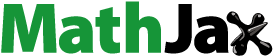
Abstract
Carbon nanotube (CNT) in vivo inhalation studies are increasingly providing estimates of the quantity of material deposited in the lung, generally estimated using standard formulae and pulmonary deposition models. These models have typically been developed and validated using data from studies using sphere-like particles. Given the importance of particle morphology to pulmonary deposition, the appropriateness of such an approach was explored to identify any potential limitations. Aerosolized CNT particles typically form ‘fiber-like’ and/or ‘broadly spherical’ agglomerates. A review of currently used deposition models indicates that none have been directly validated against results for CNT, however, models for spherical particles have been extensively validated against a wide range of particle sizes and materials and are thus expected to provide reasonable estimates for most ‘broadly spherical’ CNT particles, although experimental confirmation of this would be of benefit, especially given their low density. The validation of fiber deposition models is significantly less extensive and, in general, focused on larger particles, e.g. asbestos. This raises concerns about the accuracy of deposition estimates for ‘fiber-like’ CNT particles and recommendations are made for future research to address this. An appreciation of the uncertainties on CNT deposition estimates is important for their interpretation and thus it is recommended that model sensitivity and uncertainty assessments be undertaken. Issues surrounding the measurement and derivation of model input data are also addressed, including instrument responses and particle density assessment options. Recommendations are also made for aerosol characterization to ‘future-proof’ CNT inhalation studies regarding advances in deposition modeling and toxicological understanding.
Introduction
There is a growing consensus on the importance of providing information on the amount of material deposited in the lung during in vivo inhalation studies using particulates, reflected by the inclusion in the recently revised OECD inhalation test guidelines TG412 (OECD Citation2017a) and TG413 (OECD Citation2017b) of a recommendation for lung burden measurements to be undertaken for insoluble materials. Information on the quantity of an inhaled material deposited in the lung and its temporal variation post-exposure can give an indication of changes in clearance mechanisms, such as those indicative of so called ‘lung overload,’ potentially important for interpreting toxicity study results (ECETOC Citation2013). But estimates of the quantities of material deposited in the lung also serve other important purposes, including allowing clearer inter-comparison between studies, and, importantly, ‘extrapolation’ between animal and human models for risk assessment purposes (Jarabek et al. Citation2005). An increasing number of published in vivo inhalation studies using particulate aerosols, particularly nanomaterials, therefore provide information on the quantity of deposited material, either measured using techniques such as ICP-MS (Schwotzer et al. Citation2017) or, more frequently, estimated using standard formulae and available models (Shin et al. Citation2015). Such models have typically been developed and validated using data from studies using spherical or almost spherical particles, however, they are increasingly being used to estimate lung deposition for fibrous materials, in particular carbon nanotubes (CNT). Given the significance of particle morphology to pulmonary deposition (Crowde et al. Citation2002), it is necessary to explore whether such models are appropriate for this purpose. This is of particular importance because of the growing number of in vivo inhalation studies using such materials being undertaken in response to concerns regarding their potential toxicity. For example, there are concerns that exposure to some types of CNT may lead to some of the diseases associated with exposure to asbestos (van Berlo et al. Citation2012; Donaldson et al. Citation2013; Ellenbecker et al. Citation2018; Dong and Ma Citation2019) and IARC (Citation2017) has recently classified one particular type of multi-walled carbon nanotube (MWCNT), MWCNT-7, as possibly carcinogenic in humans (Class 2B).
Here, we summarize current understanding of the physical characteristics of aerosolized CNT particles (both as identified in industrial settings and as used in in vivo inhalation studies) pertinent to transport and deposition in the respiratory tract, with a particular focus on morphology and density. Relevant deposition mechanisms are briefly outlined, and their relative importance for different CNT aerosol particle types is discussed. Current particle (including fiber) deposition models are reviewed with reference to their validation ‘status’ for CNT aerosols. The practical application of such models to CNT aerosols is then addressed, with particular reference to the complex nature of such aerosols and the concomitant difficulty in assigning appropriate parameter values to adequately represent aerosols for input to models primarily designed for use with spherical particles. In this context, we also discuss some issues associated with the measurement of CNT aerosol particle characteristics for input to such models. Finally, we make recommendations on the aerosol characterization information to be collected during CNT in vivo inhalation experiments to improve inter-study comparability and identify areas where future research is required to improve our understanding of the transport and deposition of CNT aerosol particles in the respiratory tract and thus the accuracy and robustness of lung deposition estimates.
Carbon nanotube aerosol characteristics
Particle shape
Unlike the conception of CNT as long, thin, needlelike fibers, somewhat like crocidolite asbestos, the majority of airborne CNT particles identified in electron micrographs after sampling in real exposure situations are bent and tangled, forming low density agglomerates up to tens of micrometers in diameter. summarizes key findings relating to CNT aerosol particle properties from selected (mainly workplace) exposure assessment studies, whilst more general reviews of workplace, consumer and/or environmental exposures to CNT can be found in the literature (Canu et al. Citation2016; Dahm et al. Citation2018). A wide range of particle types have been observed, varying with activity, CNT type, production method, and functionalization. Morphologically, airborne CNT particles can be grouped into two general categories, ‘fiber-like’ and ‘broadly-spherical,’ the latter being more common, whilst noting that ‘real’ CNT aerosols may consist of a complex mix of both forms and/or intermediate forms e.g. ‘fiber-like’ particles with branches, or ‘broadly-spherical’ particles with single fibers protruding from the body.
Table 1. Characteristics of CNT aerosols sampled in various exposure situations.
‘Fiber-like’ particles are either individual, or lightly agglomerated groups of small numbers of, tubes typically of length 1–6 µm and diameter ∼50 nm. The lengths of aerosolized MWCNT are generally shorter than those characterized in ‘bulk’ i.e. as manufactured, implying that shorter, or broken, MWCNT may preferentially be aerosolized as ‘fiber-like’ particles compared to longer MWCNT which tend to appear as ‘broadly-spherical’ (or ‘isometric’) agglomerates (Chen et al. Citation2012). Compared with asbestos fibers, typically a few to tens micrometers in length and around 500 nm in diameter, ‘fiber-like’ CNT are smaller, therefore their aerodynamic behavior and respiratory deposition characteristics are likely to differ.
‘Broadly-spherical’ particles are open agglomerated structures containing tangled bunches of tubes. Single-walled carbon nanotubes (SWCNT) have a strong tendency to form ‘broadly-spherical’ particles, with a large range of sizes (100 nm up to hundreds of micrometers or more) observed (Maynard et al. Citation2004). ‘Broadly-spherical’ MWCNT particles have also been observed, with sizes generally smaller than corresponding SWCNT particles (100 nm up to a few µm) (Ihrie et al. Citation2019).
Aerosolization of CNT embedded within a matrix of other material from a ‘composite’ product is also possible (Nowack et al. Citation2013; Kovochich et al. Citation2018), however, release potential outside of production processes (i.e. during normal use) for a range of composites is expected to be low (Kingston et al. Citation2014) and released CNT are typically not free but ‘composite-bound’ (i.e. embedded and protruding from matrix particles). Such particles will have different morphology (and hence aerodynamic behavior) and may have different toxicity compared to ‘free CNT’ aerosols (Ging et al. Citation2014) and are not considered further here.
For inhalation studies bulk samples of carbon nanotubes can be aerosolized using a number of devices, including acoustic generators (McKinney et al. Citation2009), vortex shakers (Ku et al. Citation2013), dust mills, feeders or brush generators (Ma-Hock et al. Citation2009; Kasai et al. Citation2014), ultrasonic generators (Kim et al. Citation2017) and nebulizers or atomizers (Chortarea et al. Citation2015; Kadoya et al. Citation2016). The aerosol particles produced can be grouped into the same broad shape categories as indicated above but the nature of the aerosolization process, including the apparatus but also the environmental conditions e.g. humidity, may significantly affect the properties of the resulting aerosol (Ku et al. Citation2013; Ku and Kulkarni Citation2015; Pauluhn and Rosenbruch Citation2015; Ku and Birch Citation2019). The characteristics of the aerosol used for inhalation studies may therefore differ significantly from that which would be encountered in a workplace using the same material. This is an important factor to consider in the risk management process. In general, predicting the characteristics of airborne CNT particles from information on their bulk material properties (Jackson et al. Citation2015; Poulsen et al. Citation2017) is not straightforward (see Supplementary Information for further discussion).
Particle density
In addition to morphology, particle density is an important parameter in determining airborne CNT aerodynamic behavior and hence also pulmonary deposition characteristics. The range of observed airborne CNT particle densities is significant due to the wide variety of forms, with the lowest densities well below those of most other aerosol particles of relevance for human exposure. The density of individual CNT depends on the number of walls and the fiber diameter (Laurent et al. Citation2010) and the presence (if any) of ‘impurities’ e.g. metal catalysts, or functional groups. For previous studies using MWCNT, this ‘intrinsic’ density ranges from around 1.7 to 2.2 g cm−3 (Kim et al. Citation2009; Oyabu et al. Citation2011; Chen et al. Citation2012; Ku and Kulkarni Citation2015; Wang et al. Citation2015), which may correspond approximately to that expected for ‘fiber-like’ CNT particles. By comparison, the density of graphite is around 2.2 g cm−3, carbon black ranges between 1.8 and 2.1 g cm−3, and airborne asbestos particles approximately 2.5 g cm−3 (HEI Citation1991). ‘Broadly-spherical’ CNT agglomerates, however, may have significantly lower ‘effective densities’ (see Supplementary Information for a fuller description of this term), in the range 0.1–1.0 g cm−3, especially for larger agglomerates (Ku and Kulkarni Citation2015; Wang et al. Citation2015; Gaté et al. Citation2019; Ku and Birch Citation2019), with one study using aerosolized SWCNT estimating a density of the order of 0.01 g cm−3 (Baron et al. Citation2008). Particle effective densities depend on a number of factors including the CNT production method, bulk form and properties (Hao et al. Citation2003; Maynard et al. Citation2004; Ku et al. Citation2006; Chen et al. Citation2012; Pauluhn and Rosenbruch Citation2015; Wang et al. Citation2015; Ku and Birch Citation2019). The practical challenges involved in determining the density of airborne CNT particles are discussed in a later section.
Aerosol heterogeneity
In addition to the varying characteristics of CNT aerosols produced via different methods, the characteristics of a given CNT aerosol can inherently reflect a range of particle shapes, sizes and densities, and, as such, they have the potential to be significantly more heterogeneous than other aerosol types. For example, whilst clearly not homogeneous, spherical nanoparticles typically form broadly spherical aerosol agglomerates and asbestos aerosols generally comprise rigid fibers of similar density. By contrast, CNT themselves often have a wide range in both tube diameter and length (JRC Citation2014; Poulsen et al. Citation2017), and when aerosolized, can agglomerate irregularly, forming a wide variety of shapes (e.g. McKinney et al. Citation2009; Porter et al. Citation2012; Gaté et al. Citation2019), with resulting large range in aspect ratio (ratio of length to width) and dynamic shape factor (discussed in Supplementary Information), and a corresponding wide range in density. This means that, for instance, one subset of the CNT aerosol population may behave aerodynamically very differently to others, and consequently may reach different regions of the lung. The different location of deposition, along with other characteristics of the aerosol (e.g. aggregation state, presence of fibers etc.) could result in different health outcomes (Ellenbecker et al. Citation2018).
Airborne transport and deposition of CNT aerosols in the lung
Five main mechanisms are responsible for aerosol deposition in lung airways upon inhalation: inertial impaction, gravitational sedimentation, Brownian diffusion, interception, and electrostatic forces. The relative importance of each depends on both particle properties, which influence aerodynamic behavior, and airway geometry and air flow characteristics. For example, there are well-known effects on deposition due to age, size, sex and physical activity-related differences in airway geometry and air flow characteristics (ICRP Citation1994, NCRP Citation1997, Hussein et al. Citation2013) and also between different animal models, e.g. mouse and rat (Anjilvel and Asgharian Citation1995, Miller et al. Citation2016). In addition, deposition may also be altered due to differences in airway structure and air flow characteristics in people with conditions such as chronic obstructive pulmonary disease (COPD) or asthma (Chalupa et al. Citation2004, Jakobsson et al. Citation2018), although this is much less well understood. These effects are relevant to all inhaled particles and thus are not discussed in detail here, although their impact for CNT will be considered in following sections.
Impaction occurs when particles with sufficient inertia deviate from the gas streamline as the latter curves e.g. at a bend or bifurcation in the lung, and deposit on airway walls. Impaction is most important for large particles and higher air flow rates and is of primary concern in the upper airways. Sedimentation is the result of gravitational settling of particles and is more important in smaller airways, where flow velocity is lower and particle residence time is longer. Both these mechanisms are governed by the aerodynamic equivalent diameter of the particle (see Supplementary Information). A very large range of aerodynamic diameters, from 10 nm to over 100 μm, is obtained for ‘broadly-spherical’ CNT particles using typically observed dimensions and densities (). Because the density of ‘broadly-spherical’ CNT particles is usually expected to be below unit density, their aerodynamic diameter is likely to be smaller than their physical diameter.
Table 2. Predicted aerodynamic diameters (in μm) of spherical particles with diameters and densities in the ranges observed for ‘broadly-spherical’ CNT particles (details of derivation in Supplementary Information) (values in brackets are the ratio of physical to aerodynamic diameter).
Diffusional deposition occurs as a result of collisions between gas molecules and airborne particles, leading to a higher probability that particles will contact nearby airway walls, and is governed by the particle diffusion equivalent diameter (see Supplementary Information). Diffusion is most important for small particles (<300 nm) and in small airways and the alveolar region, with low flow velocity and long residence times. Diffusion equivalent diameters for ‘broadly-spherical’ MWCNT agglomerates are typically in the range 100 nm to 100 µm ().
Table 3. Aerodynamic and diffusion diameters and expected deposition mechanism(s) for different groups of CNT and asbestos particles.
Although lung deposition models are described in more detail later, it is instructive to introduce some generalizations regarding the link between ‘diameter’ and deposition efficiency. As outlined, diffusion-related mechanisms dominate for smaller particles, hence for particles with (diffusion) diameter <300 nm, decreasing diameter leads to an increase in deposition efficiency. Conversely, above this diameter, aerodynamic-related mechanisms dominate, and for particles with (aerodynamic) diameter >300 nm, deposition efficiency increases with diameter, up to the point where particle inhalability (the ability of a particle to enter the head; Millage et al. Citation2010) becomes a factor, usually around 3 µm in rats and around 10 µm in humans (Menache et al. Citation1995). Typical ‘broadly-spherical’ CNT agglomerates are expected to deposit primarily by aerodynamic, rather than diffusional, processes ().
Formulae have been developed for the aerodynamic and diffusion diameters of non-spherical particles, including fibers (see Supplementary Information). As a ‘rule of thumb,’ aerodynamic diameters are 2.5–4 times larger than fiber diameters, with only minor dependence on fiber length. With typical aerodynamic diameters 40–340 nm, expected deposition via impaction and sedimentation is relatively low for most ‘fiber-like’ CNT particles. Diffusion diameters for ‘fiber-like’ CNT particles have a wider range than aerodynamic diameters, approximately 50 nm to 1 µm, compared with 300 nm up to several microns for asbestos fibers. This means that, unlike asbestos which is not likely to deposit effectively via diffusional methods, fiber-like CNT are likely to exhibit increasing diffusional deposition with decreasing fiber diameter and length ().
The above discussion assumes simplistic model representations of the two generalized particle forms identified. The relevance of the conclusions to real particles, including, for example, single fibers that might not be straight or small groups of fibers with different orientations (Oyabu et al. Citation2011, Chen et al. Citation2012, Kasai et al. Citation2016, Ihrie et al. Citation2019), or agglomerates which are clearly non-spherical or irregular with significant non-uniform densities (Mitchell et al. Citation2007, Porter et al. Citation2012, Ihrie et al. Citation2019), as well as particles which exhibit characteristics of both the two generalized forms (e.g. ‘broadly-spherical’ particles with long protruding tails, Pauluhn Citation2010, Wang et al. Citation2015; ‘fiber-like’ particles with apparent branches, Oyabu et al. Citation2011, Stapleton et al. Citation2012); or agglomerated fibers with shapes between those of ‘broadly-spherical’ and ‘fiber-like,’ Ryman-Rasmussen et al. Citation2009, Chen et al. Citation2012) remains to be elucidated. These differences in morphology will alter the relationships described above, introducing errors into the assumed relationship between diffusion and aerodynamic diameter and determining orientation effects in air flow and hence also influence lung deposition. The wide variability in CNT particle morphologies described above is also reflected in images of lung-deposited CNT. For example, Mercer et al. (Citation2013) showed that for ‘fiber-like’ CNT the majority of initial lung burden of MWNT-7 in mice was from particles with 4 or more fibers, and Ku et al. (Citation2006) showed fibers agglomerating in alignment with each other; whereas Kasai et al. (Citation2016), using a different aerosol generation method, observed individual, un-aggregated MWNT-7 fibers in pleural and abdominal lavage fluid (although this may reflect the effect of de-agglomeration post deposition). Tangled MWCNT have also been observed in the pleura of mice following nose-only inhalation (Ryman-Rasmussen et al. Citation2009). The exact effects on aerodynamic behavior and deposition are likely to differ depending on the material, generation method and possibly also target concentration in exposure studies, and may vary also with eventual airborne particle size. Despite recent progress in this area (Chen et al. Citation2012, Citation2016a; Ku and Kulkarni Citation2015, Citation2018; Wang et al. Citation2015), further experimental studies focused on linking the physical properties and morphology of airborne CNT with their fundamental aerosol behavior, for commonly used CNT types, are still required to assess the validity of present approaches to, and/or develop a more accurate treatment of the aerodynamic behavior, and hence lung deposition, of ‘real-world’ CNT particles.
Other deposition mechanisms may also be important for CNT particles. Interception results from the particle touching the airway surface because of its physical size or shape, even when not deviating from gas streamlines through other mechanisms. For spherical or compact particles, interception efficiency is usually low. However, for high aspect ratio particles, increased length may increase the likelihood of the particle touching a surface. Interception could be a significant deposition mechanism for ‘fiber-like’ CNT particles (Bahk et al. Citation2013), leading to an increase in deposition efficiency both in the upper airways (as demonstrated by cast models, for irregularly shaped agglomerates e.g. Scheckman and McMurry Citation2011) and in smaller airways (as shown for asbestos fibers via experimental observation of preferential deposition at alveolar duct bifurcations in rats, Brody and Roe Citation1983). As air flow characteristics in the upper airways tend to align longer fibers with the air flow direction (Chen et al. Citation2016b), this could lead to penetration of long, ‘fiber-like’ CNT into smaller airways, where interception becomes much more likely. For ‘broadly-spherical particles, given that several TEM images show particles with visible ‘tails’ or protrusions (Mitchell et al. Citation2007; Pauluhn Citation2010; Pauluhn and Rosenbruch Citation2015); and noting also that they have relatively large physical size for a given aerodynamic diameter due to their low density, higher interception deposition efficiency for particles of this nature may also be expected, in particular as particles penetrate to smaller airways (although we note that Pauluhn (Citation2010) suggests that in their study, enhanced deposition via interception did not occur in rat upper airways for low-density MWCNT agglomerates). However, interception is not specifically included in the majority of deposition models, nor to our knowledge is there any quantitative experimental information on the specific contribution of interception to overall deposition efficiency for CNT particles.
Electrostatic charge-mediated deposition is often neglected in consideration of particle lung deposition. However, enhancement of deposition can occur for highly charged particles (Prodi and Mularoni Citation1985). The electrostatic charge state of airborne CNT particles could be higher than that of other, more compact particles (Baron et al. Citation2008; Nasibulin et al. Citation2008; Kulkarni et al. Citation2009). The concept of the ‘aerosol charge state’ (which is the probability of an aerosol particle of a given size carrying a given number of electronic charges, and is commonly assumed to be independent of particle composition in atmospheric physics; Wiedensohler Citation1988) differs from the property of particle ‘surface charge,’ which is intrinsic to the material and its interface with the surrounding medium, and is usually assessed in suspension via measurement of the related quantity, zeta potential (Porter et al. Citation2010, Zeinabad et al. Citation2016). Surface charge may significantly impact on toxicity of particles deposited in the lung (Fröhlich Citation2012), and may indirectly influence the size distribution of aerosolised CNT particles generated by nebulization through moderation of the stability and agglomeration state of the CNT material in suspension (Oberdörster and Kuhlbusch Citation2018). Dry generation procedures (as in, for example, acoustic generators or dust mills) may also lead to high levels of electrostatic charge on airborne CNT particles. However, the effects (if any) of aerosol charge on deposition of CNT particles have to date not been reported, and further investigation into typical electrostatic charge levels on both ‘broadly-spherical’ and ‘fiber-like’ CNT aerosol particles in a range of exposure situations (including generation methods commonly employed in in vivo studies), whether this is influenced by surface chemistry and/or zeta potential, and how this may affect deposition, is required.
Estimating deposited doses of inhaled CNT
Deposition efficiency and deposited dose
Accurate assessment of the deposited dose, not just exposure concentration, is crucial in comparing results of different toxicological studies and study types, in extrapolating these results to humans and, ultimately, in developing exposure limits to protect health (Oberdörster et al. Citation2015; Schmid and Cassee Citation2017; Ellenbecker et al. Citation2018). Deposited doses of inhaled particulate material, Dd (µg), are typically estimated using available models/software and formulae of the type
(1)
(1)
where C is the aerosol mass concentration (µg m−3), MV the minute ventilation of the animal (m3 min−1), t is the exposure duration (min) and Deff (dimensionless) is the fractional efficiency of lung deposition for the aerosol considered. Clearly any dose estimate has a degree of associated uncertainty. Frequently in such assessments, values from the literature or model defaults, rather than measured values, are used for MV, and this is one source of uncertainty in resultant predictions (Buckley et al. Citation2016); however, the major contribution arises from estimation of deposition efficiency.
In some studies which use the above method to estimate deposited CNT doses, values for deposition efficiency are simply taken from the literature (NIOSH 2013). Typical estimates for MWCNT total lung deposition efficiency range from 1 to 4% in mice, 5 to 20% in rats and 8 to 10% in humans (Kuempel et al. Citation2017), whilst a figure of 10% has been used in attempting to assess dose in several early CNT exposure studies for a broad particle size range (e.g. Mitchell et al. Citation2007 and Ryman-Rasmussen et al. Citation2009 in mice; Ma-Hock et al. Citation2009 in rats). In many cases there is a lack of clarity surrounding the relevance of the choice of deposition efficiency value used in such dose estimations, and without a quantitative justification the calculated ‘dose’ is merely a scaled exposure estimate, which alone does not provide sufficient information to compare between studies (Schmid and Cassee Citation2017).
Deposition efficiencies are increasingly being estimated using readily available models/software with input data related to the aerosol (e.g. particle size distribution and particle density) and the animal model (e.g. lung volumes and breathing rate). In particular, the Multiple Path Particle Dosimetry (MPPD) model (Anjilvel and Asgharian Citation1995; Asgharian and Anjilvel Citation1998; Miller et al. Citation2016; ARA Citation2018) has found increasing use for this purpose (O’Shaughnessy et al. Citation2014; Pauluhn and Rosenbruch Citation2015; Gaté et al. Citation2019; Lee et al. Citation2019). Nevertheless, whilst a clear improvement, there are still issues regarding the application of models to estimate deposition efficiency (and hence dose) in this manner within the literature. For example, reporting of the specific input parameter values used within the model, which are necessary to reproduce results, is frequently missing, incomplete or unclear, especially in older publications, although the importance of reporting these values is increasingly being recognized (Gaté et al. Citation2019; Lee et al. Citation2019). In addition, in some works there are variations between measured and actually utilized values e.g. O’Shaughnessy et al. (Citation2014) measured 121 nm CMD particles but used 100 nm in their model calculations, while Pauluhn and Rosenbruch (Citation2015) measured a density of 0.1–0.3 g cm−3 for ‘dry’ MWCNT but used 1.0 g cm−3 as input in the MPPD model, as they considered this better fitted their measured lung burdens. Uncertainties in the measurements of aerosol parameters used as input to deposition models, and their implications for deposition efficiency calculations and ultimately dose, are also generally ignored (this issue is discussed in more detail later). A more fundamental issue, however, is whether the available models are suitable for use in this context, and this is addressed below.
Applicability of existing whole-lung particle deposition models to CNT aerosols
The most widely used models to predict aerosol deposition are whole-lung models, often semi-empirical models utilizing simplified representations of lung structure and commonly derived from both theoretical consideration of particle deposition mechanisms and available experimental data. These models primarily address deposition of spherical particles in the whole respiratory tract or major constituent regions (e.g. alveolar region). Validation against additional experimental data for total, and to a lesser extent regional, deposition has been explicitly reported for some models (Hofmann Citation2011) and in general they predict the deposition of spherical particles reasonably well over a large diameter range (Kleinstreuer et al. Citation2008; Hofmann Citation2009; Rostami Citation2009). Examples include the ICRP Human Respiratory Tract Model (HRTM) model (ICRP Citation1994), MPPD (Anjilvel and Asgharian Citation1995; Miller et al. Citation2016), the NCRP model (NCRP Citation1997) and Hofmann (Citation2009). Although the more sophisticated models include particle shape factors or aspect ratios among their input variables, allowing for non-spherical particle deposition efficiency to be estimated, most are not intended specifically for assessing deposition of fibrous particles. To our knowledge none of these models have been validated against experimental deposition data for CNT aerosols, either ‘fiber-like’ or ‘broadly-spherical.’ Nevertheless, as they have typically been developed using experimental data for spherical particles and subsequently verified/validated against additional data for a wide range of materials and size and density ranges, these models are expected to provide a reasonable estimate, in most cases, for ‘broadly-spherical’ CNT particle deposition efficiency, although further studies providing experimental confirmation of this are recommended.
A large number of these models are flexible enough to explore the effects of some differences in size, age and activity level on CNT deposition as they include different airway models (or appropriate scaling factors) and allow a range of breathing parameter values to be used (ICRP Citation1994, NCRP Citation1997, Hofmann Citation2009, Anjilvel and Asgharian Citation1995, Miller et al. Citation2016. MPPD also usefully allows differences in deposition between a range of animal models to be explored. However, none of these models include airway configurations and air flow characteristics relevant to diseased lungs (e.g. COPD).
Although, as outlined, many models are not directly applicable to fibrous materials, by employing either measured or derived aerodynamic and diffusion equivalent diameters, estimates of deposition efficiency for ‘fiber-like’ CNT particles can be obtained from these models. For ‘fiber-like’ CNT particles, with expected aerodynamic and diffusion equivalent diameter ranges 40–400 nm and 50 nm–1 µm respectively (see Supplementary Information), such models predict that diffusion will be the dominant deposition mechanism in the alveolar region, with efficiency ranging from a few percent for the largest fibers, up to around 30% for the shortest and thinnest fibers. This is in contrast to ‘broadly-spherical’ CNT particles, and also to asbestos fibers which, with their much larger equivalent diameters, deposit in the alveoli mostly by aerodynamic, rather than diffusional, mechanisms, with efficiency approximately 10–20%. However, whilst this approach can give an indication of deposition levels, these estimates should be regarded with caution as such models rarely include deposition by interception and, more importantly, have not been validated against experimental data for fiber-like materials.
Fiber-specific lung deposition models and validation
Some models have been specifically developed to predict the deposition of fibers. Like more general models, these have developed from simple compartmental models (Harris and Fraser Citation1976) through single-path models (Asgharian and Yu Citation1988, Citation1989) to multiple-path (Asgharian and Anjilvel Citation1998) or stochastic (Sturm and Hofmann Citation2009; Sturm Citation2014, Citation2018) models. These are often based on the extension of existing models for spherical particles (Koblinger and Hofmann Citation1990; Anjilvel and Asgharian Citation1995) and are based on using aerodynamic and diffusion equivalent diameters to account for differences in diffusional, sedimentation and impaction deposition due to the different particle shape and behavior of fibers compared with spheres (using approaches such as those in Supplementary Information). Interception is also generally included as this could be a significant deposition mechanism for fibers. To account for enhanced interception of fibers, correction factors applied to individual lung generations (Sturm and Hofmann Citation2009) or direct incorporation into the expressions used to evaluate overall deposition efficiency (Asgharian and Anjilvel Citation1998; Asgharian and Yu Citation1988, Citation1989; Ding et al. Citation1997) have been employed. Deposition efficiencies associated with each mechanism may be derived either from an analytical standpoint (Asgharian and Yu Citation1989; Ding et al. Citation1997), Monte Carlo methods (Ding et al. Citation1997; Sturm and Hofmann Citation2009; Sturm Citation2018) and/or computational fluid dynamics (CFD) modeling (Balásházy et al. Citation2005; Chen et al. Citation2016b). Because of the complexity of CFD modeling, these studies often focus on the upper respiratory tract only (Tian and Ahmadi Citation2016), individual airways or bifurcations (Kleinstreuer and Feng Citation2013), or acinar regions only (Shachar-Berman et al. Citation2019), rather than the whole lung.
Unfortunately, experimental data both in vitro and in vivo (particularly in humans) against which to validate fibrous particle deposition models is limited, especially for smaller fibers and in the smaller airways, where such experiments are difficult to undertake. Experimental studies largely focus on asbestos and a small number of other materials including glass or ceramic fibers, falling into three broad categories: human postmortem (ex vivo) tissue sample analyses, airway cast model studies, and in vivo inhalation studies. Ex vivo studies of lung tissue (mainly from deceased asbestos workers) provide qualitative information on the penetration of various fiber types to different lung regions (Timbrell Citation1982). Although studies of this type cannot be used to derive deposition efficiencies, the ability of long fibers (tens of micrometers) to deposit deep within the lung was clearly shown. Airway cast experiments likewise can only provide information on deposition in the upper airways, although again serve to demonstrate the ability of fibrous particles to penetrate to the lower respiratory tract (Zhou et al. Citation2007; Su and Cheng Citation2015). Results from a number of acute inhalation exposure studies in rats which assessed deposition efficiency of fibers, mainly asbestos of length 1–6 µm, are summarized by Ding et al. (Citation1997). Over a range of fiber sizes, deposition efficiency in the whole lung was typically 16–19%, and 3–10% in the pulmonary region. However, the majority of these studies used particles with aerodynamic equivalent diameters (which we calculate from the dimensions given to be 500 nm to 40 µm) significantly larger than sizes expected for ‘fiber-like’ CNT aerosols, hence the observed deposition characteristics may not be directly comparable to CNT. The smallest particles used in any of the studies reported in Ding et al. (Citation1997) were glass fibers used by Griffis et al. (Citation1981) with median diameter 110 nm and median length 8.3 µm (aerodynamic diameter around 400–450 nm based on assumed density in the range 2.2–2.7 g cm−3), which displayed deposition efficiency of around 3–4% in the alveolar region. A more recent review of deposition data from typically 5 day in vivo rat inhalation studies using types of insulation fibers, identified deposition fractions in the range 1–16%, however, again these fibers were significantly larger than ‘fiber-like’ CNT (diameters in the range 340–2440 nm and lengths from 10 to >20 µm) and so the results are not directly applicable (Nielsen and Koponen, Citation2018).
Given the limited experimental data on fiber deposition, it is important to recognize that the validation currently possible for such models is significantly less extensive than that for models for spherical particles. An effort to validate a fiber deposition model was undertaken by Ding et al. (Citation1997), who evaluated their model performance against a number of in vivo studies in rats. Whole-lung and regional deposition was assessed, with slightly higher model prediction for total and nasal deposition, but better agreement in the lower respiratory tract and pulmonary region. Against the same experimental data, the model of Asgharian and Anjilvel (Citation1998) also performed reasonably well, predicting that for a given fiber diameter, deposition decreases with aspect ratio for diffusion-dominated regions and increases with aspect ratio in regions where impaction and interception are more important. Several studies report that increasing aspect ratio shifts the deposition efficiency curve to smaller diameters (Asgharian and Anjilvel Citation1998; Sturm and Hofmann Citation2009; Högberg et al. Citation2010). Nevertheless, existing experimental fiber deposition data mainly relate to larger and thicker fibers than typical ‘fiber-like’ CNT particles, which means that deposition models developed using and validated against data from, for example, the literature on in vivo asbestos studies may not be directly applicable to shorter, thinner CNT. This is especially likely in the alveolar region, where penetration of the larger asbestos fibers drops with increasing generations from the terminal bronchioles (Sébastien Citation1991) but smaller CNT may be expected to continue deeper into the alveolar region. Many types of CNT also tend to be less rigid and consequently more bent and/or tangled than asbestos fibers. There are therefore questions as to the suitability of models derived for straight, rigid fibers in predicting the deposition of some types of CNT particles, in particular with respect to interception (Podgorski and Gradon Citation2012).
Experimental CNT deposition data and model validation
As previously stated, to our knowledge none of the deposition models currently being applied in CNT aerosol studies have been validated against experimental deposition data for CNT aerosols. This is unsurprising since very few CNT in vivo inhalation studies have provided data that could be used for such a validation exercise. This is due in part to the significant challenges involved in the quantitative determination of CNT burdens in tissues (Jacobsen et al. Citation2017) and the concomitant lack of internationally agreed standard protocols. Techniques reported for CNT tissue burden quantification include: microscopy-based approaches (Stapleton et al. 2012), measurement of associated impurities (Pauluhn Citation2010), use of markers (Kasai et al. Citation2016), x-ray diffraction and elemental carbon analysis (Oyabu et al. Citation2011). The majority of inhalation studies in which CNT lung burdens have been determined are long-term and thus the measured lung burdens reflect the effects of both the initial deposition and subsequent clearance. Deriving deposition efficiencies from such data would therefore require corrections for clearance. Some studies have used lung burden data to determine a ‘deposition fraction’ (using an approach similar to EquationEquation (1)(1)
(1) ), which is the ratio of the measured lung burden to the total quantity of material inhaled. For example, Oyabu et al. (Citation2011) measured the lung burden at 3 days following a 4 week exposure and estimated that this was 18–20% of the material inhaled and Kasai et al. (Citation2016) estimated a deposition fraction of 1.5–2.7% following a 2 year exposure. In neither study was clearance addressed in obtaining these estimates, so these deposition fractions cannot be directly interpreted as deposition efficiencies. The authors are aware of only one study which has used a single short CNT exposure (6 h) and experimentally determined a lung burden (Kim et al. Citation2020). The authors did not use the data to derive a deposition efficiency but using the aerosol mass concentration provided and assuming (a) sacrifice at 24 h post-exposure and (b) the MPPD default breathing rate, we estimate it represents a deposition efficiency in the alveolar region of approximately 2%. Ideally, further specific targeted studies with short exposures should be undertaken to allow a more direct assessment of deposition efficiency to produce data for deposition model validation. This will likely require the further development of agreed techniques and procedures for the measurement of CNT tissue burdens. It is also important to note that to determine deposition efficiencies in the total lung additional measurements beyond simply lung burdens are required, including excreta and potentially other organs and tissues, to account for initially deposited material that is rapidly transported via the mucociliary escalator or transported to other organs and tissues (Buckley et al. Citation2016).
Error propagation in deposition models from varying aerosol parameters and the implications of aerosol heterogeneity for model use
The models described above require input data related to both the animal model and the aerosol. For the aerosol, this typically includes median, mean or mass median particle diameter (e.g. aerodynamic, mobility or physical), a measure of the particle size distribution, typically the geometric standard deviation (GSD), and particle density. Therefore, inaccuracies in the measurement of, or assumptions regarding, these parameters may propagate through the model, leading to errors in retrieved deposition efficiency and hence received dose. For example, a basic sensitivity analysis (described in Supplementary Information) using the MPPD model (v3.04) reveals that failing to account for the lower density of typical broadly-spherical CNT may lead to overestimation of the deposition efficiency for the smallest particles (mass median aerodynamic diameter, MMAD, <100 nm) by a factor of 2–3 in both humans and rats, although this effect is less apparent for large particles. Effects relating to broader size distributions are apparent but less extreme; particle deposition efficiency is typically 5–20% higher for a particle size distribution with GSD of 2.4 compared with GSD of 1.7. These results highlight the importance of adequately assessing the delivered CNT particle aerosol characteristics for use in deposition modeling. Note that this broad analysis is expected to apply to most deposition models; MPPD was used here as an example due to its wide use in nanotoxicology studies and because of its accessibility, utility and pedigree (Asgharian and Anjilvel Citation1998; Miller et al. Citation2016).
For significantly heterogeneous CNT aerosols it may be necessary to consider different components of the aerosol (e.g. where shape or density is expected to vary with particle diameter) separately within deposition models. For example, where the size distribution is clearly not log-normal it may be appropriate to consider two or more components. Some software allows such approaches directly by inputting a number of modes or binned data allowing a full distribution to be input. However, typically only a single density value will be input. For some very heterogeneous aerosols both broadly spherical and fiber-like particles may be present covering a wide density range, which can clearly be an issue for CNT aerosols as particle density can vary with form and size as described earlier. This in turn affects the inferred relationship between aerodynamic and diffusion diameter, as density appears in the expression linking the two. Hence, if CNT particle density is not known or is inaccurate, and/or if density varies with particle size, then depending on the diameter input parameter chosen (i.e. aerodynamic or diffusion diameter), typical model output may be reasonable for only part of the aerosol size distribution and may be inaccurate for other parts. For example, in the case of aerodynamic diameter measurement providing the model input parameter, deposition efficiency via impaction and sedimentation at larger aerosol sizes may be estimated accurately in models but these may not adequately reflect the diffusional deposition for smaller aerosol sizes; and vice versa for diffusion diameter measurements e.g. via SMPS. This may be a significant issue for many practically encountered CNT aerosols which extend over the size range where both diffusional and aerodynamic deposition mechanisms are operating.
Uncertainties on deposition model predictions
All model predictions have some level of associated uncertainty arising both from uncertainties on model input parameters and the extent to which the model reflects reality. As indicated above, the latter can be investigated by comparison with experimental data, although it is important to note that different models developed from similar data can predict very different results in some circumstances (e.g. differences in predictions of NRCP and ICRP lung deposition models, Yeh et al. Citation1996). An understanding of the level of uncertainty on a deposition estimate is important to an appropriate interpretation of the result. Limited uncertainty and sensitivity analyses have been undertaken for some deposition models and these have indicated potentially significant uncertainties on estimates (Harvey and Hamby Citation2001; de Winter-Sorkina and Cassee Citation2002). To the authors knowledge, no systematic uncertainty analyses have been undertaken with a focus on the modeling of fiber deposition. It is therefore recommended that such studies be undertaken to improve the understanding and interpretation of model outputs.
Measurement of CNT aerosol parameters
As indicated above, deposition models typically require the following input data defining the aerosol: median, mean or mass median particle diameter (e.g. aerodynamic, mobility or physical), a measure of the particle size distribution, typically the GSD, and particle density. General guidance on approaches for the measurement of aerosol particle size distributions is available from a number of sources (Hinds Citation1999; Kulkarni et al. Citation2011), with some CNT specific guidance also available (Chen et al. Citation2012). The following section highlights some of the particular issues associated with the measurement of CNT aerosol size distribution characteristics using commonly used instruments, and the uncertainties that may result. Issues with techniques and instruments for measuring aerosol particle density are also addressed and the measurement challenges posed by the heterogenous nature of typical CNT aerosols discussed. provides a summary of the recommended aerosol characterization requirements for CNT toxicity studies. This reflects standard toxicity study requirements, the needs of deposition modeling and the growing consensus for nanotoxicology studies that determining and reporting a range of aerosol exposure (and dose) metrics is important to allow appropriate interpretation and comparison of study results, both now and in the future (Schmid and Stoeger Citation2016; Schmid and Cassee Citation2017). Recommendations for measurements of ‘bulk’ characteristics of the material which may be important for toxicity (e.g. functionalization and/or chemical composition, zeta potential) but not specifically related to measurement of the aerosolized particles are not included in this table.
Table 4. Recommended carbon nanotube aerosol characterization and suggested measurement methods for toxicity studies.
CNT aerosol size distribution measurement
The particular characteristics of CNT aerosols pose issues in their measurement via several common techniques (Chen et al. Citation2016a). Of particular interest is the response of Differential Mobility Analyzers (DMAs) (Flagan Citation2008) within Scanning Mobility Particle Sizer (SMPS) systems, and Aerodynamic Particle Sizers (APS), to CNT particles, given their widespread use in CNT exposure studies and in particular, their use to obtain particle size distributions and effective densities.
Assumptions regarding electrostatic charging of the particle population is central to the SMPS technique. The ‘charge distribution’ on non-spherical particles such as fiber-like CNT has not been well-established, but could be higher than spherical particles of similar size. For example, charge levels for low-density CNT particles may be higher than that expected for more compact particles (Baron et al. Citation2008; Nasibulin et al. Citation2008; Kulkarni et al. Citation2009). In practice this could lead to increased polydispersity of classified particles and a decoupling of the relationship between mobility and aerodynamic diameter (Chen et al. Citation2016a), meaning standard methods for conversion between the two may not be applicable. The (generally necessary) use of a single-stage impactor prior to the DMA inlet can also lead to underestimation of both the true mobility diameter and concentration, if the mean (aerodynamic) particle diameter is below the impactor cutoff diameter, as for low density particles (Chen et al. Citation2016a). Furthermore, if the nature of the particles changes over the size distribution measured e.g. if particles become more fiber-like as they get smaller (Chen et al. Citation2012) and/or effective density depends on size (Ku and Kulkarni Citation2015; Wang et al. Citation2015; Gaté et al. Citation2019), mobility size distributions and number concentrations could be affected. Non-spherical particles may also align in the electric field in Differential Mobility Analyzers (DMAs) leading to inaccuracies in classified particle characteristics, although this behavior could be exploited to obtain further shape information (Zelenyuk and Imre Citation2007). The performance of the APS in measuring CNT particles is also somewhat uncertain, especially toward the lower sizes detectable by the instrument (this is discussed subsequently in relation to obtaining particle densities). Further work in determining instrument performance for a range of CNT, and on the electrical charge distribution on fiber-like and broadly-spherical CNT particles, is required to improve confidence in results from these techniques and thus reduce potential errors in estimated deposition efficiencies and doses in toxicological studies.
Airborne CNT particle density measurement
A number of different methods have been employed to assess the ‘effective density’ (see Supplementary Information for a clarification of this term) of a range of aerosols including CNT. However, many share a common feature which is the concurrent, or in-series, characterization of airborne particles by both mobility and mass. Often, as described earlier, mobility size distributions are assessed via SMPS or similar instrument. In the case of ‘tandem’ techniques, the DMA component can be used to select particles according to their mobility. This subset of the overall population is then passed to an instrument which reclassifies them by mass e.g. via Aerosol Particle Mass analyzer (APM) (McMurry et al. Citation2002; Kim et al. Citation2009; Ku and Kulkarni Citation2015) or Centrifugal Particle Mass Analyzer (CPMA) (Olfert et al. Citation2007), or by aerodynamic diameter e.g. via Aerodynamic Aerosol Classifier (AAC) (Tavakoli and Olfert Citation2014), allowing information on effective density and shape factor to be obtained. Another method is to measure the overall mass of the selected particle population, rather than individual particles, for example via Tapered Element Oscillating Microbalance (TEOM) e.g. Morawska et al. (Citation1999), or Quartz Crystal Microbalance (QCM) e.g. Sarangi et al. (Citation2016).
Several studies involving CNT (e.g. Mitchell et al. Citation2007; Baron et al. Citation2008) have undertaken simultaneous aerosol measurement using SMPS and APS, primarily because the combination covers a broad particle size range (often from 10 nm to 20 µm). Typically, the size ranges measured by the two instruments overlap, therefore merging of the two distributions can be achieved using a ‘correction factor,’ related to the particle shape and effective density, to convert between the distributions measured by the instruments (aerodynamic, and mobility diameter). However, a number of issues affect the accuracy of these estimates. The region of overlap of most SMPS and APS systems is relatively small (usually at most 0.5–0.7 µm e.g. Khlystov et al. Citation2004; Beddows et al. Citation2010) and information on effective density of particles far outside this region, which may be different for both larger and smaller CNT particles (Chen et al. Citation2012; Ku and Kulkarni Citation2015; Wang et al. Citation2015), is not retrievable through this method alone. Khlystov et al. (Citation2004) also discuss errors due to counting efficiency primarily in the APS, which could arise from unit-to-unit variability and the optical properties, largely the complex refractive index, of the particles (Pfeifer et al. Citation2016), which to our knowledge has not been reported for airborne CNT particles. This could affect both aerodynamic size distributions reported by the instrument, and correction factors (and hence effective density) derived using these data if, as has been previously observed, these errors are size-dependent and occur largely in the overlap range as APS counting efficiency drops (Beddows et al. Citation2010). A number of improvements to the fitting algorithm have been employed in an atmospheric aerosol context (Beddows et al. Citation2010) but to our knowledge have not been used in CNT studies. Finally, but importantly, the shape factor must be separately deduced to unfold the effects of effective density and shape from the overall correction factor, requiring further measurement e.g. via TEM of the particles specifically from the overlap size range. Use of quantitative SMPS and APS data for dose estimation and related toxicological impact should thus be undertaken with caution (Chen et al. Citation2016a) and ideally supplemented with direct measurement of mass distribution e.g. via Electrical Low Pressure Impactor (ELPI) or MOUDI/nanoMOUDI (Micro Orifice Uniform Deposit Impactor).
We note that, due to the different quantities measured by the various instruments employed, there are several different definitions of effective density, which can be numerically quite varied for the same aerosol (DeCarlo et al. Citation2004; Supplementary Information). Hence, care must be taken when comparing ‘effective densities’ reported in previous studies, and the exact definition used in future studies should be reported, as is the case for some (e.g. Ku and Kulkarni Citation2015), but not all, previous reports.
Oberdörster and Kuhlbusch (Citation2018) outline a method by which the ‘in vivo effective density’ could be estimated by measuring lung burden and using study parameters to calculate deposition efficiency as per EquationEquation (1)(1)
(1) , then performing runs with a deposition model which allows for variability in particle density as an adjustable input (e.g. MPPD) to find the density at which the best fit to Deff is achieved. However, experimental uncertainty in several parameters used to determine in vivo deposition efficiency (EquationEquation (1)
(1)
(1) ) is commonly quite large. Model outputs are also likely to be highly sensitive to small changes in input e.g. for MPPD v.3.04, perturbing the deposition efficiency of unit density, 1 µm MMAD, 1.7 GSD, particles in the Rat (Sprague-Dawley Asymmetric model with adjustment for inhalability, all other values set to default) by ± 10% then performing the iterative procedure outlined above results in a possible effective density range from 0.43 to 1.85 g cm−3, much larger than the ±10% artificial error introduced initially. Experimentally assessing effective density, for example with concurrent mobility and aerodynamic diameter and gravimetric mass measurements as is commonly reported, is likely to provide a more accurate outcome, despite the unresolved issues regarding these measurements outlined above.
CNT particle morphology and density
For certain types of particle, further observations or assumptions regarding structure can be used to constrain the relationship between mass and mobility. For example, for fiber-like MWCNT, TEM measurement of the crystal structure of the CNT can provide information on the ‘dead space’ between concentric tubes, linking the intrinsic density of the material to the effective density of the individual fibers (Laurent et al. Citation2010; Wang et al. Citation2015). In the case of agglomerate aerosols, use of a ‘mass scaling exponent’ (analogous to fractal dimension) can provide a direct mathematical link between mass and mobility diameter (Olfert et al. Citation2007; Ku and Kulkarni Citation2015). This approach has been extended to agglomerates of SWCNT and carbon nanofibers (CNF) (Ku et al. Citation2006) and MWCNT (Wang et al. Citation2015). An exploration of the appropriateness of this approach, for CNT with open structures, based on morphological characterization via TEM imaging, is provided by Ku and Kulkarni (Citation2018), who found agreement to within 30–40% and 40–50% between direct measurement of diameter from TEM images, and aerodynamic and mobility diameters respectively. However, effective densities obtained using this TEM morphological method were underestimated by up to 80% compared to those from tandem-mass-mobility measurements.
Whilst particle density is now generally being assessed in in vivo studies, albeit with potential instrumentation and fundamental issues affecting accuracy of these assessments, we also advocate in future studies reporting as much additional information on particle morphology as possible, to improve density estimates but also in anticipation of further model developments and/or to aid model validation, in particular with respect to interception. For example, from TEM image processing, a range of geometric parameters including Feret, Martin or ‘envelope’ diameters (Ku and Kulkarni Citation2015; Trubetskaya et al. Citation2017) and projected area can be obtained, details of which, in conjunction with computational modeling and experimental deposition measurements, may provide further insight into CNT particle behavior in the lung.
Characterization of subsets of the CNT aerosol distribution
If some toxicity related endpoints are specific to particular components of the aerosol (for example, only the longest fibers may be potentially implicated in asbestos-like pathology of the mesothelium; Poland et al. Citation2008), then there may be a future requirement to provide deposition doses for those in particular, to allow comparison with other aerosols. In view of the heterogeneous nature of the CNT aerosol and the variation of several important parameters (such as density and shape) with particle size, this requires a subset of the overall particle size distribution to be selected and studied independently of the overall aerosol population. A number of studies have sampled subsets of the CNT aerosol for further characterization, most commonly achieved using a cascade impactor (Shvedova et al. Citation2008; Porter et al. Citation2012; Chen et al. Citation2016a). Whilst cascade impactors are commonly used to obtain a mass size distribution, by additionally sampling onto suitable substrates, for example, morphological characterization via electron microscopy and subsequent image analysis of a sample within a known aerodynamic diameter range can be undertaken and potentially samples collected for analysis of additional factors such as mechanical stiffness (Kane et al. Citation2018). Combined with precise mass measurements for each impactor stage, some density information can also be obtained (although this method is not well suited for examining the smallest particles, which have very little mass). This approach may aid in assessing whether morphology variability with particle size, for example the prevalence of single fibers rather than broadly spherical agglomerates at smaller diameters, could affect deposition efficiency and/or location, and hence, received dose and lung burden. This would complement methodological innovations designed to generate aerosol with specific characteristics e.g. Taquahashi et al. (Citation2013) whose method largely removes the agglomerated CNT, but preserves the single fibers, of MWNT-7 CNT to be delivered in an inhalation exposure system.
Conclusions and research needs
There is considerable concern over the possible health implications of CNT deposition in the respiratory system, and as a result an increasing number of in vivo inhalation studies are being conducted to study their toxicity, with the aim of providing information to support human health risk assessments. Many such studies provide estimates of the mass of CNT deposited in the lung, rather than aerosol mass concentration alone, as a growing international consensus and associated guidance has identified the importance of this information in improving inter-study comparability and animal-human extrapolation. Although in some studies deposited dose is measured, in the majority of cases models are utilized to provide estimates. Herein we have identified potential limitations in current models in both how they are used and the results reported and interpreted, and have made recommendations for research and actions to improve the quality and robustness of lung deposition estimates (summarized in ). It is concluded that:
Figure 1. Objectives and recommendations for future work to improve characterization of CNT aerosols, deposition modeling and dose estimation.

Targeted experiments are required to investigate the importance of interception as a deposition process and also the extent to which electrostatic forces influence CNT particle deposition; as a preliminary step this could involve measuring the electrostatic charge of typical broadly-spherical and fiber-like particles to scope the potential for significant effects.
Short-term in vivo studies are required to provide experimental data on deposition which can be used for deposition model validation. This is of particular importance for ‘fiber-like’ particles for which limited data is available.
Model developers should ensure that models are flexible enough to allow the representation of the heterogenous nature of many CNT aerosols.
Systematic uncertainty studies should be undertaken to identify key parameters and provide an understanding of the typical levels of uncertainties on model predictions, as above, this is of particular importance for fibrous particles.
Research on aerosol instrument performance for CNTs should be undertaken to enable appropriate measurement of model input parameters and the development of consensus on the derivation of parameters including particle density for all or component parts of the aerosol.
Good practice guidelines on study performance should highlight the need to report and justify the model used and all the input data values assumed, and the reporting of the results to reflect the robustness of any deposition estimate (i.e. an appreciation of the uncertainty on any estimate).
All the above will improve the robustness of deposition estimates for any particular study. It is also noted that as understanding of the toxicity of inhaled CNT grows, key components of the aerosol may be identified as of greater significance for particular biological endpoints than others, for example, the longer and/or more rigid fibers may be of greater concern, and there may therefore be a need to link specific groups of aerosol particles with certain endpoints. To future-proof studies against such concerns, i.e. to allow future reevaluation of the results, it is recommended that aerosol characterization beyond minimum requirements is undertaken, to include, in particular, imaging to link particle morphology to mass and size (a summary of our recommendations on aerosol characterization for CNT toxicity studies is provided in ). Although somewhat beyond the scope of this review, it is also noted that particle lung deposition may be altered by changes in airway structure in people with preexisting conditions such as COPD or asthma, who may also potentially be more susceptible to health risks associated with CNT exposure, and as such more work could usefully be undertaken in this area to address wider risk assessment questions. This would be relevant to a wide range of particles types and also be of interest for drug delivery.
It is recognized that ultimately improvements in the areas identified above will require a co-ordinated interdisciplinary approach involving efforts from a range of groups including: deposition model developers and users, experimental aerosol scientists and technicians, exposure scientists and study designers.
Supplemental Material
Download PDF (1.1 MB)Disclosure statement
No potential conflict of interest is reported by the author(s).
References
- Anjilvel S, Asgharian B. 1995. A multiple-path model of particle deposition in the rat lung. Toxicol Sci. 28:41–50.
- [ARA] Applied Research Associates. 2018. [accessed 2020 Feb 5]. https://www.ara.com/products/multiple-path-particle-dosimetry-model-mppd-v-304
- Asgharian B, Anjilvel S. 1998. A multiple-path model of fiber deposition in the rat lung. Toxicol Sci. 44:80–86.
- Asgharian B, Yu CP. 1988. Deposition of inhaled fibrous particles in the human lung. J Aerosol Med. 1:37–50.
- Asgharian B, Yu CP. 1989. Deposition of fibers in the rat lung. J Aerosol Sci. 20:355–366.
- Bahk YK, Buha J, Wang J. 2013. Determination of geometrical length of airborne carbon nanotubes by electron microscopy, model calculation, and filtration method. Aerosol Sci Technol. 47:776–784.
- Balásházy I, Moustafa M, Hofmann W, Szöke R, El-Hussein A, Ahmed AR. 2005. Simulation of fiber deposition in bronchial airways. Inhal Toxicol. 17:717–727.
- Baron P, Deye GJ, Chen BT, Schwegler-Berry DE, Shvedova AA, Castranova V. 2008. Aerosolization of single-walled carbon nanotubes for an inhalation study. Inhal Toxicol. 20:751–760.
- Beddows DCS, Dall'osto M, Harrison RM. 2010. An enhanced procedure for the merging of atmospheric particle size distribution data measured using electrical mobility and time-of-flight analysers. Aerosol Sci Technol. 44:930–938.
- Bello D, Wardle BL, Zhang J, Yamamoto N, Santeufemio C, Hallock M, Virji MA. 2010. Characterization of exposures to nanoscale particles and fibers during solid core drilling of hybrid carbon nanotube advanced composites. Int J Occup Environ Health. 16:434–450.
- Brody AR, Roe MW. 1983. Deposition pattern of inorganic particles at the alveolar level in the lungs of rats and mice. Am Rev Respir Dis. 128:724–729.
- Buckley AJ, Hodgson A, Warren J, Guo C, Smith R. 2016. Size-dependent deposition of inhaled nanoparticles in the rat respiratory tract using a new nose-only exposure system. Aerosol Sci Technol. 50:1–10.
- Canu IG, Bateson TF, Bouvard V, Debia M, Dion C, Savolainen K, Yu IJ. 2016. Human exposure to carbon-based fibrous nanomaterials: a review. Int J Hyg Environ Health. 219:166–175.
- Chalupa DC, Morrow PE, Oberdörster G, Utell MJ, Frampton MK. 2004. Ultrafine particle deposition in subjects with asthma. Environ Health Perspect. 112:879–882.
- Chen BT, Schwegler-Berry D, Cumpston A, Cumpston J, Friend S, Stone S, Keane M. 2016a. Performance of a scanning mobility particle sizer in measuring diverse types of airborne nanoparticles: Multi-walled carbon nanotubes, welding fumes, and titanium dioxide spray. J Occup Environ Hyg. 13:501–508.
- Chen BT, Schwegler-Berry D, McKinney W, Stone S, Cumpston JL, Friend S, Porter DW, Castranova V, Frazer DG. 2012. Multi-walled carbon nanotubes: sampling criteria and aerosol characterization. Inhal Toxicol. 24:798–820.
- Chen X, Zhong W, Tom J, Kleinstreuer C, Feng Y, He X. 2016b. Experimental-computational study of fibrous particle transport and deposition in a bifurcating lung model. Particuology. 28:102–113.
- Chortarea S, Clift MJD, Vanhecke D, Endes C, Wick P, Petri-Fink A, Rothen-Rutishauser B. 2015. Repeated exposure to carbon nanotube-based aerosols does not affect the functional properties of a 3D human epithelial airway model. Nanotoxicology. 9:983–993.
- Crowde TM, Rosati JA, Schroeter JD, Hickey AJ, Martonen TB. 2002. Fundamental effects of particle morphology on lung delivery: predictions of Stokes’ law and the particular relevance to dry powder inhaler formulation and development. Pharm Res. 19:239–245.
- Dahm MM, Evans DE, Schubauer-Berigan MK, Birch ME, Fernback JE. 2012. Occupational exposure assessment in carbon nanotube and nanofiber primary and secondary manufacturers. Ann Occup Hyg. 56:542–556.
- Dahm MM, Schubauer-Berigan MK, Evans DE, Birch ME, Fernback JE, Deddens JA. 2015. Carbon nanotube and nanofiber exposure assessments: an analysis of 14 site visits. ANNHYG. 59:705–723.
- Dahm MM, Schubauer-Berigan MK, Evans DE, Birch ME, Bertke S, Beard JD, Erdely A, Fernback JE, Mercer RR, Grinshpun SA. 2018. Exposure assessments for a cross-sectional epidemiologic study of US carbon nanotube and nanofiber workers. Int J Hyg Environ Health. 221:429–440.
- DeCarlo PF, Slowik JG, Worsnop DR, Davidovits P, Jimenez JL. 2004. Particle morphology and density characterization by combined mobility and aerodynamic diameter measurements. Part 1: theory. Aerosol Sci Tech. 38:1185–1205.
- de Winter-Sorkina R, Cassee FR. 2002. From concentration to dose: factors influencing airborne particulate deposition in humans and rats. Bilthoven (Netherlands): RIVM. Report 650010031/2002.
- Ding JY, Yu CP, Zhang L, Chen YK. 1997. Deposition modelling of fibrous particles in rats: comparisons with available experimental data. Aerosol Sci Technol. 26:403–414.
- Donaldson K, Poland CA, Murphy FA, MacFarlane M, Chernova T, Schinwald A. 2013. Pulmonary toxicity of carbon nanotubes and asbestos – similarities and differences. Adv Drug Deliv Rev. 65:2078–2086.
- Dong J, Ma Q. 2019. Integration of inflammation, fibrosis, and cancer induced by carbon nanotubes. Nanotoxicology. 13:1244–1274.
- [ECETOC] European Centre for Ecotoxicology and Toxicology of Chemicals. 2013. Poorly soluble particles/lung overload. Brussels: ECETOC. Technical Report No. 122.
- Ellenbecker M, Tsai SJ, Jacobs M, Riediker M, Peters T, Liou S, Avila A, FossHansen S. 2018. The difficulties in establishing an occupational exposure limit for carbon nanotubes. J Nanopart Res. 20:131.
- Flagan RC. 2008. Differential mobility analysis of aerosols: a tutorial. KONA. 26:254–268.
- Fröhlich E. 2012. The role of surface charge in cellular uptake and cytotoxicity of medical nanoparticles. Int J Nanomed. 7:5577–5591.
- Gaté L, Knudsen KB, Seidel C, Berthing T, Chézeau L, Jacobsen NR, Valentino S, Wallin H, Bau S, Wolff H, et al. 2019. Pulmonary toxicity of two different multi-walled carbon nanotubes in rat: Comparison between intratracheal instillation and inhalation exposure. Toxicol Appl Pharmacol. 375:17–31.
- Ging J, Tejerina-Anton R, Ramakrishnan G, Nielsen M, Murphy K, Gorham JM, Nguyen T, Orlov A. 2014. Development of a conceptual framework for evaluation of nanomaterials release from nanocomposites: Environmental and toxicological implications. Sci Total Environ. 473-474:9–19.
- Griffis LC, Henderson TR, Pickrell JA. 1981. A method for determining glass in rat lung after exposure to a glass fiber aerosol. Am Ind Hyg Assoc J. 42:566–569.
- Han JH, Lee EJ, Lee JH, So KP, Lee YH, Bae GN, Lee SB, Ji JH, Cho MH, Yu IJ. 2008. Monitoring multiwalled carbon nanotube exposure in carbon nanotube research facility. Inhal Toxicol. 20:741–749.
- Hao Y, Qunfeng Z, Fei W, Weizhong Q, Guohua L. 2003. Agglomerated CNTs synthesized in a fluidized bed reactor: agglomerate structure and formation mechanism. Carbon. 41:2855–2863.
- Harris RL, Fraser DA. 1976. A model for deposition of fibers in the human respiratory system. Am Ind Hyg Assoc J. 37:73–89.
- Harvey RP, Hamby DM. 2001. Uncertainty in particulate deposition for 1 microm AMAD particles in an adult lung model. Radiat Prot Dosimetry. 95:239–247.
- Hedmer M, Isaxon C, Nilsson PT, Ludvigsson L, Messing ME, Genberg J, Skaug V, Bohgard M, Tinnerberg H, Pagels JH. 2014. Exposure and emission measurements during production, purification, and functionalization of arc-discharge-produced multi-walled carbon nanotubes. Ann Occup Hyg. 58:355–379.
- [HEI] Health Effects Institute. 1991. Asbestos in public and commercial buildings: a literature review and synthesis of current knowledge. Cambridge (MA): Health Effects Institute-Asbestos Research.
- Hinds WC. 1999. Aerosol Technology. 2nd ed. New York: Wiley.
- Hofmann W. 2009. Modelling particle deposition in human lungs: modelling concepts and comparison with experimental data. Biomarkers. 14:59–62.
- Hofmann W. 2011. Modelling inhaled particle deposition in the human lung – a review. J Aerosol Sci. 42:693–724.
- Högberg SM, Âkerstedt HO, Lundström TS, Freund JB. 2010. Respiratory deposition of fibers in the non-inertial regime – development and application of a semi-analytical model. Aerosol Sci Technol. 44:847–860.
- Hussein T, Löndahl J, Paasonen P, Koivisto AJ, Petäjä T, Hämeri K, Kulmala M. 2013. Modeling regional deposited dose of submicron aerosol particles. Sci Total Environ. 458-460:140–149.
- [IARC] International Agency for Research on Cancer. 2017. IARC monographs on the evaluation of carcinogenic risks to humans. Volume 111. Some nanomaterials and some fibres. Lyon: IARC.
- [ICRP] International Commission on Radiological Protection 1994. Human respiratory tract model for radiological protection. ICRP Publication 66. Oxford: Pergamon Press.
- Ihrie MD, Taylor-Just AJ, Walker NJ, Stout MD, Gupta A, Richey JS, Hayden BK, Baker GL, Sparrow BR, Duke KS, Bonner JC. 2019. Inhalation exposure to multi-walled carbon nanotubes alters the pulmonary allergic response of mice to house dust mite allergen. Inhal Toxicol. 31:192–202.
- Jackson P, Kling K, Jensen KA, Clausen PA, Madsen AM, Wallin H, Vogel U. 2015. Characterization of genotoxic response to 15 multiwalled carbon nanotubes with variable physicochemical properties including surface functionalizations in the fe1-muta(tm) mouse lung epithelial cell line. Environ Mol Mutagen. 56:183–203.
- Jacobsen NR, Møller P, Clausen PA, Saber TA, Micheletti C, Jensen KA, Wallin H, Vogel U. 2017. Biodistribution of carbon nanotubes in animal models. Basic Clin Pharmacol Toxicol. 121:30–43.
- Jakobsson JKF, Aaltonen HL, Nicklasson H, Gudmundsson A, Rissler J, Wollmer P, Löndahl J. 2018. Altered deposition of inhaled nanoparticles in subjects with chronic obstructive pulmonary disease. BMC Pulmon Med. 18:129.
- Jarabek AN, Asgharian B, Miller FJ. 2005. Dosimetric adjustments for interspecies extrapolation of inhaled poorly soluble particles (PSP). Inhal Toxicol. 17:317–334.
- Johnson DR, Methner MM, Kennedy AJ, Steevens JA. 2010. Potential for occupational exposure to engineered carbon-based nanomaterials in environmental laboratory studies. Environ Health Perspect. 118:49–54.
- [JRC] Joint Research Centre, European Commission 2014. Multi-walled carbon nanotubes, NM-400, NM-401, NM-402, NM-403: characterisation and physico-chemical properties. Luxembourg: JRC Repository: NM-Series of Representative Manufactured Nanomaterials, Publications Office of the European Union. Report JRC91205.
- Kadoya C, Lee BW, Ogami A, Oyabu T, Nishi K, Yamamoto M, Todoroki M, Morimoto Y, Tanaka I, Myojo T. 2016. Analysis of pulmonary surfactant in rat lungs after inhalation of nanomaterials: Fullerenes, nickel oxide and multi-walled carbon nanotubes. Nanotoxicology. 10:194–203.
- Kane AB, Hurt RH, Gao H. 2018. The asbestos-carbon nanotube analogy: An update. Toxicol Appl Pharmacol. 15:361–380.
- Kasai T, Gotoh K, Nishizawa T, Sasaki T, Katagiri T, Umeda Y, Toya T, Fukushima S. 2014. Development of a new multi-walled carbon nanotube (MWCNT) aerosol generation and exposure system and confirmation of suitability for conducting a single-exposure inhalation study of MWCNT in rats. Nanotoxicology. 8:169–178.
- Kasai T, Umeda Y, Ohnishi M, Mine T, Kondo H, Takeuchi T, Matsumoto M, Fukushima S. 2016. Lung carcinogenicity of inhaled multi-walled carbon nanotube in rats. Part Fibre Toxicol. 13:53.
- Khlystov A, Stanier C, Pandis SN. 2004. An algorithm for combining electrical mobility and aerodynamic size distributions data when measuring ambient aerosol. Aerosol Sci Technol. 38:229–238.
- Kim JK, Jo MI, Kim Y, Kim TG, Shin JH, Kim BW, Kim HP, Lee HK, Kim HS, Ahn K, Oh SM, et al. 2020. 28-day inhalation toxicity study with evaluation of lung deposition and retention of tangled multi-walled carbon nanotubes. Nanotoxicology. 14:250–262.
- Kim SH, Mulholland GW, Zachariah MR. 2009. Density measurement of size selected multiwalled carbon nanotubes by mobility-mass characterization. Carbon. 47:1297–1302.
- Kim SH, Woo KS, Liu MR, Zachariah MR. 2005. Method of measuring charge distribution of nanosized aerosols. J Colloid Interface Sci. 282:46–57.
- Kim WG, Yong SD, Yook SJ, Ji JH, Kim KH, Bae GN, Chung EK, Kim J. 2017. Comparison of black carbon concentration and particle mass concentration with elemental carbon concentration for multi-walled carbon nanotube emission assessment purpose. Carbon. 122:228–236.
- Kingston C, Zepp R, Andrady A, Boverhof D, Fehir R, Hawkins D, Roberts J, Sayre P, Shelton B, Sultan Y, et al. 2014. Release characteristics of selected carbon nanotube polymer composites. Carbon. 68:33–57.
- Kleinstreuer C, Feng Y. 2013. Computational analysis of non-spherical particle transport and deposition in shear flow with application to lung aerosol dynamics – a review. J Biomech Eng. 135:021008.
- Kleinstreuer C, Zhang Z, Li Z. 2008. Modeling airflow and particle transport/deposition in pulmonary airways. Respir Physiol Neurobiol. 163:128–138.
- Koblinger L, Hofmann W. 1990. Monte Carlo modelling of aerosol deposition in human lungs. Part I. Simulation of particle transport in a stochastic lung structure. J Aerosol Sci. 21:661–674.
- Kouassi S, Catto C, Ostiguy C, L’espérance G, Kroeger J, Debia M. 2017. Exposure assessment in a single-walled carbon nanotube primary manufacturer. Ann Work Expos Heal. 61(2):260–266. doi:10.1093/annweh/wxw017.
- Kovochich M, Fung CCD, Avanasi R, Madl AK. 2018. Review of techniques and studies characterizing the release of carbon nanotubes from nanocomposites: implications for exposure and human health risk assessment. J Expo Sci Environ Epidemiol. 28:203–215.
- Ku BK, Birch ME. 2019. Aerosolization and characterization of carbon nanotube and nanofiber materials: Relationship between aerosol properties and bulk density. J Aerosol Sci. 127:38–48. doi:10.1016/j.jaerosci.2018.10.004.
- Ku BK, Deye G, Turkevich LA. 2013. Characterization of a vortex shaking method for aerosolizing fibers. Aerosol Sci Technol. 47:1293–1301.
- Ku BK, Emery MS, Maynard AD, Stolzenburg MR, McMurry PH. 2006. In situ structure characterization of airborne carbon nanofibres by a tandem mobility-mass analysis. Nanotechnology. 17:3613–3621.
- Ku BK, Kulkarni P. 2015. Measurement of transport properties of aerosolized nanomaterials. J Aerosol Sci. 90:169–181.
- Ku BK, Kulkarni P. 2018. Application of fractal theory to estimation of equivalent diameters of airbrone carbon nanotube and nanofiber agglomerates. Aerosol Sci Technol. 52:597–608.
- Kuempel ED, Jaurand MC, Møller P, Morimoto Y, Kobayashi N, Pinkerton KE, Sargent LM, Vermeulen RCH, Fubini B, Kane AB. 2017. Evaluating the mechanistic evidence and key data gaps in assessing the potential carcinogenicity of carbon nanotubes and nanofibers in humans. Crit Rev Toxicol. 47:1–58.
- Kuijpers E, Bekker C, Fransman W, Brouwer D, Tromp P, Vlaanderen J, Godderis L, Hoet P, Lan Q, Silverman D, et al. 2016. Occupational exposure to multi-walled carbon nanotubes during commercial production synthesis and handling. ANNHYG. 60:305–317.
- Kulkarni P, Baron PA, Willeke K, editors. 2011. Aerosol measurement: principles, techniques, and applications. 3rd ed. New York: Wiley.
- Kulkarni P, Deye GJ, Baron PA. 2009. Bipolar diffusion charging characteristics of single-wall carbon nanotube aerosol particles. J Aerosol Sci. 40:164–179.
- Laurent C, Flahaut E, Peigney A. 2010. The weight and density of carbon nanotubes versus the number of walls and diameter. Carbon. 48:2994–2996.
- LeBuf RF, Stefaniak AB, Chen BT, Frazer DG, Virji MA. 2011. Measurement of airborne nanoparticle surface area using a filter-based gas adsorption method for inhalation toxicology experiments. Nanotoxicology. 5:687–699.
- Lee JH, Lee SB, Bae GN, Jeon KS, Yoon JU, Ji JH, Sung JH, Lee BG, Lee JH, Yang JS, et al. 2010. Exposure assessment of carbon nanotube manufacturing workplaces. Inhal Toxicol. 22:369–381.
- Lee YS, Sung JH, Song KS, Kim JK, Choi BS, Yu IJ, Park JD. 2019. Derivation of occupational exposure limits for multi-walled carbon nanotubes and graphene using subchronic inhalation toxicity data and a multi-path particle dosimetry model. Toxicol Res (Camb). 8:580–586.
- Ma-Hock L, Treumann S, Strauss V, Brill S, Luizi F, Mertler M, Wiench K, Gamer AO, van Ravenzwaay B, Landsiedel R. 2009. Inhalation toxicity of multiwall carbon nanotubes in rats exposed for 3 months. Toxicol Sci. 112:468–481.
- Maynard AD, Baron PA, Foley M, Shvedova AA, Kisin ER, Castranova V. 2004. Exposure to carbon nanotube material: aerosol release during the handling of unrefined single-walled carbon nanotube material. J Toxicol Environ Health Part A. 67:87–107.
- McKinney W, Chen B, Frazer D. 2009. Computer controlled multi-walled carbon nanotube inhalation exposure system. Inhal Toxicol. 21:1053–1061.
- McMurry PH, Wang X, Park K, Ehara K. 2002. The relationship between mass and mobility for atmospheric particles: a new technique for measuring particle density. Aerosol Sci Technol. 36:227–238.
- Menache MG, Miller FJ, Raabe OG. 1995. Particle inhalability curves for humans and small laboratory animals. Ann Occup Hyg. 39:317–328.
- Mercer RR, Scabilloni JF, Hubbs AF, Battelli LA, McKinney W, Friend S, Wolfarth MG, Andrew M, Castranova V, Porter DW. 2013. Distribution and fibrotic response following inhalation exposure to multi-walled carbon nanotubes. Part Fibre Toxicol. 10:33.
- Millage KK, Bergman J, Asgharian B, McClellan G. 2010. A review of inhalability fraction models: discussion and recommendations. Inhal Toxicol. 22:151–159.
- Miller FJ, Asgharian B, Schroeter JD, Price O. 2016. Improvements and additions to the multiple path particle dosimetry model. J Aerosol Sci. 99:14–26.
- Mitchell LA, Gao J, Vander Wal R, Gigliotti A, Burchiel SW, McDonald JD. 2007. Pulmonary and systemic immune response to inhaled multiwalled carbon nanotubes. Toxicol Sci. 100:203–214.
- Morawska L, Johnson G, Ristovski ZD, Agranovski V. 1999. Relation between particle mass and number for submicrometer airborne particles. Atmos Environ. 33(13):1983–1990. doi:10.1016/S1352-2310(98)00433-6.
- Nasibulin AG, Shandakov SD, Anisimov AS, Gonzalez D, Jiang H, Pudas M, Queipo P, Kauppinen EI. 2008. Charging of aerosol products during ferrocene vapor decomposition in N2 and CO atmospheres. J Phys Chem C. 112:5762–5769.
- Nielsen GD, Koponen IK. 2018. Insulation fiber deposition in the airways of men and rats. A review of experimental and computational studies. Regul Toxicol Pharm. 94:252–270. doi:10.1016/j.yrtph.2018.01.021.
- [NCRP] National Council on Radiation Protection and Measurements 1997. Deposition, retention and dosimetry of inhaled radioactive substances. Bethesda (MD): NCRP. Report No. 125.
- [NIOSH] National Institute for Occupational Safety and Health. 2013. Current intelligence bulletin 65: occupational exposure to carbon nanotubes and nanofibers. Cincinnati (OH): Centers for Disease Control and Prevention, NIOSH. DHHS (NIOSH) Publication Number 2013–145.
- Nowack B, David RM, Fissan H, Morris H, Shatkin JA, Stintz M, Zepp R, Brouwer D. 2013. Potential release scenarios for carbon nanotubes used in composites. Environ Int. 59:1–11.
- O’Shaughnessy P, Adamcakova-Dodd A, Altmaier R, Thorne P. 2014. Assessment of the aerosol generation and toxicity of carbon nanotubes. Nanomaterials. 4:439–453.
- Oberdörster G, Castranova V, Asgharian B, Sayre P. 2015. Inhalation exposure to carbon nanotubes (CNT) and carbon nanofibers (CNF): methodology and dosimetry. J Toxicol Environ Health B Crit Rev. 18:121–212.
- Oberdörster G, Kuhlbusch T. 2018. In vivo effects: methodologies and biokinetics of inhaled nanomaterials. Nanoimpact. 10:38–60.
- [OECD] Organisation for Economic Co-operation and Development. 2017a. Test No. 412: Subacute inhalation toxicity: 28-day study, OECD Guidelines for the Testing of Chemicals, Section 4. Paris: OECD Publishing. [accessed 2020 Feb 5]. http://dx.doi.org/10.1787/9789264070783-en
- [OECD] Organisation for Economic Co-operation and Development 2017b. Test No. 413: Subchronic inhalation toxicity: 90-day study, OECD Guidelines for the Testing of Chemicals, Section 4. Paris: OECD Publishing. [accessed 2020 Feb 5]. http://dx.doi.org/10.1787/9789264070806-en.
- Olfert JS, Symonds JPR, Collings N. 2007. The effective density and fractal dimension of particles emitted from a light-duty diesel vehicle with a diesel oxidation catalyst. J Aerosol Sci. 38:69–82.
- Oyabu T, Myojo T, Morimoto Y, Ogami A, Hirohashi M, Yamamoto M, Todoroki M, Mizuguchi Y, Hashiba M, Lee BW, et al. 2011. Biopersistence of inhaled MWCNT in rat lungs in a 4-week well-characterized exposure. Inhal Toxicol. 23:784–791.
- Pauluhn J. 2010. Subchronic 13-week inhalation exposure of rats to multiwalled carbon nanotubes: toxic effects are determined by density of agglomerate structures, not fibrillar structures. Toxicol Sci. 113:226–242.
- Pauluhn J, Rosenbruch M. 2015. Lung burdens and kinetics of multi-walled carbon nanotubes (Baytubes) are highly dependent on the disaggregation of aerosolized MWCNT. Nanotoxicology. 9:242–252.
- Pfeifer S, Müller T, Weinhold K, Zikova N, Martins dos Santos S, Marinoni A, Bischof OF, Kykal C, Ries L, Meinhardt F, et al. 2016. Intercomparison of 15 aerodynamic particle size spectrometers (APS 3321): uncertainties in particle sizing and number size distribution. Atmos Meas Tech. 9:1545–1551.
- Podgorski A, Gradon L. 2012. The mechanics of a fibrous aerosol particle. Aerosol Sci Technol. 46:i–ii.
- Poland CA, Duffin R, Kinloch I, Maynard A, Wallace WAH, Seaton A, Stone V, Brown S, MacNee W, Donaldson K. 2008. Carbon nanotubes introduced into the abdominal cavity of mice show asbestos-like pathogenicity in a pilot study. Nat Nanotechnol. 3:423–428.
- Porter DW, Hubbs AF, Chen BT, McKinney W, Mercer RR, Wolfarth MG, Battelli L, Wu N, Sriram K, Leonard S, et al. 2012. Acute pulmonary dose-responses to inhaled multi-walled carbon nanotubes. Nanotoxicology. 7:1179–1194.
- Porter DW, Hubbs AF, Mercer RR, Wu N, Wolfarth MG, Sriram K, Leonard S, Battelli L, Schwegler-Berry D, Friend S, et al. 2010. Mouse pulmonary dose- and time course-responses induced by exposure to multi-walled carbon nanotubes. Toxicology. 269:136–147.
- Poulsen SS, Knudsen KB, Jackson P, Weydahl IEK, Saber AT, Wallin H, Vogel U. 2017. Multi-walled carbon nanotube-physicochemical properties predict the systemic acute phase response following pulmonary exposure in mice. PLoS One. 12:e0174167.
- Prodi V, Mularoni A. 1985. Electrostatic lung deposition experiments with humans and animals. Ann Occup Hyg. 29:229–240.
- Rostami AA. 2009. Computational modeling of aerosol deposition in respiratory tract: a review. Inhal Toxicol. 21:262–290.
- Ryman-Rasmussen JP, Cesta MF, Brody AR, Shipley-Phillips JK, Everitt JI, Tewksbury EW, Moss OR, Wong BA, Dodd DE, Andersen ME, et al. 2009. Inhaled carbon nanotubes reach the subpleural tissue in mice. Nat Nanotechnol. 4:747–750.
- Sarangi B, Aggarwal SG, Sinha D, Gupta PK. 2016. Aerosol effective density measurement using scanning mobility particle sizer and quartz crystal microbalance with the estimation of involved uncertainty. Atmos Meas Tech. 9:859–875.
- Scheckman J, McMurry P. 2011. Deposition of silica agglomerates in a cast of human lung airways: enhancement relative to spheres of equal mobility and aerodynamic diameter. J Aerosol Sci. 42:508–516.
- Schmid O, Cassee FR. 2017. On the pivotal role of dose for particle toxicology and risk assessment: exposure is a poor surrogate for delivered dose. Part Fibre Toxicol. 14:52.
- Schmid O, Stoeger T. 2016. Surface area is the biologically most effective dose metric for acute nanoparticle toxicity in the lung. J Aerosol Sci. 99:133–143.
- Schwotzer D, Ernst H, Schaudien D, Kock H, Pohlmann G, Dasenbrock C, Creutzenberg O. 2017. Effects from a 90-day inhalation toxicity study with cerium oxide and barium sulfate nanoparticles in rats. Part Fibre Toxicol. 14:23.
- Sébastien P. 1991. Pulmonary deposition and clearance of airborne mineral fibers. In: Liddell D, Miller K, editors. Mineral fibers and health. Boca Raton (FL): CRC Press.
- Shin JH, Han SG, Kim JK, Kim BW, Hwang JH, Lee JS, Lee JH, Baek JE, Kim TG, Kim KS, et al. 2015. 5-Day repeated inhalation and 28-day post-exposure study of graphene. Nanotoxicology. 9:1023–1031.
- Shachar-Berman L, Ostrovski Y, Koshiyama K, Wada S, Kassinos SC, Sznitman J. 2019. Targeting inhaled fibers to the pulmonary acinus: opportunities for augmented delivery from in silico simulations. Eur J Pharm Sci. 137:105003.
- Shvedova AA, Kisin E, Murray AR, Johnson VJ, Gorelik O, Arepalli S, Hubbs AF, Mercer RR, Keohavong P, Sussman N, et al. 2008. Inhalation vs. aspiration of single-walled carbon nanotubes in C57BL/6 mice: inflammation, fibrosis, oxidative stress, and mutagenesis. Am J Physiol Lung Cell Mol Physiol. 295:L552–L565.
- Stapleton P, Minarchick V, Cumpston A, Mckinney W, Chen B, Sager T, Frazer D, Mercer R, Scabilloni J, Andrew M, et al. 2012. Impairment of coronary arteriolar endothelium-dependent dilation after multi-walled carbon nanotube inhalation: A time-course study. IJMS. 13(12):13781–13803. doi:10.3390/ijms131113781.
- Sturm R. 2014. Theoretical deposition of nanotubes in the respiratory tract of children and adults. Ann Transl Med. 2:6.
- Sturm R. 2018. Deposition of carbon nanotubes in the human respiratory tract: a theoretical approach. J Public Health Emerg. 2:19–19.
- Sturm R, Hofmann W. 2009. A theoretical approach to the deposition and clearance of fibers with variable size in the human respiratory tract. J Hazard Mater. 170:210–218.
- Su WC, Cheng YS. 2015. Estimation of carbon nanotubes deposition in a human respiratory tract replica. J Aerosol Sci. 79:72–85.
- Taquahashi Y, Ogawa Y, Takagi A, Tsuji M, Morita K, Kanno J. 2013. An improved dispersion method of multi-wall carbon nanotube for inhalation toxicity studies of experimental animals. J Toxicol Sci. 38:619–628.
- Tavakoli F, Olfert JS. 2014. Determination of particle mass, effective density, mass–mobility exponent, and dynamic shape factor using an aerodynamic aerosol classifier and a differential mobility analyzer in tandem. J Aerosol Sci. 75:35–42.
- Tian L, Ahmadi G. 2016. Transport and deposition of nano-fibers in human upper tracheobronchial airways. J Aerosol Sci. 91:22–32.
- Timbrell V. 1982. Deposition and retention of fibres in the human lung. Ann Occup Hyg. 26:347–369.
- Trubetskaya A, Beckmann G, Wadenbäck J, Holm JK, Velaga SP, Weber R. 2017. One way of representing the size and shape of biomass particles in combustion modeling. Fuel. 206:675–683.
- van Berlo D, Clift M, Albrecht C, Schins R. 2012. Carbon nanotubes: an insight into the mechanisms of their potential genotoxicity. Swiss Med Wkly. 142:w13698.
- Wang J, Bahk YK, Chen SC, Pui D. 2015. Characteristics of airborne fractal-like agglomerates of carbon nanotubes. Carbon. 93:441–450.
- Wiedensohler A. 1988. An approximation for the bipolar charge distribution for particles in the submicron size range. J Aerosol Sci. 19:387–389.
- Yeh HC, Cuddihy RG, Phalen RF, Chang IY. 1996. Comparison of calculated respiratory tract deposition of particles based on the proposed NCRP model and the new ICRP66 model. Aerosol Sci Technol. 25:134–140.
- Zeinabad HA, Zarrabian A, Saboury AA, Alizadeh AA, Falahati M. 2016. Interaction of single and multi wall carbon nanotubes with the biological systems: tau protein and PC12 cells as targets. Sci Rep. 6:29644.
- Zelenyuk A, Imre D. 2007. On the effect of particle alignment in the DMA. Aerosol Sci Technol. 41:112–124.
- Zhou Y, Su WC, Cheng YS. 2007. Fiber deposition in the tracheobronchial region: experimental measurements. Inhal Toxicol. 19:1071–1078.