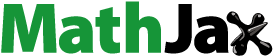
ABSTRACT
Carbonisation is one of the important methods to improve the utilisation of coal. The structural composition of coal plays an important role in coal carbonisation. This work used small-angle X-ray scattering (SAXS) to investigate the pore structure of three kinds of raw coals with different carbon content derived from Xingtai City, Hebei Province, China. The structures of the corresponding cokes obtained through carbonisation at 1200°C were investigated by elemental analysis and SAXS. In addition, the gasification reaction of the three cokes (C1, C2, C3) with CO2 were studied by thermogravimetric analysis. The SAXS results indicate that the surface fractal dimension increases while the pore size decreases, and the pore distribution became wider after carbonisation. In addition, all coals and cokes present a positive deviation from Porod’s law, indicating that there is a micro-fluctuation of electron density in the samples. Besides, it exhibites a negative correlation has been found between the degree of deviation from Porod’s law and the carbon content. The gasification reactivity of cokes C1 and C3 in CO2 are higher than that of C2, indicating that the pore structures affect the gas reactivity of the three different metamorphic degree cokes in CO2.
1. Introduction
Coal is deemed to be the main energy resource and therefore plays a crucial role in social development. Inefficient and extensive coal utilisation not only causes serious waste of energy but also bring a series of environmental pollution problems [Citation1]. It is urgent to meet the requirement of energy utilisation by promoting energy conservation and carbon reduction in the coalfield by improving the current energy structure, which is dominated by fossil fuels, as well as relieving the related environment pollution [Citation2–4].
Carbonisation has been considered as an effective way for cleaning and efficient conversion of coal [Citation5]. In addition, the coke obtained by carbonisation, as an indispensable raw material in steelmaking industries, is generally derived from solid products and obtained by high-temperature dry distillation of coal, which was regarded as one of the most mature transformations in the coal processing industry [Citation6,Citation7]. However, up to now, the development of the scientific theory of coal coking has been lagging behind the development of practical application. especially as the iron and steel industries are becoming more and more strict about the required coke quality. The traditional screening composition and chemical composition and other indicators that have been used could not meet the comprehensive evaluation and reflection of the quality of coke [Citation8–11]. The physical strength and chemical properties of coke are vital factors to ensure the smooth running of the blast furnace. Coke post-reaction strength (CSR) and coke reaction index (CRI) are important indicators of coke performance, with its pore structure significantly affecting the CRI. Research and exploration of structural changes of coal dry distillation in the process of coke production have recently attracted attention [Citation8,Citation9,Citation12–14].
Researchers have analysed the change of pore structure before and after dry distillation of coal by using conventional methods such as XRD, N2-adsorption, Raman and other characterisation methods. Despite their advantages, these conventional methods have also shown some limitations [Citation15–18]. As a non-destructive structural analysis method, in-situ small-angle X-ray scattering (in-situ SAXS) can comprehensively reveal the distribution characteristics of coal nanopores [Citation5,Citation19] and has therefore aroused intensive attraction in the field. Recently, several researchers have investigated the structure of coal via in-situ SAXS [Citation20–22]. Luo et al. [Citation23] studied the pore structure of superfine pulverised coal in different pyrolysis conditions based on SAXS. Xie et al. [Citation5] investigated the change of the physical structure of pores in two kinds of coals by in-situ SAXS and revealed three stages during carbonisation.
In the present work, SAXS was used to study the changes in the structure of three kinds of coal with different metamorphic degrees as well as cokes obtained by high-temperature dry distillation of the raw coals. The specific surface, porosity, pore size distribution, fractal dimension and other information of the coal samples have been investigated. Moreover, the change of pore structure characteristics after carbonisation and the effect of structure on coke reactivity have also been analysed. The relationship between the pore structure of coal with different metamorphic degrees and the gasification reactivity of the carbonised cokes in CO2 are revealed.
2. Experimental details
2.1. Materials preparation
Three kinds of coals with different carbon content selected from Xingtai City, Hebei Province, China were investigated as experimental samples. They are denoted as X01, X02, and X03 (with gradually increased carbon content). Under the protection of flowing nitrogen (0.5 L/min), the samples were heated from room temperature to 1200°C at a heating rate of 20°C/min. The obtained cokes of each coal sample after carbonisation are denoted as X01-1200, X02-1200 and X03-1200, respectively.
2.2. The synchrotron radiation SAXS experiment
The synchrotron radiation SAXS experiments were performed at the 1W2A station at the Beijing Synchrotron Radiation Facility (BSRF) with a detector Mar 165 CCD. A schematic diagram is shown in [Citation24]. As can be seen, the incident X-ray wavelength was 0.154 nm, the spot size was 1.4 mm (length) × 0.2 mm (width), the sample-detector distance was 1568 mm, and the exposure time 2 s. Fit2d software was used to convert the two-dimensional scattering image into a one-dimensional scattering datas [Citation25]. S software was used to calculate the fractal dimension and pore structure [Citation26].
2.3. The gasification experiments
The gasification experiments were conducted in a high-temperature furnace to investigate the mechanism of the reaction between the samples (X01, X02 and X03) and CO2. The cokes were ground and sieved to a particle size of 48 µm to eliminate the effects of diffusion on the gasification reaction. The reaction of the samples under the CO2 was evaluated via a thermogravimetric analyser. The samples after reaction are denoted as C1, C2 and C3, respectively. Typically, 5 mg of the sample were loaded into an Al2O3 crucible with height and diameter of 2 and 5 mm respectively. Specifically, the reaction temperature was increased at a fixed rate of 5 K/min up to 1273.15 K under CO2 (purity ≥99.99%) at a gas flow of 100 mL/min to limit heat transfer and minimize mass transfer effects.
The weight change caused by the reaction was recorded. The coke conversion ratio (x) was calculated by the equation:
(1)
(1) where mi represents the initial mass of the sample, mt is the sample weight at time t, and mf represents the remaining mass of the sample after gasification.
3. Results and discussion
3.1. Elemental analysis
The elemental analysis was performed with a German elemental analyzer Vario ELcube. The elemental analysis results of the raw coals and cokes are presented in . As can be seen, for the three raw coals of X01, X02 and X03, the carbon content increased after carbonisation, and the carbon content of the cokes was inversely proportional to the hydrogen content. This could be explained by the growth of a stack (crystallites) on the hexagonal carbon atom plane network and the subsequent formation of char during carbonisation, which resulted in dehydrogenation and aromatisation, releasing gas dominated by hydrogen. Upon elevating the temperature, the stack of carbon atom planar networks enlarged with compaction of the carbon skeleton and cracks shrinking during further polycondensation, finally forming coke [Citation5,Citation27]. Hence, the carbon content of the three samples increased while the hydrogen content decreased.
Table 1. Elemental analysis results of the raw coals and cokes.
3.2. SAXS Images
The two-dimensional SAXS images of raw coals and cokes are shown in . It can be seen from the SAXS images that all of the coals and cokes exhibites typical isotropic characteristics. Notably, the intensity of SAXS images of all cokes increases compared with that of the raw coals, which might be ascribed to a changing of pore structure during the carbonisation process [Citation5,Citation26,Citation27].
3.3. Fractal dimension
The irregularity of the internal structure of porous materials and the heterogeneity of the pore spatial distribution could be characterised via a fractal dimension which can be quantitatively described [Citation28]. Fractals are generally divided into surface fractals, mass fractals and pore fractals. The surface fractal (Ds) has been deemed to be an effective indicator for evaluating the degree of surface irregularity, and commonly ranges in value from 2 to 3 [Citation27–29]. The larger the Ds, the stronger the heterogeneity of the pore space distribution and the greater the surface roughness [Citation30]. Double logarithmic scattering curves of the coals and cokes are shown in . The curves have obvious straight line segments, indicating the existence of fractal structures. According to the slope of the linear segments, the fractal dimension α of the sample can be calculated from Iq = I0q-α [Citation27–29]. The results are listed in .
Table 2. Pore structure parameters of the raw coals and cokes.
The surface fractal dimensions of X01-1200 (2.69), X02-1200 (2.59) and X03-1200 (2.76) are all higher compared with those of X01 (2.53), X02 (2.57) and X03 (2.55) indicating different metamorphic degrees. This phenomenon indicates that the heterogeneity of the pore space distribution became stronger with larger surface roughness of the cokes on account of the carbonisation. A possible explanation could be generated gas derived from the coal (i.e. small-molecule gases such as carbon monoxide, hydrogen, methane and ethylene) during the high-temperature carbonisation process [Citation27,Citation29], which could increase the surface roughness and hence enhance the fractal dimension.
3.4. Pore size and distribution
Coal exhibits a complex pore structure. To further explore this, the pore size distribution () and the average pore diameter () of the samples were calculated by Monte Carlo fits [Citation31]. The results indicates that the average pore diameter of X01-1200 (13.28 nm) decreased by 57.2% compared with X01 (31.02 nm), that of X02-1200 (9.54 nm) decreased by 69.6% compared with X02 (31.43 nm) and that of X03-1200 (9.90 nm) decreased by 75.7% in comparison to X03 (40.81 nm). After carbonisation, the proportion of small pores of size 1∼2 nm increased, and the pore size distribution became wider. An explanation could be that the coals undergo a complex physical and chemical reaction during carbonisation [Citation27,Citation29]. In the initial stage of carbonisation, the coal is firstly degassed followed by dehydration and thermal decomposition to produce coal tar. Subsequently, the coal tar was transformed into semi-coke by dehydrogenation and aromatisation reactions in the middle stage of carbonisation. In the final step of carbonisation, the semi-coke further cracked and shrunk, producing porous coke with cracks. As a result, the proportion of small pores increased, while the average pore size decreased and the pore size distribution became wider. The results demonstrate that the pore physical structure changed on account of chemical reactions during the carbonisation, which is advantageous for understanding and controlling coal carbonisation.
3.5. Porod deviation
According to a principle of small-angle X-ray scattering, Porod’s law holds when there is an obvious phase interface between the two phases of the system. When there is a transitional fuzzy interface between the two phases, a Porod negative deviation occurs; when the two phases have microelectron density fluctuations, a Porod positive deviate occurs [Citation26,Citation32]. The positive deviation existed for all three cokes, indicating fluctuations in the density of microelectronics in the coke, which might be attributed to the presence of amorphous carbon [Citation33]. In order to obtain accurate scattering data for the pore structures, a Porod correction was employed to eliminate additional scattering from other structures.
The process of the Porod correction is shown in and the degree of Porod deviation is presented in . As can be seen, a smaller slope with a reduced degree of deviation, along with a decreased fluctuation of the microelectronic density inside the coke, could be associated with a sample structure similar to the graphite-microporous two-phase system [Citation33]. It can be seen from that the X01-1200 displayed the lowest slope (0.447), indicating that, among the three kinds of coal, the electronic density fluctuation of the coke produced by the distillation of coal X01 was a minimum and also showed the lowest proportion of amorphous carbon in the coal. Noteworthy, combined with the carbon content of coke (), is that the higher the carbon content, the smaller the degree of deviation, i.e. the carbon content of X01-1200 was a maximum (87.57%) among the three cokes, while showing a minimum slope of 0.447. It can therefore be reasonably deduced that there is a negative correlation between the degree of Porod deviation and the carbon content.
Figure 5. Schematic of correction of positive deviation from Porod’s law in SAXS (line a is a Porod positive deviation curve; line b is Porod curve after correction).

Table 3. The slope of porod correction.
3.6. Gasification tests
The carbon conversion curves are presented in , which shows that the gasification reaction curves of the three cokes displayed an almost similar trend with a gradually decreased conversion in the gasification process. However, there are some differences which suggest that the pore structure plays a significant role in controlling the gasification process. Samples C1 and C3 are typically more reactive in CO2, while sample C2 displays the lowest reaction rate, which is in good agreement with the pore structure of coke. The active pores of samples C1 and C3 are prone to connect and expand in the reaction, which increases the reaction rate via an increment in the specific surface area [Citation34]. The results reveal that the carbon content of coal exerts a great influence on its reactivity.
4. Conclusions
Three kinds of coal from Xingtai were selected for high-temperature carbonisation (1200°C) and the changes in their pore structure before and after carbonisation investigated. It is found that the three samples exhibited an increased surface fractal dimension with reduced pore size, an enhanced proportion of microporocity, and a broader range of pore distribution after high-temperature carbonisation. The three kinds of cokes all presented a Porod positive deviation, which indicated that, in addition to micropores, there are fluctuations in microelectronic density inside the coke, and the degree of Porod deviation negatively correlated to a certain extent with the carbon content, that is to say the coke exhibited better quality with a higher carbon content and a small deviation. The pore structures could affect the gas reactivity of the three different metamorphic degrees of the cokes in CO2.
Acknowledgements
The authors would like to thank the 1W2A station at Beijing Synchrotron Radiation Facility (BSRF) for supplying the SAXS measurement facilities. We thank Prof Zhihong Li (Institute of High Energy Physics, Chinese Academy of Sciences) for providing the S software.
Disclosure statement
No potential conflict of interest was reported by the author(s).
Additional information
Funding
References
- S.A. Stout, and A.P. Brey, Appraisal of coal- and coke-derived wastes in soils near a former manufactured gas plant, Jacksonville, Florida. Int. J. Coal Geol. 213 (2019), p. 103265.
- Z.-H. Ma, X.-Y. Wei, G.-H. Liu, F.-J. Liu, and Z.-M. Zong, Value-added utilization of high-temperature coal tar: A review. Fuel. 292 (2021), p. 119954.
- J. Xu, J. Dai, H. Xie, and C. Lv, Coal utilization eco-paradigm towards an integrated energy system. Energ. Policy 109 (2017), pp. 370–381.
- G. Yue, J. Lyu, and S. Li, Clean and highly-efficient utilization of coal. Front. Energy 15 (2021), pp. 1–3.
- F. Xie, Z. Li, W. Wang, D. Li, Z. Li, B. Lv, and B. Hou, In-situ SAXS study of pore structure during carbonization of non-caking coal briquettes. Fuel 262 (2020), p. 116547.
- M.J. Roberts, R.C. Everson, G. Domazetis, H.W.J.P. Neomagus, J.M. Jones, C.G.C.E. Van Sittert, G.N. Okolo, D.V. Niekerk, and J.P. Mathews, Density functional theory molecular modelling and experimental particle kinetics for CO2-char gasification. Carbon. N. Y. 93 (2015), pp. 295–314.
- J. Moulijn, and F. Kapteijn, Towards a unified theory of reactions of carbon with oxygen-containing molecules. Carbon. N. Y. 33 (1995), pp. 1155–1165.
- M. Grigore, R. Sakurovs, D. French, and V. Sahajwalla, Influence of mineral matter on coke reactivity with carbon dioxide. ISIJ Int. 46 (2006), pp. 503–512.
- R. Xu, H. Zheng, W. Wang, J. Schenk, and Z. Xue, Influence of iron minerals on the volume, strength, and CO2 gasification of ferro-coke. Energy Fuels 32 (2018), pp. 12118–12127.
- S. Gupta, Z. Ye, B.-c. Kim, O. Kerkkonen, R. Kanniala, and V. Sahajwalla, Mineralogy and reactivity of cokes in a working blast furnace. Fuel Process. Technol. 117 (2014), pp. 30–37.
- S.S. Gornostayev, O. Kerkkonen, and J. Härkki, Occurrence and composition of some mineral phases in the tuyere coke. ISIJ Int. 45 (2005), pp. 1–7.
- S.-M. Shin, I.-H. Jeong, and S.-M. Jung, Graphitisation effect of coke with changing annealing time and temperature on pore structure and apparent gasification rate with H2O. Ironmak. Steelmak. 45 (2018), pp. 739–746.
- X. Xing, G. Zhang, H. Rogers, P. Zulli, and O. Ostrovski, Effects of annealing on microstructure and microstrength of metallurgical coke. Metall. Mater. Trans. B. 45 (2014), pp. 106–112.
- F. Meng, S. Gupta, D. French, P. Koshy, C. Sorrell, and Y. Shen, Characterization of microstructure and strength of coke particles and their dependence on coal properties. Powder Technol. 320 (2017), pp. 249–256.
- F. Wang, J.L. Zhang, Y. Chen, Q.M. Luo, and Y.L. Hao, Current status of comprehensive use and management recommendations of coal tar in China. Appl. Mech. Mater. 768 (2015), pp. 82–88.
- Q. Zhou, H. Hu, Q. Liu, S. Zhu, and R. Zhao, Effect of atmosphere on evolution of sulfur-containing gases during coal pyrolysis. Energy Fuels 19 (2005), pp. 892–897.
- Y. Mochizuki, Y. Ono, and N. Tsubouchi, Evolution profile of gases during coal carbonization and relationship between their amounts and the fluidity or coke strength. Fuel 237 (2019), pp. 735–744.
- L. Luo, W. Yao, J. Liu, H. Zhang, J. Ma, and X. Jiang, The effect of the grinding process on pore structures, functional groups and release characteristic of flash pyrolysis of superfine pulverized coal. Fuel 235 (2019), pp. 1337–1346.
- G.N. Okolo, R.C. Everson, H.W.J.P. Neomagus, M.J. Roberts, and R. Sakurovs, Comparing the porosity and surface areas of coal as measured by gas adsorption, mercury intrusion and SAXS techniques. Fuel 141 (2015), pp. 293–304.
- M.H. Reich, S.P. Russo, I.K. Snook, and H.K. Wagenfeld, The application of SAXS to determine the fractal properties of porous carbon-based materials. J. Colloid Interf. Sci. 135 (1990), pp. 353–362.
- Y. Zhao, L. Peng, S. Liu, B. Cao, Y. Sun, and B. Hou, Pore structure characterization of shales using synchrotron SAXS and NMR cryoporometry. Mar. Petrol. Geol. 102 (2019), pp. 116–125.
- A. Ghazal, M. Gontsarik, J.P. Kutter, J.P. Lafleur, D. Ahmadvand, A. Labrador, S. Salentinig, and A. Yaghmur, Microfluidic platform for the continuous production and characterization of multilamellar vesicles: A synchrotron small-angle X-ray scattering (SAXS) study. J. Phys. Chem. Lett. 8 (2017), pp. 73–79.
- L. Luo, J. Liu, Y. Zhang, H. Zhang, J. Ma, Y. You, and X. Jiang, Application of small angle X-ray scattering in evaluation of pore structure of superfine pulverized coal/char. Fuel 185 (2016), pp. 190–198.
- Z. Li, Z. Wu, G. Mo, X. Xing, and P. Liu, A small-angle x-ray scattering station at Beijing synchrotron radiation facility. Instrum. Sci. Technol. 42 (2014), pp. 128–141.
- A.P. Hammersley, FIT2D: A multi-purpose data reduction, analysis and visualization program. J. Appl. Crystallogr 49 (2016), pp. 646–652.
- Z.-H. Li, A program for SAXS data processing and analysis. Chinese Phys. C. 37 (2013), p. 108002.
- F. Xie, D. Li, Z. Li, Z. Li, G. Mo, and B. Lv, Small-angle X-ray scattering study on the fractal structure of solid products of bituminous coal at different carbonization temperatures. Phil. Mag. Lett. 99 (2019), pp. 95–101.
- B.B. Mandelbrot, The fractal geometry of nature, Freeman, San Francisco, 1982.
- Y. Wang, Z. Li, J. Kong, L. Chang, D. Li, and B. Lv, In-situ SAXS study on fractal of Jincheng anthracite during high-temperature carbonisation. Phil. Mag. Lett. 101 (2021), pp. 320–329.
- Y. Sun, Y. Zhao, X. Wang, L. Peng, and Q. Sun, Synchrotron radiation facility-based quantitative evaluation of pore structure heterogeneity and anisotropy in coal. Petrol. Explor. Dev. 46 (2019), pp. 1195–1205.
- I. Bressler, B.R. Pauw, and A.F. Thunemann, McSAS: Software for the retrieval of model parameter distributions from scattering patterns. J. Appl. Crystallogr. 48 (2015), pp. 962–969.
- J. Cambedouzou, and O. Diat, Quantitative small-angle scattering on mesoporous silica powders: From morphological features to specific surface estimation. J. Appl. Crystallogr. 45 (2012), pp. 662–673.
- Y. Zhao, S. Liu, D. Elsworth, Y. Jiang, and J. Zhu, Pore structure characterization of coal by synchrotron small-angle X-ray scattering and transmission electron Microscopy. Energy Fuels 28 (2014), pp. 3704–3711.
- Z. Wang, K. Li, J. Zhang, X. Zhang, G. Xue, X. Chen, M. Sun, and C. Jiang, Effect of the moisture content of coal blends on coke structure and its apparent gasification kinetics with CO2. ISIJ Int. 60 (2020), pp. 1909–1917.