidation. Various antioxidant systems, such as the GSH-GPX4 antioxidant system, FSP1/CoQ10/NAD(P)H system, GTP cyclohydrolase-1 (GCH-1), transport (ESCRT)-III, and dihydroorotate dehydrogenase (DHODH)-mediated defense system, inhibit lipid peroxidation [Citation8–12].
The term “autophagy” originates from the Greek word for “self-eating” and describes a conserved cellular process that removes and degrades intracellular components, including pathogens, unfolded proteins, and damaged organelles, through lysosomal degradation after activation by various stress signals [Citation13]. Autophagy plays a central role in lipid metabolism management and homeostasis maintenance in eukaryotic cells. Autophagy is initiated by the activated unc-51-like kinase complex (ULK complex) under conditions of mTOR complex 1 (mTORC1) inhibition or 5′-AMP-activated protein kinase (AMPK) activation in response to stress signals. Vacuolar protein sorting 34 (VPS34) is subsequently activated [Citation14–16]. The VPS34 complex induces PI3P at the membrane, assembling and recruiting ubiquitin-like coupling systems. LC3 lipidation involves ATG3, ATG7, and ATG5-ATG12-ATG16L complexes, while ubiquitin enzymes recruit cargo receptors such as NBR and SQSTM1/P62. ATG9 is involved in phagosome expansion and autophagosome formation. The HOPS complex and SNAREs promote fusion between lysosomes and autophagosomes (referred to as autolysosomes). Finally, lysosomal hydrolases degrade cargo to recycle nutrients. Autophagy can be nonselective or selective, with both forms regulating ferroptosis. Selective autophagy can affect ferroptosis by influencing lipid peroxidation and iron accumulation, including ferritinophagy, lipophagy, clockophagy, chaperone-mediated autophagy (CMA), and mitophagy, while nonselective autophagy primarily modulates ferroptosis through alterations in energy metabolism. Exploring the relationship between ferroptosis and autophagy can deepen our understanding of the signaling pathways involved in the regulation of upstream sensors, signaling cascades, and downstream molecules associated with these two types of RCD, as well as uncover new crosslinks and cause-effect relationships among the signal transduction pathways of ferroptosis and autophagy. From a treatment perspective, focusing on this field may lead to the exploration of new signal regulation networks that provide attractive target points for therapeutic intervention. Precise simultaneous regulation of these two types of RCD may improve disease outcomes and current clinical approaches.
A bibliometric analysis of the crosstalk between ferroptosis and autophagy is necessary to gain awareness of the topic’s hotspots, develop the paradigm related to the recognition of this field, identify future research trends and applications related to regulating RCD to improve disease outcomes, and achieve a more precise understanding of the mechanisms underlying these two forms of RCD. This bibliometric analysis aimed to summarize the advancements in this field, changes in the paradigm, popular topics in different periods, and future trends of research and applications in ferroptosis and autophagy.
Using the WOSCC database, we found 756 records associated with the crosstalk between ferroptosis and autophagy. Furthermore, keyword analysis indicated that exploring the crosstalk between ferroptosis and autophagy contributes to cancer treatment. Therefore, our focus was on the potential applications of ferroptosis and autophagy in cancer, with an interpretation and explanation of the general trends provided by scientometrics.
2. Materials and methods
2.1. CiteSpace and VOS viewer
Both CiteSpace and VOS Viewer are science mapping tools used to describe the construction of a field based on bibliographic records. Through a topic search for the term “ferroptosis and autophagy” in WOSCC, we identified 756 records from 2012 to 2022. After excluding records with limited representation, such as editorial materials, meeting abstracts, and corrections, the number of articles in the dataset was reduced to 742, including both review articles and original research articles. If the term “ferroptosis and autophagy” is not explicitly mentioned in the item term abstracts or titles, the 742 records will not include any relevant publications ().
2.2. Data acquisition and processing
We conducted a comprehensive search of all articles from WOSCC using the following search strategy: TS=(ALL=(ferroptosis)) AND ALL=(autophagy); indexes=WOSCC, including Science Citation Index Expanded (SCIE), Social Sciences Citation Index (SSCI), and Emerging Sources Citation Index (ESCI); Timespan = 2012–2022; Slice length = 1 year; threshold for each 1 year: Top N = 25. To gain a better understanding of the influence of references or keywords, we calculated several essential indicators, such as the strength of citation bursts, betweenness centrality, and sigma values. Therefore, betweenness centrality measures the significance of nodes representing each publication or keyword in the graph. If the calculated centrality values were >0.1, the publication or keyword was identified as a key component. Sigma values were calculated based on the emergence burst and centrality, indicating the significance of variations in citations and structure.
3. Results
3.1. Annual growth trend
A total of 756 publications were extracted by searching the WOSCC. After filtering, 742 publications were included in the final analysis. The number of articles published per year is shown in . The overall trend showed steady growth. Between 2012 and 2017, the annual number of publications related to ferroptosis and autophagy experienced a gradual increase. Since 2017, there has been an accelerated growth in the annual number of publications. The majority of articles were published in 2021 (n = 249). The relationship between ferroptosis and autophagy is receiving increasing attention. Each increasing trend may indicate the expansion of the application of the regulatory network based on the crosstalk between autophagy and ferroptosis or a transformation of the paradigm regarding the recognition of these two forms of RCD. We will elaborate on each trend in the subsequent sections.
3.2. Distribution of countries and organizations
The references were published by 980 organizations from 55 countries or regions. In and , the top three countries with the most articles were China (n = 511), United States (n = 166), and Germany (n = 37). However, the centrality of China was 0.33, lower than that of Germany (centrality = 0.40) but higher than that of the USA (centrality = 0.32). It can be concluded that these three countries may provide a new paradigm to harness or recognize the relationship between ferroptosis and autophagy or reveal the novel regulatory networks of these two types of RCD from the perspective of improving the outcomes of various diseases. In , the size of the label is determined by the weight of the item representing the links between different institutions. In , Guangzhou Medical University and Central South University are the most productive institutions (n = 42), but their centralities are relatively low, with respective values of 0.04 and 0.03.
3.3. Authors and co-cited authors
In , 4183 authors were involved in our bibliometric analysis associated with research on ferroptosis and autophagy. Among them, 119 authors published at least three articles. Kang and Tang published the most articles related to ferroptosis and autophagy (n = 49), followed by Jiao Liu (n = 22), Guido Kroemer (n = 20), and Daniel Klionsky (n = 12). Kang and Tang also had the highest link strength (n = 193). The authorships of Rui Kang, Daolin Tang, Jiao Liu, Guido Kroemer, and Daniel Klionsky are steady and close.The most cited reference demonstrated by the co-citation analysis was authored by Dixon SJ (n = 523) in 2013, followed by Yang WS (n = 356), Gao MH (n = 305), Stockwell BR (n = 273), and Hou W (n = 261) (; ). The top ten authors with the highest centrality are listed in . In , a co-citation analysis of the authors is conducted. The colour indicates that the year of publication is closer to 2012 or 2022 if it is closer to purple or yellow, respectively.
Table 1. The top 10 countries and organizations with the most count.
3.4 Journals and co-cited journals
A total of 512 academic journals published articles on the relationship between autophagy and ferroptosis from 2012 to 2022. In journals that are being cited are on the right-hand side, while the journals that are citing are on the left-hand side, and the citation relationship is depicted by a coloured path. Each circle represents a specific number of articles and authors. The width and height of the circles represent the number of authors and articles, respectively. The highlighted lines indicate that these connections are more important than others.
For example, the connection between journals related to “molecular biology immunology” and “molecular biology genetics” can be inferred to be tighter through co-citation analysis. shows the top five journals with the highest bursts, centrality, sigma value, and citation count.
Table 2. The top 5 countries with the most centrality.
3.5. Co-citied references analysis
Of the 42,095 cited references, 243 were cited more than twenty times. A total of 243 references with 26,257 links were divided into four clusters, as shown in shows that cluster 0 (NCOA4-mediated ferritinophagy) started earlier. Cluster 1 (RNA-binding protein) is still ongoing, indicating that studies in this field may be a hotspot in the future (). The top 25 references with the strongest citation bursts are shown in . The strongest burst (strength = 30.64) occurred in a paper entitled “Regulation of ferroptotic cancer cell death by GPX4,” published in Cell by Yang et al. in 2014, with a citation burst from 2015 to 2019. This article focuses on the regulatory role of GPX4 in ferroptosis [Citation17]. Since GPX4 has been proven to be a central regulator of ferroptosis, many studies have been conducted to confirm this mechanism in treating various diseases, and many drugs have been created based on the regulation of ferroptosis according to this target. lists the top 10 publications with the highest centrality and count through co-citation analysis. Among them, the most co-cited reference was the article entitled “Ferroptosis, a regulated cell death nexus linking metabolism, redox biology, and disease,” published in Cell by Stockwell BR et al. in 2017. This study aimed to determine the relationship between ferroptosis and the pathogenesis and pathophysiological processes of various diseases. The reference with the highest centrality was published in Cell by Dixon et al. in 2012, entitled “Ferroptosis, an iron-dependent form of nonapoptotic cell death” [Citation7]. The article separated ferroptotic cell death from other forms of RCD and altered the paradigm of recognition of RCD.
Table 3. The top 10 authors with the most cited count and the highest centrality.
3.6. Keyword analysis of trending research topic
A total of 3021 keywords were extracted. As shown in , the top three keywords with the highest frequencies were autophagy (n = 283), cell death (n = 243), and oxidative stress (n = 165). The keywords with centrality values greater than 0.1 included molecular mechanism (n = 0.18), apoptosis (n = 0.17), activation (n = 0.17), cancer (n = 0.16), autophagy (n = 0.15), NF-κB (n = 0.14), tumor cells (n = 0.14), expression (n = 0.11), signaling pathway (n = 0.11), and mixed lineage kinase (n = 0.1) ().
Table 4. The collection table formed by the top 5 journals with the most citation count and the highest bursts, centrality and sigma value.
The largest cluster (#0) has 58 members and a silhouette value of 0.739. It is labeled as targeted therapy by LLR, cancer therapy by LSI, and pathogen defense response (8.91) by MI. The most relevant citer to the cluster was “Ferroptosis: bug or feature?” authored by Dixon et al. in the journal “Immunological Reviews” [Citation18].
The most representative publications to the second and third largest clusters are respectively “Therapeutic strategies of drug repositioning targeting autophagy to induce cancer cell death: from pathophysiology to treatment.” and “Murine double minute-2 prevents p53-overactivation-related cell death (podoptosis) of podocytes.” (Cluster 2) () [Citation19,Citation20]. The top eight keywords with the strongest citation bursts are shown in . Iron homeostasis was the keyword with the strongest citation bursts (strength = 5). As shown in , targeted therapy (cluster 0) is ongoing (). The keywords used are shown in . The weight of each item was recorded.
Figure 11. The timeline view of keywords associated with crosstalk between ferroptosis and autophagy.
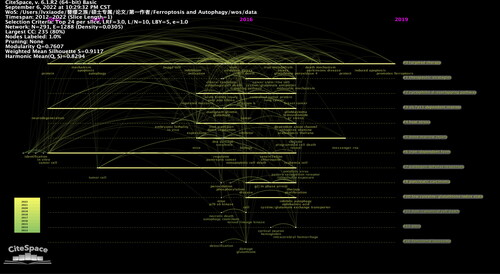
Table 5. The collection of the top 10 cited references with the most count and centrality.
4. Discussion
4.1. General information
Based on data from the WOSCC from 2012 to 2022, a total of 756 articles associated with the crosstalk between ferroptosis and autophagy were issued in 512 journals by 4183 authors in 980 organizations from 55 countries or regions.
The growing trend in publications indicates that the crosstalk between ferroptosis and autophagy is attracting increasing interest and attention. The annual count of publications has experienced a climate shift since 2018. From the integrative analysis of the timeline view of co-cited references and the annual trend of publications, we found that references published after 2018 mainly belonged to cluster 0 (NCOA4-mediated ferritinophagy) and cluster 1 (RNA-binding protein). The most relevant reference to cluster 0 was published in Autophagy by Xin Chen et al. entitled “Ferroptosis, machinery and regulation” in 2021. This article elaborately discusses the molecular mechanisms and direct or indirect regulation of ferroptosis [Citation21]. The summary of the relationship between autophagy and ferroptosis focuses on the role of selective autophagy in modulating ferroptosis. During autophagy, a family of autophagy-related (ATG) proteins regulates the construction of a series of membrane structures (such as phagophores, autophagosomes, and autolysosomes) and the development of autophagy. The forms of selective autophagy, such as ferritinophagy, lipophagy, clockophagy, mitophagy, and CMA, are involved in ferroptotic cell death by influencing iron accumulation and lipid peroxidation.
Ferritinophagy, the autophagic turnover of ferritin, induces cellular ferroptosis via iron accumulation. The repression of gene regulating microtubule-ATG5-associated protein 1 light chain 3 (MAPILC3/LC3), ATG7, ATG13, ATG3, and ELAV-like RNA-binding protein 1 (ELAVL1/HuR) further modulated ferritinophagy-mediated ferroptosis, which was used in sorafenib-induced liver fibrosis by promoting ferroptosis in human hepatic stellate cells [Citation22–25].
Lipophagy is an important form of selective autophagy that decomposes lipid droplets (LDs) via autolysosomes. LDs can transfer free fatty acids into the core to protect cells from PUFA-induced oxidative stress [Citation26]. When lipophagy was enhanced, the level of PUFAs can be increased leading to ferroptosis. Lipophagy-mediated ferroptosis has been reported to be suppressed in vitro after knockdown of the Rab7a member RAS oncogene family (RAB7A) [Citation27].
As a type of selective autophagy, clockophagy, which degrades the core circadian clock protein aryl hydrocarbon receptor nuclear translocator-like (ARNTL/BMAL1), can accelerate ferroptosis. ARNTL is involved in the inhibition of ferroptosis by activating hypoxia-inducible factor 1-alpha (HIF1α) through transcriptional downregulation of HIF2 expression. Sequestosome 1 (SQSTM1/P62), the autophagy receptor for clockophagy, mediates ARNTL degradation and promotes ferroptosis [Citation28].
Mitophagy is crucial for maintaining the quality and quantity of mitochondria, and Parkin RBR E3 ubiquitin protein ligase (PRKN/PARK2) and PTEN-induced kinase 1 (PINK1) are the two major regulators of mitophagy [Citation29]. Mitochondrial iron accumulation can be triggered by the degradation of SLC25A28 and SLC25A37 mediated by the PINK1-PRKN pathway [Citation30]. The role of mitophagy in ferroptosis requires further exploration [Citation31,Citation32]. Mitophagy can promote ferroptotic death in human cancer cells via a mitochondrial complex I inhibitor (BAY87-2243) or heme oxygenase 1 (HMOX1). In contrast, enhanced mitophagy can suppress ferroptosis. For instance, mitophagy induced by the uncoupler carbonyl cyanide m-chlorophenylhydrazone (CCCP) represses ferroptosis in human HT1080 cells expressing PRKN [Citation33].
CMA directly degrades cytoplasmic proteins in the lysosomes [Citation34]. The pathway begins with a combination of heat shock protein family A member 8 (HSPA8/HSC70) and proteins with a KFERQ-like motif. Subsequently, the targeted proteins are recognized by lysosome-associated membrane protein type 2 A (LAMP2A), leading to lysosomal degradation. GPX4, a key enzyme in the antioxidant defense system in ferroptosis, is a protein containing a KEFRQ-like motif. It has been proved to be degraded by HSP90-mediated CMA during erastin-induced ferroptosis. 2-amino-5-chloro-N,3-dimethylbenzamide (CDDO) can inhibit the combination of HSP90 and LAMP2A, resulting in the suppression of ferroptosis by increasing the level of GPX4 during erastin-induced ferroptosis in vitro [Citation35].
The most relevant reference to cluster 1 was published in Cell Research by Tang et al. entitled “Ferroptosis: molecular mechanisms and health implications” in 2020. The reference not only emphasized the known role of selective autophagy and CMA in ferroptosis but also summarized the relationship between non-selective autophagy and ferroptosis activators [Citation36]. Furthermore, the reference collected evidence that epigenetic regulation and some transcriptional factors impacted the propensity of cellular ferroptotic death.
From a comprehensive analysis of annual growth trends and references with the strongest citation bursts, we found that citation bursts of two publications began in 2018. One was published in Cellular and Molecular Life Sciences by Cao et al. entitled “Mechanisms of ferroptosis.” In 2016, another study published in Molecular and Cellular Oncology by Yan Yu et al. entitled “The ferroptosis inducer erastin enhances sensitivity of acute myeloid leukemia cells to chemotherapeutic agents” in 2015 [Citation37,Citation38]. It can be concluded that the first article by Jennifer Yinuo Cao provided the fundamental mechanism to research the relationship of ferroptosis and autophagy in the context of initial dynamics and regulatory networks, crosslinked signal pathways, and synergistic or antagonistic effect on varieties of disease, and the second article authored by Yan Yu created the direction of research related to the interaction of these two forms of RCD and discovering the novel targets and relevant drugs based on the crosslinked regulatory patterns.
As shown in the analysis of countries and organizations analysis ()(), the United States and China contributed the most to publications on research related to ferroptosis and autophagy. As illustrated in and , of the top 10 institutions that published the most research items, seven were in China, one in Sweden, and one in the United States. However, the centrality of China (n = 0.33) was lower than that of Germany (n = 0.4), which was higher than that of the USA (n = 0.32), indicating that Germany maintained its leading role in fields related to ferroptosis and autophagy. Moreover, the centrality of Germany, China, the United States, France, and England was high, which means that their global cooperation in research was essential for the development of ferroptosis and autophagy.
Table 6. Summary of the largest 10 clusters of keywords analysis.
According to , , and , both Kang and Tang published the most articles associated with ferroptosis and autophagy (n = 49). Dixon SJ is the most co-cited author, implying his distinguished contribution to this field. The research and work of his group are to discover the molecular mechanisms of ferroptosis and explore potential anticancer targets based on ferroptosis sensitization [Citation39–41].
Table 7. The collection table formed by the top 10 keywords with the most count and the highest centrality.
In journal analysis, Cell published studies on ferroptosis and asthma with the most cited counts. The journal Oncotarget published studies with the fourth-highest sigma value and the highest burst (n = 43.91). Cancer cells had the highest centrality (n = 0.62) and sigma value (n = 41.07), which played critical roles in disseminating research on ferroptosis and autophagy. The other journals of the top five with the most cited counts were Nature, Cell Death and Differentiation, Autophagy, and National Academy of Science of the USA. The other journals with centrality values more than 0.1 included Cell, Cell Death Differentiation, and Cancer Research.
4.2. The hotspots and trending
Bibliometrics provides various techniques for analyzing academic areas. Therefore, keyword co-occurrence can identify hotspots, whereas the timeline view tracks their evolution. Additionally, reference clusters and citation bursts help discover novel paradigms in the discipline and characterize emerging topics.
In this study, we attempted to comprehensively evaluate the frontiers and hotspots of research on the crosstalk between ferroptosis and autophagy through the analysis of the keyword timeline (), keyword co-occurrence ( and ), keyword burst (), reference timeline (), and reference burst ().
4.2.1. Molecular mechanism of ferroptosis and autophagy
With the rapid expansion of studies on ferroptosis and autophagy, new molecular mechanisms for orchestrating these two forms of RCD have been actively investigated. The keyword with the highest centrality (n = 0.18) was “molecular mechanism,” which indicated that the articles associated with this field always altered the paradigm related to the recognition of ferroptosis and autophagy. For example, knockdown of NCOA4 increased ferritin levels and inhibited ferroptosis, whereas overexpression of NCOA4 increased ferritin degradation and promoted ferroptosis in PANC-1 and HT1080 cells, indicating a specific role of ferritinophagy-mediated ferritin degradation in promoting ferroptosis [Citation22]. The largest cluster in the co-cited reference analysis was NCOA4-mediated ferritinophagy. It can be concluded that most studies on the relationship between ferroptosis and autophagy focus on regulatory mechanisms. The crosstalk between the two forms of RCD is still being explored, such as the pathway of selective autophagy that influences ferroptosis and the exact mechanism of the transition from ferroptosis to autophagy. The receptors involved in the process of autophagy-dependent ferroptosis, such as NCOA4, RAB7A, SQSTM1, OPTN, CALCOCO2, TAX1BP1, have been explored with the advancement of the molecular mechanisms related to the pathways linking autophagy and ferroptosis. Recent studies have revealed that insufficient cellular autophagy can turn off Nrf2-mediated antioxidant defense while initiating nuclear factor NF-E2-related factor (Nrf2) - manipulated iron deposition and lipid peroxidation, leading to the development of ferroptosis [Citation42].
The role of autophagy in the regulation of iron metabolism and lipid peroxidation requires further investigation. Multiple autophagy-independent ferroptosis processes have also been reported to form a specific regulatory network. Despite the variations in the initial signal triggering ferroptosis, all pathways ultimately result in ROS-dependent lipid peroxidation, which is the cause of ferroptosis. The crosslinks among multiple modulatory networks based on the autophagy regulating ferroptosis will be further explored in the future.
In addition, common signal transduction pathways that modulate both ferroptosis and autophagy have attracted the attention of researchers. This idea may be derived from research on ferroptosis and other forms of RCD. For example, the mixed lineage kinase 3 (MLK3) signalling pathway has been shown to induce pyroptosis and ferroptosis in myocardial fibrosis in response to pressure overload. And the expression of miR-351 can inhibit the expression of MLK3 [Citation43]. This paradigm may be beneficial for understanding the relationship between ferroptosis and autophagy. For example, p53, a nuclear transcription factor, can trigger the expression of autophagy-related genes to induce autophagy. However, in the cytoplasm, it exerts a negative regulatory effect by inhibiting autophagy. Meanwhile, p53 can transcriptionally suppress the expression of SLC7A11, leading to GSH depletion and ferroptosis, which can be promoted by p53 acetylation at K117, K161, and K162 (hereafter p533KR/3KR) but repressed by an additional p53 acetylation at K98 [4445]. P53 facilitates ferroptosis by modulating lipid peroxidation via transcriptional induction of SAT1 or GSL2 [Citation46,Citation47]. Phosphorylation of protein kinases in relevant signaling pathways may provide novel targets for the treatment of multiple diseases. Similarly, the expression of specific signaling molecules can be inhibited by RNA. Nuclear transcription factor NF-κB is also involved in the regulation of autophagy and ferroptosis. It has been found that in fibroblasts collected from patients with Niemann–Pick disease, the level of lipid peroxidation and reactive oxygen species was higher than that in cells derived from healthy controls. Furthermore, fibroblasts obtained from patients were more susceptible to oxidative stress-induced apoptosis with the participation of NF-κB-dependent signaling pathways in this phenomenon [Citation48]. In addition to common transcription factors, similar epigenetic regulation impacting the propensity of cells to undergo ferroptosis and autophagy may also be hotspots.
The role of ferroptosis in the regulation of autophagy has also been the focus of the discovery of a novel relationship between these two forms of RCD. For example, phospholipid peroxidation inhibits autophagy by stimulating the delipidation of oxidized LC3-PE [Citation49]. With the analysis of keywords with the strongest citation burst illustrated, we found another approach in which ferroptosis modulates autophagy. Autophagy can be induced by endoplasmic reticulum (ER) stress sensed by three upstream signaling proteins: IRE1 (inositol requiring protein-1), ATF6 (activating transcription factor-6), and PERK [protein kinase RNA (PKR)-like ER kinase]. Inhibition of cystine-glutamate exchange by ferroptotic agents leads to activation of the ER stress response and upregulation of the CHAC1 (glutathione-specific gamma-glutamylcyclotransferase 1) gene [Citation50,Citation51].
4.2.2. The reciprocal induction based on the alteration of cellular microenvironment
The connection between ferroptosis and autophagy can also occur sequentially by modulating cellular microenvironments, such as the inflammatory environment. Alteration of a variety of immune signaling molecules resulting from one form of RCD can induce or inhibit other forms of cellular RCD. For example, HMGB1 (high mobility group box 1) is released by ferroptotic cells and drives inflammation by activating macrophages to produce proinflammatory cytokines [Citation37,Citation52]. HMGB1 has been reported to play a central role in autophagy induction. Extracellular HMGB1 binds to the receptor for advanced glycation end-products to induce autophagy [Citation53]. Type I IFN production represses system Xc- and induces ferroptosis, which can be modulated by autophagy [Citation54]. The autophagy pathway activates type I IFN production in plasmacytoid dendritic cells [Citation55]. In contrast, autophagy proteins negatively regulate RIG-I-like receptor (RLR)-mediated induction of type I IFN production through the autophagic elimination of damaged mitochondria [and reduction of reactive oxygen species (ROS)] and by the binding of ATG5–ATG12 to the caspase recruitment domains of RLR signaling molecules [Citation56,Citation57]. Moreover, the autophagy protein ATG9A, but not ATG7, can negatively modulate the activation of STING, a transmembrane protein that is required for the efficient activation of type I IFN and pro-inflammatory cytokine production in response to stimulatory DNA [Citation58]. Thus, it appears that autophagy proteins can regulate the development of ferroptotic cell death. DAMPs that trigger autophagy, such as extracellular ATP/K+ efflux and reactive oxygen species (ROS), can also bind to NOX4 or its isoenzymes to induce ferroptosis [Citation59–61]. The inflammasome signaling pathway can be activated by TLR4-TRAF6-IKKs-NLRP3 signal transduction. Thus, the regulation of TLR4 may be involved in autophagy and ferroptosis.
Further functional investigations into the complicated reciprocal regulation based on alteration of the cellular environment may provide a new way to effectively treat diseases.
4.2.3. Potential applications of ferroptosis and autophagy in cancer
From the summary of the largest 10 clusters of keyword analysis, we found that the labels of the first and second largest clusters were cancer therapy and therapeutic strategies, respectively. It can be inferred that research on specific crosslinked regulatory mechanisms can ultimately promote cell type-specific or tissue-specific interventions in the crosstalk between ferroptosis and autophagy in multiple distinct diseases. The potential applications of specific mechanisms may originate from the exploration of novel targets, new signaling pathways, and effective regulatory methods.
Regulation of autophagy and ferroptosis in tumorigenesis and cancer therapy is not only influenced by tumor suppressors and oncogenes but also by the tumor microenvironment (TME). Targeted pathways related to ferroptosis and autophagy have been implicated in immunotherapy, chemotherapy, and radiation therapy. The interrelated signal molecules in the transduction pathways related to the modulation of autophagy and ferroptosis can provide an effective target for cancer therapy in the context of gene mutations, epithelial-mesenchymal transition, tumorigenesis, and development. For example, both ferroptosis and autophagy can be applied for the targeted treatment of cancers with RAS mutations [Citation7,Citation62]. Acquisition of epithelial-mesenchymal transition is associated with the inhibition of cytotoxic T lymphocyte (CTL)-mediated tumor cell lysis. Resistant cells exhibit attenuation in the formation of an immunologic synapse with CTLs, along with the induction of autophagy in the target cells [Citation63]. Meanwhile, enhanced cell adhesion, which involves CDH1/E-cadherin, the Hippo pathway, and integrins such as ITGA6 and ITGB4, confers resistance to ferroptosis [Citation64–66]. In addition, tumor heterogeneity in autophagy-dependent ferroptosis may result in different biological behaviors regarding the dynamic characteristics of cell death [Citation67].
4.2.3.1 The crosslinks between ferroptosis and autophagy in immunotherapy resistance
Immunotherapy resistance, which leads to poor prognosis in various tumors, can be handled and explored from the perspective of the crosstalk between ferroptosis and autophagy. For example, early ferroptotic cells can release DAMPs (ATP and high-mobility group box 1 (HMGB1)) and induce the phenotypic maturation of dendritic cells derived from the bone marrow. The stimulated adaptive immune system can transform the immunologically cold state into a checkpoint blockade-responsive state, which results in the ability to enhance potent antitumor immune responses that have been destroyed by the expression of the WNT/β-catenin signaling pathway, loss of PTEN expression, activation of the MAPK signaling pathway, loss of tumor antigen expression, and interferon-gamma signaling pathway [Citation68–70]. Moreover, autophagy relies on multiple DAMPs beyond HMGB1 and ATP, such as surface exposure to calreticulin, secretion of type-1 interferons, and release of annexin A1 [Citation71]. Hence, further research is necessary to refute or confirm the possibility that different cell death modalities, such as ferroptosis and autophagy, may induce an adaptive immune system through diverse mechanisms using only a partially overlapping set of DAMPs. In addition, various eat-me signals on the surface of tumor cells can also stimulate antitumor immunity to overcome immunotherapy resistance when ferroptosis or autophagy occurs. Enrichment of 1-steaoryl-2-15-HpETE-sn-glycero-3-phosphatidylethanolamine (SAPE-OOH) facilitates phagocytosis by TLR2 in macrophages [Citation72]. Mitophagy functions as an inhibitor of innate immunity in response to various stimuli that suppress type I IFN production, remove damaged mitochondria, and inhibit inflammasome activation, thus reducing the production of IL-1β and IL-18 as a result of preventing the accumulation of mitochondria-derived DAMPs, such as ROS and mitochondrial DNA (mtDNA) [Citation73]. Eat-me signals such as NIPSNAP1 (nipsnap homolog 1) and NIPSNAP2 can accumulate on the mitochondrial outer membrane following mitochondrial depolarization, recruiting autophagy receptors and adaptors, as well as human Atg8 (autophagy-related 8)-family proteins to facilitate mitophagy and formation of anti-inflammatory TME [Citation74].
In addition to tumor-cell-intrinsic mechanisms, diverse extrinsic mechanisms play a critical role in immunotherapy resistance, such as immune suppressor cells within the TME, including regulatory T cells (Tregs) and tumor-associated macrophages (TAMs) [Citation68]. The number, differentiation, and infiltration of Tregs can have a profound effect on immunotherapy. For example, the effect of anti-cytotoxic T-lymphocyte antigen 4 (CTLA-4) immunotherapy is related to the ratio of effector T cells to Tregs in the TME, and the mechanism has been used to potentiate the efficacy of CTLA-4 blockade by increasing the ratio of effector T cells and Tregs with GM-CSF-transduced tumor cell vaccine [Citation75]. CTLA-4 is an effective target for cancer therapy and is closely associated with autophagy and ferroptosis. The PI3K/AKT/mTOR pathway can be activated to induce translocation of forkhead box protein O1 (FOXO1) to the nucleus, suppressing LC3β transcription and autophagosome formation in the presence of CTLA-4 [Citation76]. The expression of CTLA-4 has been reported to be higher in groups with higher ferroptosis scores. However, the outcome of immune tolerance regulated by overexpression of CTLA-4 via ferroptosis is altered in various tumors, which needs to be further explored specifically in extensive investigations [Citation77,Citation78]. Activation of autophagy can increase the expression of CTLA-4 and expand Tregs, which can inhibit inflammation and regulate the effect of anti-CTLA-4 immunotherapy [Citation79].
Furthermore, anti-PD-L1 immunotherapy resistance is strongly associated with Treg infiltration [Citation80]. Hence, the crosslinks between autophagy and ferroptosis in Treg depletion are potential targets for restoring antitumor immunity. Notably, recent studies have shown that after activation by TCR/CD28 co-stimulation, Treg cells can induce the expression of GPX. Deletion of GPX4 in Treg cells can result in ferroptosis and production of IL-1β, which can mediate lung neutrophilia and IL-33 expression [Citation81,Citation82]. In addition, activation of Treg cells is closely related to autophagy.
Cancer immunotherapy can be enhanced by blocking PD-1/PD-L1 immune checkpoints. Increasing evidence suggests that ferroptosis and autophagy may affect immunotherapy resistance by modulating PD-1/PD-L1 through various methods, in addition to regulating Tregs. The epidermal growth factor receptor (EGFR)/β1,3-N-acetylglucosaminyltransferase-3 (B3GNT3) pathway in a breast tumor model uses PD-L1 glycosylation to inhibit autophagic degradation of PD-L1, allowing for tumor immune escape [Citation83]. Palmitoylation of the acyl transferase DHHC3 can induce autophagic degradation of PD-L1 in a colon tumor model, which promotes immune suppression [Citation84]. Huntingtin-interacting protein 1-related (HIP1R), an autophagy receptor for PD-L1 binding, can suppress tumor growth by inducing the degradation of PD-L1 in lysosomes and activating T cells [Citation85]. In addition, PD-L1 has been proved to be positively linked with heterogeneous nuclear ribonucleoprotein L (HnRNPL). Overexpression of HnRNPL can increase the stability of YY1 mRNA and, in turn, generate pro-proliferation and anti-apoptosis effects [Citation86,Citation87]. Ferroptosis plays a significant role in T cell-induced adverse effects on cancer cells, whereas HnRNPL inhibits ferroptosis in Jurkat T cells, leading to castration-resistant prostate cancer (CRPC) and cancer immune escape via the YY1/PD-L1 axis. Moreover, repression of HnRNPL can enroll CD8+ T cells to boost the anti-PD-1 effect in CRPC tumors. However, tumor cells can promote autophagy by inhibiting the degradation of PD-L1 through transcriptional modifications. Tumor-derived exosomes can suppress the antitumor activity of T cells and reinforce the immune evasion ability by inhibiting DC maturation, upregulating the expression of CD11b and PD-L1, and downregulating the expression of surface markers such as MHC-II, CD80, and CD86 [Citation88–90]. In addition to PD-1/PD-L1, CTLA4, and indoleamine 2,3-dioxygenase (IDO), SIRPα/CD47 immune checkpoints can protect cancer cells from macrophage phagocytosis [Citation91,Citation92]. Pancreatic cancer cells can evade phagocytes through CD47 binding to SIRPα on macrophages [Citation93]. Likewise, PD-L1 can be released by exosomes that are secreted by tumor cells as vesicles (30–150 nm), which have a double-layer membrane and evade the degradation of lysosomes [Citation94]. Autophagy plays a vital role in the formation of exosomes. For example, the C-terminus (GIPC) and G alpha-interacting protein (GAIP), key autophagy regulators, have been shown to increase exosome biogenesis in pancreatic tumor cells, indicating autophagy-mediated exocytosis [Citation95]. The indispensable roles of ATG5 and ATG16L1 in exosome biogenesis have been well established [Citation96]. A therapeutic strategy for anti-exosomal PD-L1 has been developed with a deeper understanding of ferroptosis. Phototheranostic metal-phenolic networks (PFGMPNs) formed via semiconductor polymer assembly that encapsulates FIN (Fe3+) and GW4869 (exosome inhibitor) can enhance ferroptosis and induce effective antitumor immunity [Citation97]. The crosslinks between autophagy and ferroptosis may provide potential therapeutic strategies combined with immune checkpoint inhibitors (ICIs) and drive immunotherapy of tumors to promising results, especially on CTLA-4 and PD-1 targets.
Tolerogenic DCs enhance the proliferation of CD25+ Foxp3+ Treg cells. Furthermore, autophagy induces tolerance, driving the DC phenotype to tolerogenic functions [Citation98]. Another subset of cell TAMs can also affect tumor cell responses to immunotherapy. The polarization of TAMs has been shown to contribute to the pathogenesis and immunotherapy of tumors. TAMs can polarize into two major phenotypes: antitumor M1 (TAM1) and protumor M2 (TAM2). TAM2 is often the dominant subset of TAMs in TME. Compared to TAM2, TAM1 has been shown to exert higher resistance to ferroptosis because of the higher levels of inducible nitric oxide (NO) synthase (iNOS)/NO•. The strategy for enhancing antitumor immunity in the TME can be based on the regulation of ferroptosis by iNOS/NO•, which inhibits TAM2 survival without influencing TAM1 [Citation99]. Similarly, the overexpression of miR-326 can promote autophagy along with the downregulation of iNOS expression, which can be further explored to activate TAM1 and enhance antitumor immunity in the TME [Citation100]. The expression of TYRO3 can inhibit tumor cell ferroptosis and facilitate the polarization of TAM1 to TAM2, resulting in anti-PD-1/PD-L1 immunotherapy resistance [Citation101]. The polarization of TAMs is tightly connected with p62-mediated autophagy, leading to the degradation of NF-κB induced by TLR2 signaling.
4.2.3.2 The crosslinks between ferroptosis and autophagy in synergies with antitumor immune response
The crosslinks between ferroptosis and autophagy play a critical role in synergies with antitumor immune responses from the perspective of modulating the adaptive and innate immune systems. The relationship between crosslinks and adaptive immune cells has been discovered in the survival of naïve T cells in the periphery, depending on TCR interactions with stromal cells and IL-7 signaling, and requiring Atg3-dependent autophagy in an intrinsic manner [Citation102]. Meanwhile, IL-7 signaling has been shown to induce mTORC2 signaling in memory CD4+ T cells to promote longevity by preventing ferroptosis, which is tightly connected with GPX4 downregulation [Citation103]. In contrast, naïve T cells have been reported to easily undergo apoptosis due to the selective loss of FAK family-interacting protein of 200 kDa (FIP200). This leads to poor antitumor immunity via autophagy deficiency, mitochondrial overactivation, and high reactive oxygen species production in T cells. Mitochondrial complex I inhibition can rescue T cell apoptosis and promote tumor immunity [Citation104]. Mitochondrial complex I inhibition can trigger ferroptosis by increasing the levels of mitophagy-dependent ROS [Citation31]. This contradiction inspired us to further explore the regulatory network between ferroptosis and autophagy, to find the balance between these two forms of RCD, and to provide a potential explanation for the deficiency of SLC7A11 in human naïve CD4+ T cells. T cell activation is closely related to ferroptosis and autophagy. For example, when TCR is stimulated, activated T cells increase autophagy levels, which results from a rapid elevation of calcium levels, activating the phosphorylation of the ULK1 complex by AMPK and promoting autophagy [Citation105,Citation106]. When CD4+ T cells are activated, ferroptosis-related proteins are drastically upregulated [Citation107,Citation108]. Autophagy and ferroptosis can regulate T cell differentiation by affecting different metabolic pathways. Enhanced glycolysis promotes the differentiation of T cells into T helper (Th) cells when the mTOR signaling pathway is induced. With higher levels of activated AMPK, primitive T cells would undergo lipid peroxidation and preferentially differentiate into T regulatory cells (Treg cells) [Citation109]. The phosphoinositide 3-kinase (PI3K)/protein kinase B (AKT)/mammalian target of rapamycin (mTOR) pathway is a critical regulator of autophagy, and lipid peroxidation directly promotes ferroptosis. The development and function of T cells can be disrupted by inadequate degradation of mitochondrial components, and ROS production can be induced by defective autophagy [Citation110]. The formation of memory T cells can be induced by lipophagy [Citation111]. Autophagy plays an indispensable role in the establishment of the lasting memory of effector CD8+ T cells, contributing to antitumor immunity [Citation112,Citation113]. CD8+ T cells can induce ferroptosis in surrounding macrophages and other activated immune cells to accelerate inflammation through IFN-γ, which can downregulate the expression of SLC7A11 and SLC3A2 [Citation114].
In addition, the crosslinks between autophagy and ferroptosis are involved in B cell development, antibody production, and differentiation. The specific deletion of Atg5 in B cells results in a deficient transition for these autophagy-insufficient B cell progenitors between pro- and pre-B cell phases in the bone marrow, indicating the critical role of autophagy in B cell development [Citation115]. In addition to the process of advancement in the center, B cells can be severely influenced by both ferroptosis and autophagy in the periphery. B cells include two main subgroups. One of them, called B1-lymphocytes (B1a and B1b lymphocytes), which arise from fetal liver precursors, always gather in the peritoneal and pleural cavities as well as mucosal tissues. Another group called B2-lymphocytes (follicular B lymphocytes and marginal zone B (MZB) lymphocytes), derived from precursors in the bone marrow, are enriched in secondary lymphoid organs. B1 lymphocytes and MZB cells are always involved in the rapid humoral response to achieve natural defense, whereas follicular B lymphocytes play an essential role in response to exogenous antigens. Compared to follicular B cells, MZB and B1 cells have a tighter connection with ferroptosis. The enhanced ferroptosis sensitivity and fatty acid uptake resulting from the higher expression of CD36 (the protein responsible for fatty acid transport) leads to regulators targeting GPX4, which easily induces the activation of maintenance, progression, and antibody response of B1 cells and MZB cells [Citation116]. Autophagy has been confirmed to be required for B1a cell homeostasis [Citation117]. Follicular B cells differentiate into plasma cells and memory B cells with high affinity and long life, characterized by selection and mutation through the germinal center (GC) reaction induced by TFH cells. Plasma cells require autophagy for sustainable immunoglobulin production. In contrast, TFH cells are susceptible to ferroptosis, and the selenium-GPX4-ferroptosis axis is the principal regulator of TFH cell homeostasis [Citation118]. Effector B cells can be divided into BE1 and BE2 lymphocytes based on cytokine secretion. Therefore, BE1 lymphocytes can generate IFNγ to promote the transition between Th0 and Th1 cells, while BE2 lymphocytes can produce IL-4 to induce Th0 cell differentiation to Th2. Hence, the regulation of B cells by ferroptosis and autophagy may coordinate the immune system by modulating T cell differentiation.
The differentiation of monocytes to macrophages has been reported to be facilitated by autophagy, which is stimulated by colony-stimulating factor 1 (CSF1) and CSF2 [Citation119]. p53 acetylation and ROS production due to iron overload can lead to M1 polarization by upregulating the expression of M1 markers, including IL-1β, IL-6, and TNF-α, and decreasing the levels of M2 markers such as TGM2 [Citation120,Citation121]. M1 polarization can also be stimulated by suppression of the mTOR pathway, which is the master regulator of autophagy [Citation122]. NF-κB can be activated after M1 polarization; in fact, the activation of NF-κB drives macrophages to either M1 or M2 polarization. NF-κB p65 cytosolic ubiquitination induced by TLR2 signaling can result in its degradation via p62-mediated autophagy. The repression of autophagy can rescue NF-κB activity and drive macrophages to the M2 phenotype [Citation123,Citation124]. Similarly, IL-6 and CCL2 can trigger M2 phenotype by inducing autophagy in macrophages [Citation125]. In addition, along with the occurrence of ferroptotic cell death, inflammation-related immunosuppression can occur through macrophage polarization [Citation100].
As an immunological tolerance molecule, IDO induced by tumor-associated myeloid-derived suppressor cells (MDSCs), TAMs, and tumor cells can alleviate effective antitumor immunity, promote immunological tolerance, and facilitate tumor growth by suppressing inflammatory DC maturation, augmenting tolerogenic antigen-presenting cells (APCs), and suppressing CTL responses [Citation126]. Autophagy can suppress inflammation and inhibit inflammation-mediated IDO production [Citation127].
In summary, studying the crosslinks between autophagy and ferroptosis in cancer therapy is an extensive and rapidly developing field. The emerging view about the role of these two forms of RCD in immunotherapy resistance and synergies with the antitumor immune response has become an increasingly promising strategy to improve the prognosis of cancer patients and discover more specific cell death-inducing drugs that act on tumor cells with minimal side effects on normal tissues.
In addition to cancer therapy, the exploration of the crosslinks between ferroptosis and autophagy contributes to various diseases with respect to the modulation of immunity and inflammation. The mechanism of regulating inflammation and immunity results in the development of selective and safer drugs that specifically target the common signaling pathways of ferroptosis and autophagy to improve therapeutic strategies for various diseases.
4.3. Strength and limitations
This is the first bibliometric study to systematically analyze publications associated with the crosstalk between ferroptosis and autophagy. This study will enlighten the public about the importance of dynamic and associative opinions to recognize ferroptosis and autophagy, provide scholars with an entire picture of research associated with the connection between ferroptosis and autophagy, and serve as an objective and comprehensive guide for the future advancement of the research field associated with the relationship between ferroptosis and autophagy.
The limitations of this study include language and publishing bias due to the exclusion of articles published in languages other than English. Furthermore, the Matthew effect, which may influence the results of the bibliometric analysis, was not considered.
4. Conclusion
In summary, this study provides useful data for potential collaborations among researchers, institutions, and countries/regions, and the relationship of particular journals. It summarizes the development of recognition related to the connection between ferroptosis and autophagy and the current molecular crosslinked mechanisms in the context of common signal transduction pathways or affecting the cellular environment to induce the adaptive stress response, activate the particular form of RCD, and predict the hot topics, trends, and paradigms in research in this field. Further research is needed to identify additional and common physiological triggers for ferroptosis and autophagy, clarify new molecular mechanisms that insulate ferroptosis from autophagy or connect ferroptosis and autophagy, discover the specific immune consequences of ferroptosis or autophagy, and explore more approaches for these two forms of RCD to indirectly intrigue or inhibit each other’s relevant programs. Using bibliometric analysis of keyword clusters, we elaborately discussed how autophagy and ferroptosis affect cancer therapy and their potential roles in treating cancer. This paradigm may help us find new targets with the development of pharmacological mechanisms and combine them with therapeutic strategies for multiple diseases to effectively improve the prognosis of patients.
Authors’ contributions
XiaodiLv, Yanglai Gao, Ming Dong, and Ying Wei designed this study. XiaodiLv, Bin Wang, Weifeng Tang, Jingjing Qin, and Wenqian Wang retrieved, screened, and analysed the literature. XiaodiLv wrote the manuscript. Gao and Wei revised the manuscript. All authors have contributed to the manuscript and approved the submitted version.
Dislosure statement
No potential conflict of interest was reported by the author(s).
Data availability statement
The datasets used and/or analyzed during the current study are available from the corresponding author on reasonable request.
Additional information
Funding
References
- Galluzzi L, Vitale I, Aaronson SA, et al. Molecular mechanisms of cell death: recommendations of the nomenclature committee on cell death 2018. Cell Death Differ. 2018;25(3):1–19.
- Christgen S, Tweedell RE, Kanneganti T-D. Programming inflammatory cell death for therapy. Pharmacol Ther. 2021;232:108010.
- Galluzzi L, Pedro B-S, Manuel J, et al. Regulated cell death and adaptive stress responses. Cell Mol Life Sci. 2016;73(11–12):2405–2410.
- Fuchs Y, Steller H. Programmed cell death in animal development and disease. Cell. 2011;147(4):742–758.
- Conradt B. Genetic control of programmed cell death during animal development. Annu Rev Genet. 2009;43(1):493–523.
- Dolma S, Lessnick SL, Hahn WC, et al. Identification of genotype-selective antitumor agents using synthetic lethal chemical screening in engineered human tumor cells. Cancer Cell. 2003;3(3):285–296.
- Dixon SJ, Lemberg KM, Lamprecht MR, et al. Ferroptosis: an iron-dependent form of nonapoptotic cell death. Cell. 2012;149(5):1060–1072.
- Ursini F, Maiorino M. Lipid peroxidation and ferroptosis: the role of GSH and GPx4. Free Radic Biol Med. 2020;152:175–185.
- Doll S, Freitas FP, Shah R, et al. FSP1 is a glutathione-independent ferroptosis suppressor. Nature. 2019;575(7784):693–698.
- Mao C, Liu X, Zhang Y, et al. DHODH-mediated ferroptosis defence is a targetable vulnerability in cancer. Nature. 2021;593(7860):586–590.
- Dai E, Meng L, Kang R, et al. ESCRT-III–dependent membrane repair blocks ferroptosis. Biochem Biophys Res Commun. 2020;522(2):415–421.
- Kraft VA, Bezjian CT, Pfeiffer S, et al. GTP cyclohydrolase 1/tetrahydrobiopterin counteract ferroptosis through lipid remodeling. ACS Cent Sci. 2019;6(1):41–53.
- Mizushima N, Levine B. Autophagy in human diseases. N Engl J Med. 2020;383(16):1564–1576.
- Hara T, Takamura A, Kishi C, et al. FIP200, a ULK-interacting protein, is required for autophagosome formation in mammalian cells. J Cell Biol. 2008;181(3):497–510.
- Backer JM. The intricate regulation and complex functions of the class III phosphoinositide 3-kinase Vps34. Biochem J. 2016;473(15):2251–2271.
- Ohashi Y, Tremel S, Williams RL. VPS34 complexes from a structural perspective. J Lipid Res. 2019;60(2):229–241.
- Yang WS, SriRamaratnam R, Welsch ME, et al. Regulation of ferroptotic cancer cell death by GPX4. Cell. 2014;156(1–2):317–331.
- Dixon SJ. Ferroptosis: bug or feature? Immunol Rev. 2017;277(1):150–157.
- Thomasova D, Bruns HA, Kretschmer V, et al. Murine double minute-2 prevents p53-overactivation-related cell death (podoptosis) of podocytes. J Am Soc Nephrol. 2015;26(7):1513–1523.
- Yoshida GJ. Therapeutic strategies of drug repositioning targeting autophagy to induce cancer cell death: from pathophysiology to treatment. J Hematol Oncol. 2017;10(1):67.
- Chen X, Li J, Kang R, et al. Ferroptosis: machinery and regulation. Autophagy. 2021;17(9):2054–2081.
- Hou W, Xie Y, Song X, et al. Autophagy promotes ferroptosis by degradation of ferritin. Autophagy. 2016;12(8):1425–1428.
- Gao M, Monian P, Pan Q, et al. Ferroptosis is an autophagic cell death process. Cell Res. 2016;26(9):1021–1032.
- Park E, Chung SW. ROS-mediated autophagy increases intracellular iron levels and ferroptosis by ferritin and transferrin receptor regulation. Cell Death Dis. 2019;10(11):822.
- Zhang Z, Yao Z, Wang L, et al. Activation of ferritinophagy is required for the RNA-binding protein ELAVL1/HuR to regulate ferroptosis in hepatic stellate cells. Autophagy. 2018;14(12):2083–2103.
- Bailey AP, Koster G, Guillermier C, et al. Antioxidant role for lipid droplets in a stem cell niche of drosophila. Cell. 2015;163(2):340–353.
- Bai Y, Meng L, Han L, et al. Lipid storage and lipophagy regulates ferroptosis. Biochem Biophys Res Commun. 2019;508(4):997–1003.
- Yang M, Chen P, Liu J, et al. Clockophagy is a novel selective autophagy process favoring ferroptosis. Sci Adv. 2019;5(7):eaaw2238.
- Narendra DP, Jin SM, Tanaka A, et al. PINK1 is selectively stabilized on impaired mitochondria to activate parkin. PLOS Biol. 2010;8(1):e1000298.
- Li C, Zhang Y, Cheng X, et al. PINK1 and PARK2 suppress pancreatic tumorigenesis through control of mitochondrial iron-mediated immunometabolism. Dev Cell. 2018;46(4):441–455. e8.
- Basit F, Van Oppen LM, Schöckel L, et al. Mitochondrial complex I inhibition triggers a mitophagy-dependent ROS increase leading to necroptosis and ferroptosis in melanoma cells. Cell Death Dis. 2017;8(3):e2716-e–e2716.
- Chang L-C, Chiang S-K, Chen S-E, et al. Heme oxygenase-1 mediates Bay 11–7085 induced ferroptosis. Cancer Lett. 2018;416:124–137.
- Gao M, Yi J, Zhu J, et al. Role of mitochondria in ferroptosis. Mol Cell. 2019;73(2):354–363. e3.
- Dice JF. Chaperone-mediated autophagy. Autophagy. 2007;3(4):295–299.
- Wu Z, Geng Y, Lu X, et al. Chaperone-mediated autophagy is involved in the execution of ferroptosis. Proc Natl Acad Sci U S A. 2019;116(8):2996–3005.
- Tang D, Chen X, Kang R, et al. Ferroptosis: molecular mechanisms and health implications. Cell Res. 2021;31(2):107–125.
- Yu Y, Xie Y, Cao L, et al. The ferroptosis inducer erastin enhances sensitivity of acute myeloid leukemia cells to chemotherapeutic agents. Mol Cell Oncol. 2015;2(4):e1054549.
- Cao JY, Dixon SJ. Mechanisms of ferroptosis. Cell Mol Life Sci. 2016;73(11-12):2195–2209.
- Kahlson MA, Dixon SJ. Copper-induced cell death. Science. 2022;375(6586):1231–1232.
- Li Z, Ferguson L, Deol KK, et al. Ribosome stalling during selenoprotein translation exposes a ferroptosis vulnerability. Nat Chem Biol. 2022;18(7):751–761.
- Poltorack CD, Dixon SJ. Understanding the role of cysteine in ferroptosis: progress & paradoxes. Febs J. 2022;289(2):374–385.
- Peng Q, Liu H, Luo Z, et al. Effect of autophagy on ferroptosis in foam cells via Nrf2. Mol Cell Biochem. 2022;477(5):1597–1606.
- Wang J, Deng B, Liu Q, et al. Pyroptosis and ferroptosis induced by mixed lineage kinase 3 (MLK3) signaling in cardiomyocytes are essential for myocardial fibrosis in response to pressure overload. Cell Death Dis. 2020;11(7):574.
- Jiang L, Kon N, Li T, et al. Ferroptosis as a p53-mediated activity during tumour suppression. Nature. 2015;520(7545):57–62.
- Tarangelo A, Magtanong L, Bieging-Rolett KT, et al. p53 suppresses metabolic stress-induced ferroptosis in cancer cells. Cell Rep. 2018;22(3):569–575.
- Gao M, Monian P, Quadri N, et al. Glutaminolysis and transferrin regulate ferroptosis. Mol Cell. 2015;59(2):298–308.
- Ou Y, Wang S-J, Li D, et al. Activation of SAT1 engages polyamine metabolism with p53-mediated ferroptotic responses. Proc Natl Acad Sci U S A. 2016;113(44):E6806–E6812.
- Yu H, Guo P, Xie X, et al. Ferroptosis, a new form of cell death, and its relationships with tumourous diseases. J Cell Mol Med. 2017;21(4):648–657.
- Li W, Luo L-X, Zhou Q-Q, et al. Phospholipid peroxidation inhibits autophagy via stimulating the delipidation of oxidized LC3-PE. Redox Biol. 2022;55:102421.
- Lee Y-S, Lee D-H, Choudry HA, et al. Ferroptosis-induced endoplasmic reticulum stress: cross-talk between ferroptosis and apoptosis. Mol Cancer Res. 2018;16(7):1073–1076.
- Sumpter R, Jr, Levine B. Autophagy and innate immunity: triggering, targeting and tuning. Semin Cell Dev Biol. 2010;21(7):699–711. editors Elsevier.
- Wen Q, Liu J, Kang R, et al. The release and activity of HMGB1 in ferroptosis. Biochem Biophys Res Commun. 2019;510(2):278–283.
- Xu T, Jiang L, Wang Z. The progression of HMGB1-induced autophagy in cancer biology. Onco Targets Ther. 2019;12:365–377.
- Lv X, Dong M, Tang W, et al. Ferroptosis, novel therapeutics in asthma. Biomed Pharmacother. 2022;153:113516.
- Lee HK, Lund JM, Ramanathan B, et al. Autophagy-dependent viral recognition by plasmacytoid dendritic cells. Science. 2007;315(5817):1398–1401.
- Tal MC, Sasai M, Lee HK, et al. Absence of autophagy results in reactive oxygen species-dependent amplification of RLR signaling. Proc Natl Acad Sci U S A. 2009;106(8):2770–2775.
- Jounai N, Takeshita F, Kobiyama K, et al. The Atg5–Atg12 conjugate associates with innate antiviral immune responses. Proc Natl Acad Sci U S A. 2007;104(35):14050–14055.
- Saitoh T, Fujita N, Hayashi T, et al. Atg9a controls dsDNA-driven dynamic translocation of STING and the innate immune response. Proc Natl Acad Sci U S A. 2009;106(49):20842–20846.
- Biswas D, Qureshi OS, Lee W-Y, et al. ATP-induced autophagy is associated with rapid killing of intracellular mycobacteria within human monocytes/macrophages. BMC Immunol. 2008;9(1):35.
- Scherz-Shouval R, Shvets E, Fass E, et al. Reactive oxygen species are essential for autophagy and specifically regulate the activity of Atg4. Embo J. 2007;26(7):1749–1760.
- Chen X, Xu S, Zhao C, et al. Role of TLR4/NADPH oxidase 4 pathway in promoting cell death through autophagy and ferroptosis during heart failure. Biochem Biophys Res Commun. 2019;516(1):37–43.
- Jonckheere N, Van Seuningen I. Fine-tuning autophagy in pancreatic adenocarcinoma: full blockage is required. Ann Transl Med. 2019;7(Suppl 1):S43–S43.
- Akalay I, Janji B, Hasmim M, et al. Epithelial-to-mesenchymal transition and autophagy induction in breast carcinoma promote escape from T-cell–mediated lysis. Cancer Res. 2013;73(8):2418–2427.
- Viswanathan VS, Ryan MJ, Dhruv HD, et al. Dependency of a therapy-resistant state of cancer cells on a lipid peroxidase pathway. Nature. 2017;547(7664):453–457.
- Brown CW, Amante JJ, Goel HL, et al. The α6β4 integrin promotes resistance to ferroptosis. J Cell Biol. 2017;216(12):4287–4297.
- Yang W-H, Huang Z, Wu J, et al. A TAZ–ANGPTL4–NOX2 axis regulates ferroptotic cell death and chemoresistance in epithelial ovarian CancerTAZ promotes ferroptosis in OvCa. Mol Cancer Res. 2020;18(1):79–90.
- Li J, Liu J, Xu Y, et al. Tumor heterogeneity in autophagy-dependent ferroptosis. Autophagy. 2021;17(11):3361–3374.
- Sharma P, Hu-Lieskovan S, Wargo JA, et al. Primary, adaptive, and acquired resistance to cancer immunotherapy. Cell. 2017;168(4):707–723.
- Efimova I, Catanzaro E, Van der Meeren L, et al. Vaccination with early ferroptotic cancer cells induces efficient antitumor immunity. J Immunother Cancer. 2020;8(2):e001369.
- Tang D, Kepp O, Kroemer G. Ferroptosis becomes immunogenic: implications for anticancer treatments. Oncoimmunology. 2021;10(1):1862949.
- Galluzzi L, Vitale I, Warren S, et al. Consensus guidelines for the definition, detection and interpretation of immunogenic cell death. J Immunother Cancer. 2020;8(1):e000337.
- Luo X, Gong H-B, Gao H-Y, et al. Oxygenated phosphatidylethanolamine navigates phagocytosis of ferroptotic cells by interacting with TLR2. Cell Death Differ. 2021;28(6):1971–1989.
- White E, Lattime EC, Guo JY. Autophagy regulates stress responses, metabolism, and anticancer immunity. Trends Cancer. 2021;7(8):778–789.
- Abudu YP, Pankiv S, Mathai BJ, et al. NIPSNAP1 and NIPSNAP2 act as “eat me” signals to allow sustained recruitment of autophagy receptors during mitophagy. Autophagy. 2019;15(10):1845–1847.
- Quezada SA, Peggs KS, Curran MA, et al. CTLA4 blockade and GM-CSF combination immunotherapy alters the intratumor balance of effector and regulatory T cells. J Clin Invest. 2006;116(7):1935–1945.
- Alissafi T, Banos A, Boon L, et al. Tregs restrain dendritic cell autophagy to ameliorate autoimmunity. J Clin Invest. 2017;127(7):2789–2804.
- Bai D, Feng H, Yang J, et al. Genomic analysis uncovers prognostic and immunogenic characteristics of ferroptosis for clear cell renal cell carcinoma. Mol Ther Nucleic Acids. 2021;25:186–197.
- Liu J, Zhang Z, Zhang W, et al. Ferroptosis mediation patterns reveal novel tool to implicate immunotherapy and multi-omics characteristics in bladder cancer. Front Cell Dev Biol. 2022;10:791630.
- Kato H, Perl A. Blockade of treg cell differentiation and function by the interleukin-21–mechanistic target of rapamycin axis via suppression of autophagy in patients with systemic lupus erythematosus. Arthritis Rheumatol. 2018;70(3):427–438.
- Oweida A, Hararah MK, Phan A, et al. Resistance to radiotherapy and PD-L1 blockade is mediated by TIM-3 upregulation and regulatory T-cell infiltration. Clin Cancer Res. 2018;24(21):5368–5380.
- Mahmutovic Persson I, Menzel M, Ramu S, et al. IL-1β mediates lung neutrophilia and IL-33 expression in a mouse model of viral-induced asthma exacerbation. Respir Res. 2018;19(1):16.
- Xu C, Sun S, Johnson T, et al. The glutathione peroxidase Gpx4 prevents lipid peroxidation and ferroptosis to sustain Treg cell activation and suppression of antitumor immunity. Cell Rep. 2021;35(11):109235.
- Li C-W, Lim S-O, Chung EM, et al. Eradication of triple-negative breast cancer cells by targeting glycosylated PD-L1. Cancer Cell. 2018;33(2):187–201. e10.
- Yao H, Lan J, Li C, et al. Inhibiting PD-L1 palmitoylation enhances T-cell immune responses against tumours. Nat Biomed Eng. 2019;3(4):306–317.
- Wang H, Yao H, Li C, et al. HIP1R targets PD-L1 to lysosomal degradation to alter T cell–mediated cytotoxicity. Nat Chem Biol. 2019;15(1):42–50.
- Zhou X, Zou L, Liao H, et al. Abrogation of HnRNP L enhances anti-PD-1 therapy efficacy via diminishing PD-L1 and promoting CD8+ T cell-mediated ferroptosis in castration-resistant prostate cancer. Acta Pharm Sin B. 2022;12(2):692–707.
- Zhou X, Li Q, He J, et al. HnRNP-L promotes prostate cancer progression by enhancing cell cycling and inhibiting apoptosis. Oncotarget. 2017;8(12):19342–19353.
- Abusamra AJ, Zhong Z, Zheng X, et al. Tumor exosomes expressing Fas ligand mediate CD8+ T-cell apoptosis. Blood Cells Mol Dis. 2005;35(2):169–173.
- Ning Y, Shen K, Wu Q, et al. Tumor exosomes block dendritic cells maturation to decrease the T cell immune response. Immunol Lett. 2018;199:36–43.
- Zhao X, Yuan C, Wangmo D, et al. Tumor-secreted extracellular vesicles regulate T-cell costimulation and can be manipulated to induce tumor-specific T-cell responses. Gastroenterology. 2021;161(2):560–574. e11.
- Jaiswal S, Jamieson CH, Pang WW, et al. CD47 is upregulated on circulating hematopoietic stem cells and leukemia cells to avoid phagocytosis. Cell. 2009;138(2):271–285.
- Casey SC, Tong L, Li Y, et al. MYC regulates the antitumor immune response through CD47 and PD-L1. Science. 2016;352(6282):227–231.
- Kamerkar S, LeBleu VS, Sugimoto H, et al. Exosomes facilitate therapeutic targeting of oncogenic KRAS in pancreatic cancer. Nature. 2017;546(7659):498–503.
- Colombo M, Raposo G, Théry C. Biogenesis, secretion, and intercellular interactions of exosomes and other extracellular vesicles. Annu Rev Cell Dev Biol. 2014;30(1):255–289.
- Bhattacharya S, Pal K, Sharma AK, et al. GAIP interacting protein C-terminus regulates autophagy and exosome biogenesis of pancreatic cancer through metabolic pathways. PLOS One. 2014;9(12):e114409.
- Guo H, Chitiprolu M, Roncevic L, et al. Atg5 disassociates the V1V0-ATPase to promote exosome production and tumor metastasis independent of canonical macroautophagy. Dev Cell. 2017;43(6):716–730. e7.
- Xie L, Li J, Wang G, et al. Phototheranostic metal-phenolic networks with antiexosomal PD-L1 enhanced ferroptosis for synergistic immunotherapy. J Am Chem Soc. 2022;144(2):787–797.
- Xiong A, Duan L, Chen J, et al. Flt3L combined with rapamycin promotes cardiac allograft tolerance by inducing regulatory dendritic cells and allograft autophagy in mice. 2012;7(10):e46230.
- Zhu Y, Knolhoff BL, Meyer MA, et al. CSF1/CSF1R blockade reprograms tumor-infiltrating macrophages and improves response to T-cell checkpoint immunotherapy in pancreatic cancer ModelsCSF1R blockade improves checkpoint immunotherapy. Cancer Res. 2014;74(18):5057–5069.
- Dai E, Han L, Liu J, et al. Autophagy-dependent ferroptosis drives tumor-associated macrophage polarization via release and uptake of oncogenic KRAS protein. Autophagy. 2020;16(11):2069–2083.
- Jiang Z, Lim S-O, Yan M, et al. TYRO3 induces anti–PD-1/PD-L1 therapy resistance by limiting innate immunity and tumoral ferroptosis. J Clin Invest. 2021;131(8):e139434.
- Sena LA, Li S, Jairaman A, et al. Mitochondria are required for antigen-specific T cell activation through reactive oxygen species signaling. Immunity. 2013;38(2):225–236.
- Wang Y, Tian Q, Hao Y, et al. The kinase complex mTORC2 promotes the longevity of virus-specific memory CD4+ T cells by preventing ferroptosis. Nat Immunol. 2022;23(2):303–317.
- Xia H, Wang W, Crespo J, et al. Suppression of FIP200 and autophagy by tumor-derived lactate promotes naive T cell apoptosis and affects tumor immunity. Sci Immunol. 2017;2(17):eaan4631.
- Hubbard VM, Valdor R, Patel B, et al. Macroautophagy regulates energy metabolism during effector T cell activation. J Immunol. 2010;185(12):7349–7357.
- Botbol Y, Patel B, Macian F. Common γ-chain cytokine signaling is required for macroautophagy induction during CD4+ T-cell activation. Autophagy. 2015;11(10):1864–1877.
- Garg SK, Yan Z, Vitvitsky V, et al. Differential dependence on cysteine from transsulfuration versus transport during T cell activation. Antioxid Redox Signal. 2011;15(1):39–47.
- Levring TB, Hansen AK, Nielsen BL, et al. Activated human CD4+ T cells express transporters for both cysteine and cystine. Sci Rep. 2012;2(1):266.
- Michalek RD, Gerriets VA, Jacobs SR, et al. Cutting edge: distinct glycolytic and lipid oxidative metabolic programs are essential for effector and regulatory CD4+ T cell subsets. J Immunol. 2011;186(6):3299–3303.
- Pua HH, Guo J, Komatsu M, et al. Autophagy is essential for mitochondrial clearance in mature T lymphocytes. J Immunol. 2009;182(7):4046–4055.
- Singh R, Kaushik S, Wang Y, et al. Autophagy regulates lipid metabolism. Nature. 2009;458(7242):1131–1135.
- Xu X, Araki K, Li S, et al. Autophagy is essential for effector CD8+ T cell survival and memory formation. Nat Immunol. 2014;15(12):1152–1161.
- Carleton G, Lum JJ. Autophagy metabolically suppresses CD8+ T cell antitumor immunity. Autophagy. 2019;15(9):1648–1649.
- Wang W, Green M, Choi JE, et al. CD8+ T cells regulate tumour ferroptosis during cancer immunotherapy. Nature. 2019;569(7755):270–274.
- Miller BC, Zhao Z, Stephenson LM, et al. The autophagy gene ATG5 plays an essential role in B lymphocyte development. Autophagy. 2008;4(3):309–314.
- Muri J, Thut H, Bornkamm GW, et al. B1 and marginal zone B cells but not follicular B2 cells require Gpx4 to prevent lipid peroxidation and ferroptosis. Cell Rep. 2019;29(9):2731–2744. e4.
- Pengo N, Scolari M, Oliva L, et al. Plasma cells require autophagy for sustainable immunoglobulin production. Nat Immunol. 2013;14(3):298–305.
- Yao Y, Chen Z, Zhang H, et al. Selenium–GPX4 axis protects follicular helper T cells from ferroptosis. Nat Immunol. 2021;22(9):1127–1139.
- Zhang Y, Morgan MJ, Chen K, et al. Induction of autophagy is essential for monocyte-macrophage differentiation. Blood, JAm Soc Hemato. 2012;119(12):2895–2905.
- Handa P, Thomas S, Morgan-Stevenson V, et al. Iron alters macrophage polarization status and leads to steatohepatitis and fibrogenesis. J Leukoc Biol. 2019;105(5):1015–1026.
- Zhou Y, Que KT, Zhang Z, et al. Iron overloaded polarizes macrophage to proinflammation phenotype through ROS/acetyl-p53 pathway. Cancer Med. 2018;7(8):4012–4022.
- Chen W, Ma T, Shen X-N, et al. Macrophage-induced tumor angiogenesis is regulated by the TSC2–mTOR pathwaytsc2–mTOR regulates macrophage-induced tumor angiogenesis. Cancer Res. 2012;72(6):1363–1372.
- Chang C-P, Su Y-C, Lee P-H, et al. Targeting NFKB by autophagy to polarize hepatoma-associated macrophage differentiation. Autophagy. 2013;9(4):619–621.
- Chang C, Su Y, Hu C, et al. TLR2-dependent selective autophagy regulates NF-κB lysosomal degradation in hepatoma-derived M2 macrophage differentiation. Cell Death Differ. 2013;20(3):515–523.
- Roca H, Varsos ZS, Sud S, et al. CCL2 and interleukin-6 promote survival of human CD11b + peripheral blood mononuclear cells and induce M2-type macrophage polarization. J Biol Chem. 2009;284(49):34342–34354.
- Jiang G-M, Tan Y, Wang H, et al. The relationship between autophagy and the immune system and its applications for tumor immunotherapy. Mol Cancer. 2019;18(1):17.
- Folgiero V, Miele E, Carai A, et al. IDO1 involvement in mTOR pathway: a molecular mechanism of resistance to mTOR targeting in medulloblastoma. Oncotarget. 2016;7(33):52900–52911.