Abstract
5-aminolevulinic acid (5-ALA) is contained in all organisms and a starting substrate for heme biosynthesis. Since administration of 5-ALA specifically leads cancer cells to accumulate protoporphyrin IX (PpIX), a potent photosensitizer, we tested if 5-ALA also serves as a thermosensitizer. 5-ALA enhanced heat-induced cell death of cancer cell lines such as HepG2, Caco-2, and Kato III, but not other cancer cell lines including U2-OS and normal cell lines including WI-38. Those 5-ALA-sensitive cancer cells, but neither U2-OS nor WI-38, accumulated intracellular PpIX and exhibited an increased reactive oxygen species (ROS) generation under thermal stress with 5-ALA treatment. In addition, blocking the PpIX-exporting transporter ABCG2 in U2-OS and WI-38 cells enhanced their cell death under thermal stress with 5-ALA. Finally, a ROS scavenger compromised the cell death enhancement by 5-ALA. These suggest that 5-ALA can sensitize certain cancer cells, but not normal cells, to thermal stress via accumulation of PpIX and increase of ROS generation.
Graphical Abstract
5-ALA treatment leads cancer cells to accumulate PpIX (heme precursor), resulting in ROS increase under thermal stress and thereby enhancing heat-induced cancer cell death.
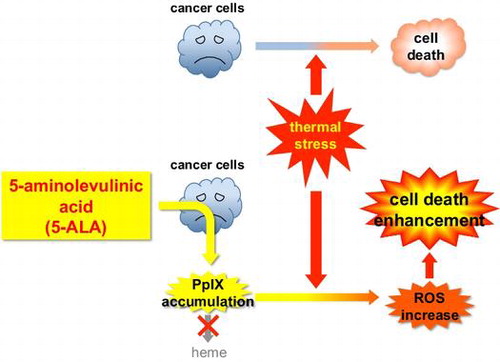
Selective killing of cancer cells is the ultimate goal for cancer therapy, but it is still difficult to develop such therapy without any side effects on non-cancerous cells. One of the therapeutic approaches to target cancer cells for selective killing is cancer-specific delivery of certain sensitizer compound(s) that can be accumulated specifically in cancer cells and sensitize them to physical or chemical treatments. Photodynamic therapy (PDT) is based on such an approach—a local endoscopic treatment using a photochemical reaction induced by an oncotropic photosensitizer and a laser irradiation.Citation1) PDT has been shown to be effective in the ablation of superficial cancers, but long-lasting photosensitivity derived from the photosensitizer is a serious disadvantage. To circumvent this, cancer-specific accumulation of protoporphyrin IX (PpIX), an endogenous photosensitizer, by temporal administration of its precursor 5-aminolevulinic acid (5-ALA) has been applied to PDT.Citation2)
5-ALA is widely distributed in both plant and animal cells, and it is the common precursor of tetrapyrrole compounds such as heme and chlorophylls. In animal cells, 5-ALA is metabolized via several steps that finally lead to heme production in mitochondria. 5-ALA administration can increase the heme production and thereby enhance the activity of hemoproteins. For example, Ogura et al. demonstrated an improvement of cytochrome c oxidase (COX) activity by 5-ALA administration.Citation3) Since COX activity is decreased with aging and also in various diseases including cancer, 5-ALA would be a promising candidate of treatment option for these diseases. There is no report of toxic side effects caused by 5-ALA administration, and 5-ALA supplementation has been used in several fields to date.Citation4–6) Besides those nutritional and metabolic effects, administration of 5-ALA enhances the cancer-specific accumulation of PpIX, an immediate precursor of heme.Citation7) 5-ALA-induced PpIX can be fluoresced and visualized in neoplastic regions. This technique exploits 5-ALA-induced differences in fluorescent signatures between normal and cancer tissues, and therefore, has been used clinically for the detection of malignant tissues by 5-ALA-induced fluorescence and for the selective phototoxic destruction of malignant cells by 5-ALA-induced PDT.Citation2,8,9) 5-ALA-induced PDT has been intensively investigated and applied to variety of cancers including skin and brain cancers since 5-ALA has advantages such as no side effects, chemical stability, and short-term photosensitivity compared to other photosensitizers.Citation2) However, as PDT depends solely on light irradiation, its application has been limited to cancerous regions that can be accessed by the irradiation devices.
The cancer-specific sensitization effect and non-toxic feature of 5-ALA prompted us to examine the possibility of 5-ALA being an efficient sensitizer under thermal stress, and as a candidate for novel application(s) of 5-ALA to cancer therapy such as hyperthermia. Hyperthermia has been widely recognized and practiced as a less invasive and less side effect therapy for cancer. Especially, its advantage as an effective sensitizer for radiotherapyCitation10) and chemotherapyCitation11) has led hyperthermia to an adjuvant with these therapies for broad types of cancer. The rationale of hyperthermia is based on a direct killing effect at temperatures above 41–42 °C.Citation12) However, the thermal dose–response relation varies among cell lines, and furthermore depends on microenvironmental factors such as pH.Citation13) Also, for many cancers, suboptimal thermal doses limit radiosensitization.Citation14) Thus, any small molecules or compounds to enhance hyperthermic effect have the potential to improve not only hyperthermia itself but combined therapies such as thermoradiotherapy.
If 5-ALA can enhance hyperthermic effect in cancer cells, combination of 5-ALA administration and hyperthermia would provide a solution for both hyperthermia and 5-ALA-induced PDT by compensating each other for their limitations. In this report, we show that exogenous 5-ALA enhances cell death under thermal stress in certain types of human cancer cell lines but not in normal human fibroblasts. The enhancement of cell death was accompanied by accumulation of intracellular PpIX and by increased generation of reactive oxygen species (ROS). This is the first demonstration that 5-ALA can sensitize cancer cells to thermal stress and suggests a therapeutic potential of improving the efficacy of hyperthermia.
Materials and methods
Cells and cell culture
HEK293 (human embryonic kidney cells transformed with adenovirus), HT1080 (human fibrosarcoma), and U2-OS (human osteosarcoma) were provided by M. Ohtsubo. HepG2 (human hepatocellular carcinoma), Caco-2 (human colorectal adenocarcinoma), KATO III (human gastric carcinoma), MCF7 (human breast adenocarcinoma), and A431 (human epidermoid carcinoma) were derived from M. Nakajima’s cell bank in M. D. Anderson Cancer Center, The University of Texas, Houston, Texas. WI-38 and MRC5 (human normal diploid fibroblasts) were provided by RIKEN BioResource Center (Tsukuba, Japan). NIH3T3 cells (mouse normal fibroblasts) were provided by C. Sherr.
All cells used were grown in Dulbecco’s modified Eagle medium (DMEM) containing 10% fetal bovine serum (FBS) with 5% CO2. 5-aminolevulinic acid hydrochloride (5-ALA) was purchased from Cosmo Bio Co., Ltd, (Tokyo, Japan). A final concentration of 0.2 mM of 5-ALA was added to the culture at 37 °C for 2 h in DMEM without FBS, and then the cells were grown at 37 or 42 °C for 24 h in DMEM with 10% FBS. 10 μM of fumitremorgin C (FTC; BioAustralis Fine Chemicals Pty. Ltd, Smithfield, Australia) was added to the culture at the same time as 5-ALA addition. In N-acetylcysteine (NAC; EMD Chemicals, Inc., San Diego, CA, USA) treatment experiments, NAC was added at final concentration of 4 mM 2 h before the addition of 5-ALA, removed from the culture during the 2-h incubation with 5-ALA and without FBS, and added at final concentration of 0.2 mM during the 24-h incubation with 5-ALA and 10% FBS.
Trypan blue staining
Cells were grown with or without 5-ALA in DMEM at 37 and 42 °C under 5% CO2 for 24 h and then trypsinized. After an equal volume of 0.4% trypan blue solution (Life Technologies Japan, Ltd, Tokyo, Japan) was added to the cell suspension, trypan blue-stained (dead) and unstained (live) cells were counted under microscope.
HPLC analysis of protoporphyrin IX (PpIX)
Cells were grown and harvested as above, rinsed with 4 ml of phosphate-buffered saline (PBS), and lysed with 500 μL of 0.1 M NaOH. 1.5 ml of N,N-dimetylformamide was added to the sample and then centrifuged at 13,000 rpm for 10 min. The supernatant was subjected to high-pressure liquid chromatography (HPLC) analysis.
PpIX was separated using an HPLC system (Type Prominence, Shimadzu, Kyoto, Japan) equipped with a reverse-phase C18 column (CAPCELL PAK, C18, SG300, 5 μm, 4.6 mm × 250 mm, Shiseido, Tokyo). Elution was started with solvent A (1 M ammonium acetate containing 12.5% acetonitrile and pH 5.2) for 5 min, then a linear gradient of 0–100% solvent B (50 mM ammonium acetate containing 80% acetonitrile, pH 5.2) in solvent A for 25 min, followed by 100% solvent B for 10 min. Porphyrins were detected with a fluorospectrometer (λex = 404 nm; λdet = 624 nm) and PpIX concentration was estimated from the area of PpIX peak (retention time = 33.5 min) compared with a standard PpIX sample.
Western blot analysis
Cells were grown and harvested as above, rinsed once with PBS, and lysed with SDS sample buffer [50 mM Tris–HCl (pH 6.8), 2% SDS, 2.5% β-mercaptoethanol, 10% glycerol, and 0.01% bromophenol blue]. After boiled at 100 °C for 5 min, the cell lysates were subjected to SDS-PAGE using 12% (for detecting frataxin) or 8% (for ferrochelatase and ABCG2) SDS-polyacrylamide gel, followed by western blot analysis using antibodies against human ferrochelatase (GeneTex, Inc., Irvine, CA, USA), frataxin (Santa Cruz Biotechnology, Santa Cruz, CA, USA), ABCG2 (Covance Inc., Princeton, NJ, USA), or Hsc70/Hsp70 (generous gift of K. Ohtsuka). In the case of ABCG2 immunoblots, cells were first lysed with lysis buffer [50 mM Tris–HCl (pH 7.4), 1% Triton X-100, 20 mM N-ethylmaleimide, 1 × Protease Inhibitor Cocktail (Nacalai Tesque, Inc., Kyoto, Japan)], and then mixed with SDS sample buffer and boiled before subjected to SDS-PAGE.
Detection of ROS
Following the 5-ALA (0–2 mM) treatment of cells in DMEM without FBS at 37 °C for 2 h, 5 μM of 3′-(p-aminophenyl) fluorescein (APF, Sekisui Medical Co., Ltd, Tokyo, Japan) was added to the DMEM containing 10% FBS with or without 5-ALA. After a 24-h incubation at 37 or 42 °C, cells were harvested, suspended in PBS, and subjected to flow cytometry using a FACScalibur (BD Biosciences, San Jose, CA, USA). APF-derived fluorescence (λ = 515 nm) was detected with a FL1 detector, under the same instrument setting for each cell line where the peak of FL1 histogram for the cells grown at 37 °C without 5-ALA was adjusted to 200 linear value.
Statistical analysis
Student t-tests were used to identify significant differences between data groups in the cell death assays. Results with p < 0.05 were considered to represent statistically significant differences and indicated with asterisks in figures.
Results
5-ALA enhances cell death under thermal stress in certain types of cancer cell lines
We tested the effect of 5-ALA on cell death under thermal stress in various cell lines including human cancer-derived and normal cell lines that are as follows: HEK293, human embryonic kidney cells transformed with adenovirus; HepG2, human hepatocellular carcinoma; Caco-2, human colorectal adenocarcinoma; KATO III, human gastric carcinoma; HT1080, human fibrosarcoma; U2-OS, human osteosarcoma; MCF7, human breast adenocarcinoma; A431, human epidermoid carcinoma; WI-38 and MRC5, human normal diploid fibroblasts; and NIH3T3, mouse normal fibroblasts. These cells were grown at 37 or 42 °C for 24 h in the absence and presence of 5-ALA and assayed for cell death. Fig. (A) shows that in four cancer cell lines (HEK293, HepG2, Caco-2, and KATO III), cell death under thermal stress was enhanced with increasing concentration of 5-ALA. The enhancement was twofold to threefold compared to 0 mM 5-ALA control. Patterns of cell death under thermal stress in these cell lines were shown to be mostly necrosis with a small population of apoptosis by annexin V-propidium iodide staining and 5-ALA treatment increased both necrosis and apoptosis (data not shown). In contrast, in other cancer cell lines (HT1080, U2-OS, MCF7, and A431) and normal cell lines (WI-38, MRC5, and NIH3T3) tested, 5-ALA had little effect on their cell death under thermal stress (Fig. (B) and (C)). In all cases, 5-ALA did not affect the cell death under the normal temperature (37 °C). These results demonstrate that 5-ALA enhances the cell death under thermal stress in certain types of cancer cell lines.
Fig. 1. 5-ALA enhances cell death of certain cancer cell lines but not normal cell lines under thermal stress.
Notes: Cells were grown with indicated concentrations of 5-ALA at 37 (open bars) and 42 °C (closed bars) for 24 h and counted for cell death by trypan blue staining. (A) Human transformed cell line HEK293 and cancer cell lines HepG2, Caco-2, and KATO III. (B) Human cancer cell lines HT1080, U2-OS, MCF7, and A431. (C) Normal human cell lines WI-38, MRC5, and mouse NIH3T3 cells. Bars represent mean ± SEM (n = 3–5). Brackets: *p < 0.05; **p < 0.01.
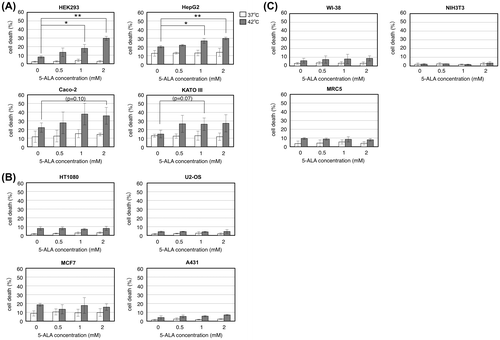
Enhancement of cell death under thermal stress by 5-ALA is accompanied by intracellular protoporphyrin IX (PpIX) accumulation
Since the administration of 5-ALA leads cancer cells to accumulate its downstream metabolite protoporphyrin IX (PpIX), which can generate ROS upon light irradiation and thereby act as a photosensitizer,Citation15) we next measured intracellular accumulation of PpIX under the same culture conditions as above. In those cancer cell lines (HEK293, HepG2, Caco-2, and KATO III) whose cell death under thermal stress was enhanced by 5-ALA treatment, intracellular PpIX was markedly accumulated at 42 °C in the presence of 5-ALA (Fig. (A)). When grown at 37 °C, these cell lines except for HEK293 also exhibited accumulation of PpIX with increasing concentration of 5-ALA. Especially in HepG2 and KATO III cells, we observed a marked accumulation of PpIX at 37 °C, up to the level equivalent to that at 42 °C. On the other hand, 5-ALA treatment did not cause any substantial accumulation of PpIX in U2-OS and WI-38 (Fig. (B)), whose cell death was not affected by 5-ALA. These data suggest that the enhancement of cell death under thermal stress by 5-ALA is associated with accumulation of PpIX. The accumulation of PpIX itself, however, does not necessarily enhance the cell death, since at normal growth temperature (37 °C), the cell death of HepG2 and KATO III was not enhanced by 5-ALA (Fig. (A)) in spite of the accumulation of PpIX. This suggests that the PpIX accumulation combined with thermal stress enhances the cell death in those cell lines.
Fig. 2. Effect of 5-ALA on intracellular PpIX accumulation.
Notes: Cells were grown with indicated concentrations of 5-ALA at 37 (open bars) and 42 °C (closed bars) for 24 h and the cell lysates were subjected to HPLC analysis of intracellular PpIX amount (pmol/104 cells). (A) Human transformed cell line HEK293 and cancer cell lines HepG2, Caco-2, and KATO III. (B) Human cancer cell line U2-OS and normal human cell line WI-38. Bars represent mean ± SEM (n = 3–5).
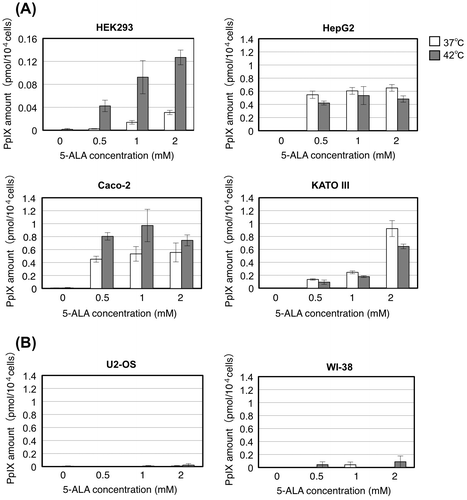
Also, we observed that level and kinetics of the PpIX accumulation vary among the cell lines. Especially in HEK293, the PpIX accumulation level was quite low compared to HepG2, Caco-2, and KATO III. Even so, HEK293 cells exhibited marked increase of cell death along with the PpIX accumulation (Figs. (A) and (A)), suggesting that this cell line is highly sensitive to intracellular PpIX level. In contrast, in HepG2, cell death increased but PpIX level did not with increasing concentration of 5-ALA, and in KATO III, PpIX levels increased but cell death did not change with 0.5–2 mM of 5-ALA. Thus, the intracellular PpIX levels are not linearly correlated with the cell death enhancement, which might ascribed to different intracellular distributions of PpIX (e.g. cytoplasmic vs. mitochondrial, see below and Discussion).
In order to discern whether PpIX directly enhances the cell death under thermal stress, we added PpIX instead of 5-ALA to the culture. We observed that, in the four cancer cell lines (HEK293, HepG2, Caco-2, and KATO III) which were sensitized by 5-ALA, cell death under thermal stress was enhanced with increasing concentration of exogenous PpIX (Fig. (A)). 1 μM of exogenous PpIX caused the cell death enhancement equivalent to that with 2 mM 5-ALA in these cells. In contrast, in U2-OS and WI-38 cells, which were insensitive to 5-ALA, the addition of PpIX to the culture hardly showed any effect on their cell death (Fig. (B)). Quantification of PpIX amount revealed that intracellular PpIX was indeed accumulated with the increasing concentration of exogenous PpIX in those 5-ALA-sensitive cells (HEK293, HepG2, Caco-2, and KATO III) but to somewhat less extent than in the case with 2 mM 5-ALA (Fig. (C)). We observed again that HEK293 showed a marked cell death enhancement at relatively small PpIX amounts, which implies the hypersensitivity of this cell line to PpIX. Also, we observed that PpIX was accumulated to the equivalent level at both 37 and 42 °C in HepG2 and KATO III cells with the addition of PpIX to the culture, which is similar to the case with 5-ALA treatment (Fig. (A)). Nonetheless, a marked cell death enhancement by exogenous PpIX in these cells was observed only at 42 °C (Fig. (A)), suggesting again that the combination of PpIX accumulation and thermal stress leads to the cell death enhancement.
Fig. 3. PpIX directly enhances cell death of certain cancer cell lines under thermal stress.
Notes: Human transformed cell line HEK293 and cancer cell lines HepG2, Caco-2, and KATO III (A, C) or human cancer cell line U2-OS and normal human cell line WI-38 (B, D) were grown with indicated concentrations of PpIX or 2 mM 5-ALA at 37 (open bars) and 42 °C (closed bars) for 24 h and counted for cell death by trypan blue staining (A, B) or the cell lysates were subjected to HPLC analysis of intracellular PpIX amount (C, D). Bars represent mean ± SEM (n = 3–5). Brackets: *p < 0.05; **p < 0.01.
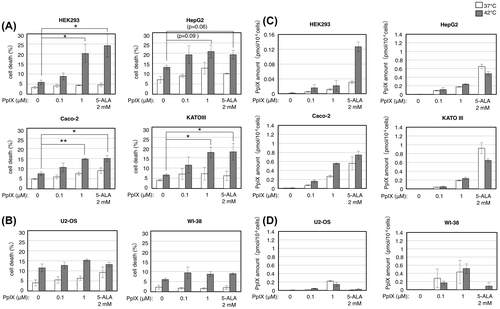
On the other hand, addition of exogenous PpIX to U2-OS and WI-38 cells also resulted in accumulation of intracellular PpIX while the addition of 2 mM 5-ALA did not (Fig. (D)). In fact, the accumulation of PpIX in WI-38 cells (ca. 0.5 pmol/104 cells with 1 μM exogenous PpIX) was equivalent to or more than that in those 5-ALA-sensitive cells (HEK293, HepG2, Caco-2, and KATO III) treated with exogenous PpIX. This and the results shown in Fig. (B), that the PpIX addition did not enhance the cell death of U2-OS and WI-38 cells, suggest that these cells can tolerate the PpIX accumulation to some extent (see below and Discussion).
Effect of 5-ALA on the expression of heme synthase ferrochelatase, Fe2+-carrier protein frataxin and PpIX-exporting transporter ABCG2
We attempted to obtain insight into the mechanism by which intracellular PpIX is accumulated in certain types of cancer cells. Since it has been reported that cancer-specific PpIX accumulation is mainly generated by ALA conversion rather than by initial ALA uptake,Citation16) we examined whether or not Fe2+ chelation step for converting PpIX to heme is suppressed and/or PpIX-exporting transporter is downregulated in those cancer cells which accumulate PpIX. Thus, we analyzed the expression of ferrochelatase (FeCh), which chelates ferrous iron and PpIX to form heme,Citation17) frataxin, a Fe2+-carrier protein essential for heme production,Citation18) and ABCG2, a major PpIX-exporting transporter.Citation19) First, as shown in Fig. (A), protein levels of FeCh were generally not affected by thermal stress and/or 5-ALA treatment in all the cell lines tested, except for a reduced FeCh expression level in KATO III at 42 °C with 2 mM 5-ALA. KATO III cells, nonetheless, accumulated PpIX equivalently at both 37 and 42 °C (Fig. (A)), indicating that FeCh expression level is not responsible for the PpIX accumulation. Next, Fig. (B) shows that frataxin expression in all the cancer cells tested was downregulated at 42 °C, regardless of 5-ALA treatment. Quite differently, in the normal cell line WI-38, frataxin expression was rather induced upon 5-ALA treatment at both temperatures. These suggest that in normal cells, heme production is stimulated by 5-ALA, while in cancer cells, heme production is suppressed under thermal stress. Finally, as shown in Fig. (C), ABCG2 expression was mostly constant regardless of the temperature or 5-ALA treatment in all the cell lines tested, except for HEK293 and Caco-2, in which the expression was downregulated at 42 °C. Thus, the relationship between the expression of frataxin and ABCG2 and the intracellular PpIX accumulation upon 5-ALA treatment varies among the cell lines: in HEK293 and Caco-2 cells, both proteins were down-regulated and PpIX was accumulated at 42 °C; in WI-38 cells, both proteins were expressed at 42 °C and PpIX was not accumulated; in other cell lines whose frataxin expression was downregulated and ABCG2 expression was not at 42 °C, HepG2 and Kato III cells accumulated PpIX similarly at both temperature while U2-OS showed no PpIX accumulation. Taken together, these results suggest that the mechanism underlying the PpIX accumulation is cell type-dependent and does not solely depend on suppression of frataxin or ABCG2 expression level.
Fig. 4. Effect of 5-ALA on ferrochelatase, frataxin and ABCG2 expression under thermal stress.
Notes: Cells were grown with indicated concentrations of 5-ALA at 37 and 42 °C for 24 h and the cell lysates were subjected to western analysis using antibodies against ferrochelatase (FeCh) (A), frataxin (B), and ABCG2 (C). α-tubulin expression was analyzed in all the experiments as internal control.
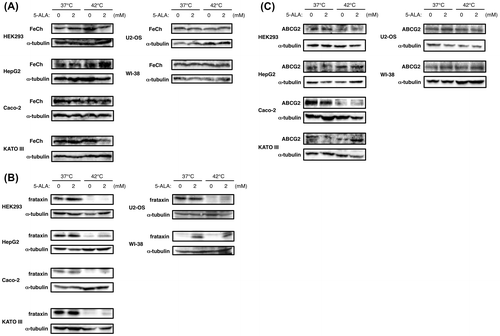
Blocking ABCG2 in 5-ALA-insensitive cells induces the enhancement of cell death by 5-ALA under thermal stress
Although the amount of ABCG2 expression was mostly constant in either 5-ALA-sensitive or 5-ALA-insensitive cell lines we tested, the function of the transporter could be impaired. We reasoned that blocking ABCG2 function would further enhance the cell death with 5-ALA treatment and thermal stress if the transporter is functional. We therefore examined the effect of fumitremorgin C (FTC), a specific and potent inhibitor of ABCG2,Citation20) on the cell death enhancement by 5-ALA under thermal stress. Fig. (A) shows that FTC treatment combined with 5-ALA treatment and thermal stress drastically enhanced the cell death of U2-OS and WI-38 cells, which were shown to be insensitive to 5-ALA in previous experiments. The combination of FTC and 5-ALA treatments also led these cells to markedly accumulate intracellular PpIX at both 37 and 42 °C (Fig. (B)). The PpIX accumulation at 42 °C was about 2.5-fold when compared to the case with addition of exogenous PpIX in both cells (Fig. (D)), indicating that there is a threshold level for these cells to tolerate intracellular PpIX. These results strongly suggest that U2-OS and WI-38 cells can efficiently export excess intracellular PpIX via functional ABCG2 and therefore show tolerance to 5-ALA treatment under thermal stress. And once again, the results suggest that the combination of PpIX accumulation and thermal stress enhances the cell death.
Fig. 5. Blocking ABCG2 induces cell death enhancement by 5-ALA under thermal stress in U2-OS and WI-38 cells but not in the 5-ALA-sensitive cells.
Notes: U2-OS and WI-38 cells were grown with indicated combinations of 5-ALA and an ABCG2 inhibitor fumitremorgin C (FTC) at 37 (open bars) and 42 °C (closed bars) for 24 h and counted for cell death by trypan blue staining (A) and the cell lysates were subjected to HPLC analysis of intracellular PpIX amount (B). 5-ALA-sensitive cells were grown with indicated combinations of 5-ALA and FTC at 37 and 42 °C for 24 h and counted for cell death by trypan blue staining (C). Bars represent mean ± SEM (n = 3–5). Brackets: *p < 0.05; **p < 0.01.
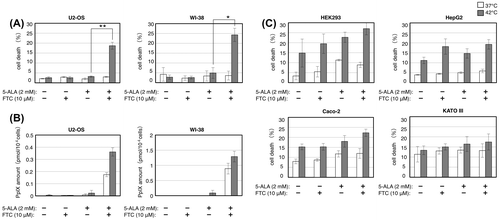
In contrast to the above results, Fig. (C) shows that FTC treatment caused a slight or moderate effect but not such a substantial effect, as above, on the cell death enhancement by 5-ALA under thermal stress in other 5-ALA-sensitive cancer cell lines (HEK293, HepG2, Caco-2, and KATO III). This and the expression level of ABCG2 in these cells (Fig. (C)) suggest that, although the ABCG2 expression was constant regardless of the temperature or 5-ALA treatment in HepG2 and KATO III cells, these cells accumulate PpIX upon 5-ALA treatment because their ABCG2 is dysfunctional. This is also consistent with the equivalent accumulation of PpIX at both 37 and 42 °C in these cells (Figs. (A) and (C)).
Cell death enhancement by 5-ALA treatment and thermal stress is correlated with increase of intracellular ROS generation in 5-ALA-sensitive cancer cell lines
To investigate a physiological correlation between the enhancement of cell death under thermal stress and the accumulation of PpIX by 5-ALA treatment, we assayed ROS generation. Initially, we employed a cell-permeable and relatively broad-range oxidation-detecting substrate, 2′,7′-dichlorodihydrofluorescein diacetate (DCF-DA), and observed that intracellular oxidization of DCF-DA was markedly enhanced upon 5-ALA treatment under thermal stress in HEK293 cells (see supplementary Fig. S1 online).
We then measured intracellular ROS generation using a more selective ROS indicator, 3′-(p-aminophenyl) fluorescein (APF), which reacts with limited species of ROS such as hydroxyl radical, peroxynitrite, and hypochlorite anions.Citation21,22) APF permeates into cells and fluoresces when oxidized by these ROS. Fig. shows that intracellular APF-derived fluorescence substantially increased under thermal stress with 5-ALA treatment in HEK293, HepG2, Caco-2, and KATO III cells, whereas there was little or slight change in the APF-derived fluorescence in U2-OS and WI-38 cells. In KATO III and WI-38 cells, the APF-derived fluorescence slightly increased at 37 °C in the presence of 5-ALA, indicating that 5-ALA treatment increases ROS generation without thermal stress to some extent in these cells. Nevertheless, the APF-derived fluorescence further increased at 42 °C with 2 mM 5-ALA in KATO III cells, while it was almost equivalent to that at 37 °C in WI-38 cells. These results demonstrate that 5-ALA treatment and thermal stress synergistically increase intracellular ROS generation in those cancer cell lines (HEK293, HepG2, Caco-2, and KATO III), which exhibit cell death enhancement and PpIX accumulation upon 5-ALA treatment under thermal stress.
Fig. 6. Effect of 5-ALA on ROS generation under thermal stress.
Notes: Cells were grown with indicated concentrations of 5-ALA and 5 μM 3′-(p-aminophenyl) fluorescein (APF) at 37 and 42 °C for 24 h, and subjected to flow cytometery. x axis shows the fluorescence (relative to that at 37 °C without 5-ALA, whose peak value is adjusted to 200) derived from APF oxidized by intracellular ROS.
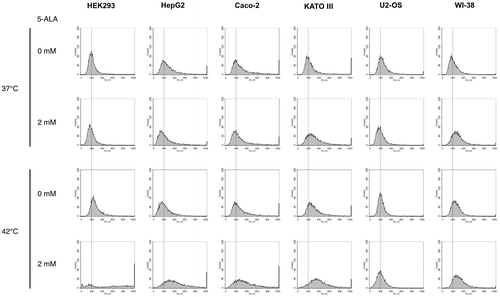
Finally, to further confirm that the increase in ROS generation is responsible for the cell death enhancement by 5-ALA, we tested the effect of an antioxidant N-acetylcysteine (NAC), which is a scavenger of free radicals as it interacts with ROS such as hydroxyl radical, hypochlorite anions, and H2O2,Citation23,24) on the cell death induced by 5-ALA treatment under thermal stress. As shown in Fig. , addition of NAC compromised the enhancement of cell death by 5-ALA under thermal stress in HEK293, HepG2, Caco-2, and KATO III cells, while NAC did not show any effect on cell death at 37 °C. In HepG2 cells, NAC addition also compromised the cell death at 42 °C without 5-ALA treatment in spite of no substantial increase in APF fluorescence under the same condition (Fig. ), suggesting that this cell line might bear increased generation of APF-nonreactive ROS or elevated intracellular oxidation independent of ROS increase under thermal stress. These results strongly suggest that the cell death enhancement by 5-ALA under thermal stress in those 5-ALA-sensitive cell lines is derived from the increase of ROS generation.
Fig. 7. N-acetylcysteine (NAC) compromises the cell death enhancement by 5-ALA under thermal stress.
Notes: Cells were grown with indicated combinations of 5-ALA and N-acetylcysteine (NAC) at 37 (open bars) and 42 °C (closed bars) for 24 h and counted for cell death by trypan blue staining. Bars represent mean ± SEM (n = 3–5). Brackets: *p < 0.05; **p < 0.01.
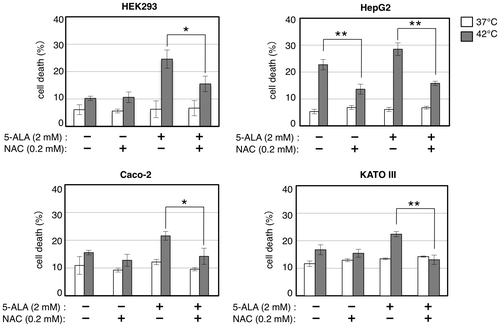
Discussion
We show that 5-ALA can enhance heat-induced cell death in certain cancer cell lines. There have been some reports on chemical enhancers of heat shock or hyperthermia. Anti-inflammatory drug indomethacin and its synthetic derivatives have been shown to lower the threshold temperature for hyperthermic radiosensitization,Citation25,26) and inhibitors of matrix metalloproteinase 3 (MMP-3) enhanced heat shock-induced cell death in HeLa cells.Citation27) In contrast to these compounds, 5-ALA is a natural amino acid with no toxic side effects on normal cells. The concentration of 5-ALA we added to the cell culture (up to 2 mM) is comparable to a standard dose of 5-ALA administration to human adults (30–60 mg/kg body weight).Citation28) Although its effect on hyperthermic cell death depends on certain types of cancer, 5-ALA is a safe and potent candidate for thermosensitizer.
Although we tested only a limited number of cancer cell lines, we could categorize them into two groups by whether they can be sensitized by 5-ALA to thermal stress or not. The 5-ALA-sensitive cell lines are either epithelial-derived (HEK293) or gastric intestinal-derived (HepG2, Caco-2, and KATO III), suggesting that 5-ALA is effective for cancers from these origins. However, further investigation with more varieties of cancer is necessary to determine the cancer type specificity.
The 5-ALA sensitization effect was accompanied by PpIX accumulation, and indeed, addition of exogenous PpIX exerted the same effect as 5-ALA did. Accumulated PpIX itself has little effect on cell death at 37 °C and enhances cell death only at 42 °C, suggesting that thermal stress induces the cytotoxicity of PpIX. Alternatively, 5-ALA might disturb cellular response(s) to thermal stress, such as induction of heat shock proteins, independently of PpIX accumulation. However, this seems to be unlikely since we observed normal induction of major heat shock proteins Hsp70 and Hsc70 under thermal stress, regardless of the 5-ALA concentration (see Supplementary Fig. S2 online). We also observed that the levels of intracellular PpIX accumulation were different and not linearly correlated with the cell death enhancement among the cell lines tested. Although we do not have direct evidence to explain these, the subcellular distribution of PpIX might vary depending on the cell lines: i.e. in some cell lines (such as HEK293) PpIX might be predominantly accumulated in mitochondria and exert cytotoxic effect more efficiently at relatively small amounts, while in others it might be predominantly accumulated in cytosol and therefore exert less effect. This could also explain why the direct administration of PpIX led to relatively high levels of its accumulation in U2-OS and WI-38 cells compared to the addition of 5-ALA which must be metabolized to PpIX in mitochondria (Fig. (D)).
Regarding the mechanism underlying PpIX accumulation under thermal stress, we observed no change in ferrochelatase (FeCh) expression but a marked reduction in frataxin protein level under thermal stress in all the cancer cell lines tested. Since frataxin is essential as a Fe2+ chaperone for FeCh which chelates Fe2+ to PpIX in the final step of heme biosynthesis,Citation18) its downregulation suggests a suppression of heme biosynthesis in those cancer cells under thermal stress. However, HepG2 and KATO III cells accumulated intracellular PpIX at both 37 and 42 °C and U2-OS cells did not accumulate PpIX at 42 °C, even though the frataxin expression was downregulated at 42 °C in these cells. In addition, the PpIX-exporting transporter ABCG2 was almost constantly expressed at both temperatures in most of the cell lines tested, suggesting that expression levels of frataxin and ABCG2 are not solely responsible for the PpIX accumulation. Indeed, the effect of an ABCG2 inhibitor fumitremorgin C on the cell death enhancement and PpIX accumulation implicated that those 5-ALA-sensitive cell lines express either reduced level of ABCG2 or constant level of defective ABCG2, while 5-ALA-insensitive cell lines express functional ABCG2. We therefore suggest that suppression of the PpIX-exporting system, either quantitatively or qualitatively, plays a key role in PpIX accumulation in the 5-ALA-sensitive cells. To confirm this, however, it is necessary to discern whether these cells bear loss-of-function mutation(s) in ABCG2 or other protein(s) involved in essential pathways for ABCG2 function such as membrane localization, dimerization, and modification.Citation29) With regard to this, we recently identified one missense mutation (Q141 K) in ABCG2 mRNA isolated from HepG2 cells (data not shown). ABCG2 has been also implicated in urate efflux transport, and this mutation, known as a common polymorphism associated with high urate levels and gout, has been shown to cause a reduced urate transport.Citation30) Although the effect of this mutation on the PpIX transport has not been known yet, it might be one of the potential causes of ABCG2 dysfunction in HepG2.
We observed increased ROS generation with 5-ALA treatment plus thermal stress in those 5-ALA-sensitive cell lines. Since a ROS scavenger N-acetylcysteine compromised the cell death enhancement, the increase of ROS generation is most likely the cause of the sensitivity to 5-ALA. It should be noted that the ROS generated upon light irradiation to PpIX is primarily singlet oxygen,Citation15) which is hardly detected by APF.Citation21) This suggests that the ROS generation resulted from 5-ALA treatment under thermal stress is mediated by a mechanism distinct from photosensitization by PpIX. It is also hard to assume that relatively mild heat treatment (42 °C) directly excites PpIX to generate ROS. One possibility is that H2O2, generated as a by-product of PpIX biosynthesis by protoporphyrinogen oxidase,Citation31) is responsible for generating hydroxyl radical via Fenton reaction. However, our observation that PpIX can directly enhance the cell death under thermal stress suggests that PpIX itself, not a byproduct of PpIX biosynthesis, is responsible for the ROS generation and resulting cell death under thermal stress. Therefore, we rather speculate that accumulation of PpIX might have a catastrophic impact on damaged mitochondrial function in cancer cells, leading to an elevated ROS generation.
In conclusion, we demonstrate for the first time that 5-ALA can enhance cell death in certain types of cancer cells under thermal stress and suggest that 5-ALA is a potent sensitizer for hyperthermia. Further analyses including in vivo studies in animal models, as well as clarifying molecular mechanism of ROS generation and the cell death enhancement by 5-ALA, should shed light on the clinical application of 5-ALA to hyperthermia and its combined cancer therapies.
Conflict of interest
The authors declare no conflict of interest.
Supplementary material
The supplemental material for this paper is available at http://dx.doi.org/10.1080/09168451.2014.975186.
Supplemental Materials
Download MS Word (196 KB)Acknowledgments
We thank Drs. S. Taketani, Y. Sueishi, M. Ishizuka, M. Takahashi, Y. Hagiya for helpful discussions, Drs. N. Higashi, M. Ohtsubo, K. Ohtsuka, C. Sherr and RIKEN BioResource Center for materials, and N. Nozawa, T. Sato, and S. Ohno for technical assistance.
Additional information
Funding
References
- Dolmans DE, Fukumura D, Jain RK. Photodynamic therapy for cancer. Nat. Rev. Cancer. 2003;3:380–387.10.1038/nrc1071
- Kennedy JC, Pottier RH. Endogenous protoporphyrin IX, a clinically useful photosensitizer for photodynamic therapy. J. Photochem. Photobiol., B. 1992;14:275–292.10.1016/1011-1344(92)85108-7
- Ogura S, Maruyama K, Hagiya Y, Sugiyama Y, Tsuchiya K, Takahashi K, Abe F, Tabata K, Okura I, Nakajima M, Tanaka T. The effect of 5-aminolevulinic acid on cytochrome c oxidase activity in mouse liver. BMC Res. Notes. 2011;4:66.10.1186/1756-0500-4-66
- Wang JP, Kim HJ, Chen YJ, Yoo JS, Cho JH, Kang DK, Hyun Y, Kim IH. Effects of delta-aminolevulinic acid and vitamin C supplementation on feed intake, backfat, and iron status in sows. J. Anim. Sci. 2009;87:3589–3595.10.2527/jas.2008-1489
- Wang JP, Lee JH, Jang HD, Yan L, Cho JH, Kim IH. Effects of δ-aminolevulinic acid and vitamin C supplementation on iron status, production performance, blood characteristics and egg quality of laying hens. J. Anim. Physiol. Anim. Nutr. (Berl). 2011;95:417–423.10.1111/jpn.2011.95.issue-4
- Morokuma Y, Yamazaki M, Maeda T, Yoshino I, Ishizuka M, Tanaka T, Ito Y, Tsuboi R. Hair growth stimulatory effect by a combination of 5-aminolevulinic acid and iron ion. Int. J. Dermatol. 2008;47:1298–1303.10.1111/ijd.2008.47.issue-12
- Ishizuka M, Abe F, Sano Y, Takahashi K, Inoue K, Nakajima M, Kohda T, Komatsu N, Ogura S, Tanaka T. Novel development of 5-aminolevurinic acid (ALA) in cancer diagnoses and therapy. Int. Immunopharmacol. 2011;11:358–365.10.1016/j.intimp.2010.11.029
- Peng Q, Warloe T, Berg K, Moan J, Kongshaug M, Giercksky KE, Nesland JM. 5-Aminolevulinic acid-based photodynamic therapy. Cancer. 1997;79:2282–2308.10.1002/(ISSN)1097-0142
- Stummer W, Pichlmeier U, Meinel T, Wiestler OD, Zanella F, Reulen HJ. Fluorescence-guided surgery with 5-aminolevulinic acid for resection of malignant glioma: a randomised controlled multicentre phase III trial. Lancet Oncol. 2006;7:392–401.10.1016/S1470-2045(06)70665-9
- van der Zee J, González D, van Rhoon GC, van Dijk JD, van Putten WL, Hart AA. Comparison of radiotherapy alone with radiotherapy plus hyperthermia in locally advanced pelvic tumours: a prospective, randomised, multicentre trial. Lancet. 2000;355:1119–1125.10.1016/S0140-6736(00)02059-6
- Urano M, Kuroda M, Nishimura Y. For the clinical application of thermochemotherapy given at mild temperatures. Int. J. Hyperthermia. 1999;15:79–107.10.1080/026567399285765
- Dewey WC. Arrhenius relationships from the molecule and cell to the clinic. Int. J. Hyperthermia. 1994;10:457–483.10.3109/02656739409009351
- Dewhirst MW, Ozimek EJ, Gross J, Cetas TC. Will hyperthermia conquer the elusive hypoxic cell? Implications of heat effects on tumor and normal-tissue microcirculation. Radiology. 1980;137:811–817.10.1148/radiology.137.3.7003650
- Dewhirst MW, Vujaskovic Z, Jones E, Thrall D. Re-setting the biologic rationale for thermal therapy. Int. J. Hyperthermia. 2005;21:779–790.10.1080/02656730500271668
- Yamamoto J, Yamamoto S, Hirano T, Li S, Koide M, Kohno E, Okada M, Inenaga C, Tokuyama T, Yokota N, Terakawa S, Namba H. Monitoring of singlet oxygen is useful for predicting the photodynamic effects in the treatment for experimental glioma. Clin. Cancer Res. 2006;12:7132–7139.10.1158/1078-0432.CCR-06-0786
- Krieg RC, Messmann H, Rauch J, Seeger S, Knuechel R. Metabolic characterization of tumor cell-specific protoporphyrin IX accumulation after exposure to 5-aminolevulinic acid in human colonic cells. Photochem. Photobiol. 2002;76:518–525.10.1562/0031-8655(2002)076<0518:MCOTCS>2.0.CO;2
- Taketani S, Fujita H. The ferrochelatase gene structure and molecular defects associated with erythropoietic protoporphyria. J. Bioenerg. Biomembr. 1995;27:231–238.10.1007/BF02110038
- Yoon T, Cowan JA. Frataxin-mediated iron delivery to ferrochelatase in the final step of heme biosynthesis. J. Biol. Chem. 2004;279:25943–25946.10.1074/jbc.C400107200
- Krishnamurthy P, Schuetz JD. The Role of ABCG2 and ABCB6 in porphyrin metabolism and cell survival. Curr. Pharm. Biotechnol. 2011;12:647–655.10.2174/138920111795163995
- Rabindran SK, Ross DD, Doyle LA, Yang W, Greenberger LM. Fumitremorgin C reverses multidrug resistance in cells transfected with the breast cancer resistance protein. Cancer Res. 2000;60:47–50.
- Setsukinai K, Urano Y, Kakinuma K, Majima HJ, Nagano T. Development of novel fluorescence probes that can reliably detect reactive oxygen species and distinguish specific species. J. Biol. Chem. 2003;278:3170–3175.10.1074/jbc.M209264200
- Cohn CA, Simon SR, Schoonen MA. Comparison of fluorescence-based techniques for the quantification of particle-induced hydroxyl radicals. Part. Fibre Toxicol. 2008;5:2.10.1186/1743-8977-5-2
- Aruoma OI, Halliwell B, Hoey BM, Butler J. The antioxidant action of N-acetylcysteine: its reaction with hydrogen peroxide, hydroxyl radical, superoxide, and hypochlorous acid. Free Radical Biol. Med. 1989;6:593–597.10.1016/0891-5849(89)90066-X
- Zafarullah M, Li WQ, Sylvester J, Ahmad M. Molecular mechanisms of N-acetylcysteine actions. Cell. Mol. Life Sci. 2003;60:6–20.10.1007/s000180300001
- Locke JE, Bradbury CM, Wei SJ, Shah S, Rene LM, Clemens RA, Roti Roti J, Horikoshi N, Gius D. Indomethacin lowers the threshold thermal exposure for hyperthermic radiosensitization and heat-shock inhibition of ionizing radiation-induced activation of NF-κB. Int. J. Radiat. Biol. 2002;78:493–502.10.1080/095530002317577312
- Sekhar KR, Sonar VN, Muthusamy V, Sasi S, Laszlo A, Sawani J, Horikoshi N, Higashikubo R, Bristow RG, Borrelli MJ, Crooks PA, Lepock JR, Roti Roti JL, Freeman ML. Novel chemical enhancers of heat shock increase thermal radiosensitization through a mitotic catastrophe pathway. Cancer Res. 2007;67:695–701.10.1158/0008-5472.CAN-06-3212
- Kato N, Kobayashi T, Honda H. Screening of stress enhancer based on analysis of gene expression profiles: enhancement of hyperthermia-induced tumor necrosis by an MMP-3 inhibitor. Cancer Sci. 2003;94:644–649.10.1111/cas.2003.94.issue-7
- Grant WE, Hopper C, MacRobert AJ, Speight PM, Bown SG. Photodynamic therapy of oral cancer: photosensitisation with systemic aminolaevulinic acid. Lancet. 1993;342:147–148.10.1016/0140-6736(93)91347-O
- Wakabayashi K, Nakagawa H, Adachi T, Kii I, Kobatake E, Kudo A, Ishikawa T. Identification of cysteine residues critically involved in homodimer formation and protein expression of human ATP-binding cassette transporter ABCG2: a new approach using the flp recombinase system. J. Exp. Ther. Oncol. 2006;5:205–222.
- Woodward OM, Kottgen A, Coresh J, Boerwinkle E, Guggino WB, Kottgen M. Identification of a urate transporter, ABCG2, with a common functional polymorphism causing gout. Proc. Nat. Acad. Sci. USA. 2009;106:10338–10342.10.1073/pnas.0901249106
- Ferreira GC, Dailey HA. Mouse protoporphyrinogen oxidase. Kinetic parameters and demonstration of inhibition by bilirubin. Biochem. J. 1988;250:597–603.