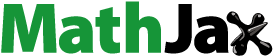
ABSTRACT
A transition of dendrite growth kinetics from stable hcp to metastable fcc phases was observed for refractory Re95W5 alloy in an electrostatic levitation state, which attained a liquid undercooling up to 872 K (0.26TL). Stable hcp dendrite dominated in the single recalescence for pure Re in the whole of its undercooling regime, but prevailed for Re95W5 alloy only in the specific range of 159–867 K undercooling. The rapid dendrite growth velocity displayed a power-law relationship with the degree of undercooling. Once the refractory alloy melt was undercooled beyond 867 K undercooling, metastable fcc dendrite grew primarily from the liquid phase in the first recalescence, which was followed by a complete ‘fcc to hcp’ solid-state transformation in the second recalescence. An abrupt change of growth kinetics took place to depress the dendrite growth velocity drastically from 41.8 m·s−1 for stable hcp phase at 867 K undercooling to 10.9 m·s−1 for metastable fcc phase at 872 K undercooling.
1. Introduction
Rapid dendrite growth within undercooled alloys is of practical importance for its ability to modulate solidification processes and thus assist in the design of materials with better application properties [Citation1–5]. Several intriguing growth phenomena were investigated with the assistance of diverse experimental techniques including drop tube (DT) [Citation6], glass fluxing [Citation7], electromagnetic levitation [Citation8], electrostatic levitation [Citation9], and acoustic levitation [Citation10]. For peritectic alloys, traditional peritectic solidification might be replaced by direct formation of peritectic phase, suppression of peritectic phase, peritectic coupled growth, etc. [Citation11]. Furthermore, anomalous eutectic and dendrite growths could be dominant over lamellar eutectic coupled growth for eutectic alloys [Citation12,Citation13]. In addition, metastable solidification sometimes plays an important role in the rapid crystal growth process [Citation14,Citation15]. Undercooling played a dominant factor in these studies relating to the controlling of dendrite growth modes or metastable phase growth. Thus, it is essentially necessary to undercool liquid metals or alloys in a large undercooling regime to further explore the rapid dendrite growth kinetics in experiments concerning the stable phase dendrite growth kinetics, metastable phase crystallization, microstructural morphologies, crystal orientation relationship, etc.
In this work we selected the Re95W5 alloy (in atomic percentage) by combining these two refractory elements with arc-melting in consideration of their high undercooling abilities. Pure Re and refractory Re95W5 alloy were successfully melted, deeply undercooled and then solidified with the assistance of electrostatic levitation (ESL), in which stable hcp and metastable fcc dendrite growth were observed. In-situ solid/liquid (S/L) interface migration, dendrite growth velocities and microstructural morphology are all examined to investigate the distinctive kinetics transition from stable hcp and metastable fcc dendrite growth.
2. Experimental details
Nearly spherical samples with 2.3 mm diameter were prepared from elemental materials with 99.99% purity. For pure Re, small pieces were cut from the elemental material before being arc-melted into a sample 133.91±0.4 mg in weight. As for Re95W5 alloy, master alloys 5.0 mm in diameter were prepared firstly by arc-melting, then cut into small pieces, and arc-melted again to prepare the ESL samples with a weight of 126.94±0.4 mg. ESL experiment was subsequently conducted for pure Re and refractory Re95W5 alloy. Experimental and testing procedures were carried out as presented in reference [Citation11]. It is noteworthy that spontaneous nucleation and triggered nucleation was adopted for pure Re and only spontaneous nucleation for Re95W5 alloy, and the melting point and liquidus temperature for Re and Re95W5 alloy were calibrated as 3459 and 3405 K according to the binary Re-W phase diagram respectively [Citation16]. In addition, for the quenching experiments, the sample was quenched into the liquid GaInSn alloy by turning off the levitation program around the middle of the two recalescences.
3. Results
The cooling profiles as recorded is presented in c,d. For pure Re, the rapid dendrite growth in recalescence is firstly initiated, followed by slow dendrite growth in the thermal plateau. The thermal plateau duration time versus undercooling relationship generally satisfies the equation
(1)
(1) in which A and B are determined to be 0.66 and −8.03×10−4, respectively. With an increase of undercooling, rapid solidification gradually dominated the solidification process. The thermal plateau duration is close to zero under the maximum undercooling of 820 K, indicating a domination of rapid dendrite growth in recalescence. We are thus confronted with the question: What will happen if one further undercools a liquid alloy beyond this undercooling threshold? Here, we designed the Re95W5 alloy for further exploration. This undercooled melt solidifies in a similar way to pure Re between 159 and 867 K. The parameters of A and B are 0.85 and −9.82×10−4, respectively. On further increasing the undercooling, this crystal growth process with single recalescence transforms into another process, featuring two evident recalescences. The double recalescences can be adjacent or separate to each other with the time interval ranging from 0.09–2.32 s. It is noteworthy that there is a short thermal plateau around 0.09 s just after the first recalescence. Phase structure analyses confirmed that all solidified samples have only a rhenium phase with hcp structure in their final microstructures.
Figure 1. Metastable fcc phase formation initiated by substantial undercooling of liquid Re95W5 refractory alloy. (a) Typical undercoolings shown in Re-W alloy phase diagram [Citation16]. (b) XRD patterns. (c) and (d) Cooling profiles for pure Re and Re95W5 alloy.
![Figure 1. Metastable fcc phase formation initiated by substantial undercooling of liquid Re95W5 refractory alloy. (a) Typical undercoolings shown in Re-W alloy phase diagram [Citation16]. (b) XRD patterns. (c) and (d) Cooling profiles for pure Re and Re95W5 alloy.](/cms/asset/d8b6a8f1-45ec-4624-bbb9-dded8c288bba/tphl_a_2285751_f0001_oc.jpg)
It is apparent that stable hcp dendrite grows during recalescence and the subsequent thermal plateau for pure Re or refractory Re95W5 alloy that undergo a single recalescence. For the alloy which recalescences twice, there is no thermal reaction after the second recalescence. In addition, the final microstructure comprises only a stable hcp phase. It is likely that an unidentified phase is transformed to the hcp phase completely in the second recalescence. Considering this, the unidentified phase must have formed in the first recalescence and subsequent short thermal plateau. The unidentified phase was inferred to have a fcc crystal structure by previous DT experiments and first-principles calculations [Citation17,Citation18]. In fact, there are different metastable phases for Re-W alloy in undercooling experiments, which include bcc [Citation19] and A15 [Citation14]. It is also possible that metastable fcc phase dominated the growth process in the first recalescence. However, it is very difficult to characterise its crystal structure because of its ephemeral nature. Thus, the single recalescing dendrite growth for pure Re and Re95W5 alloy is a stable hcp dendrite growth process, whereas the double-recalescing process for Re95W5 alloy beyond 867 K undercooling is considered to be a metastable fcc dendrite growth process followed by a complete ‘fcc to hcp’ solid-state transformation.
The solid/liquid (S/L) or solid/solid (S/S) interface migration process during recalescence as recorded and processed is illustrated in . Dendrite growth velocities or the solid-state transformation velocities are extracted as V = D/Δt, where D is the diameter of the alloy and Δt is the time for the recalescence process. Firstly, the stable hcp dendrite growth is analysed. For both pure Re and Re95W5 alloy, when the degree of undercooling is relatively small, the solid/liquid (S/L) interface is rough. When the undercooling is very large, smoother S/L interfaces are observed. S/L interface morphologies with six-fold symmetry on the alloy surface are initially observed for undercoolings at 820 K for pure Re and 867 K for Re95W5 alloy and become smoother when advancing forward. The minimum dendrite growth velocities are 2.6 m·s−1 for pure Re at 191 K and 1.4 m·s−1 for Re95W5 alloy at 159 K, whereas the maximum velocities are 38.3 m·s−1 for pure Re at 820 K and 41.8 m·s−1 for Re95W5 alloy at 867 K. A power-law function is adopted to describe the dendrite growth velocities versus undercooling relationship:
(2)
(2) The values of a and b can be found in . The dendrite growth velocities both increase monotonically with undercooling.
Figure 2. A fast videographic study of stable and metastable dendrite growth kinetics for pure Re and refractory Re95W5 alloy. (a) Stable hcp dendrite growth for pure Re. (b) Stable hcp dendrite growth for Re95W5 alloy. (c) Metastable fcc dendrite growth and subsequent ‘fcc to hcp’ solid-state transformation for Re95W5 alloy. (d) Measured dendrite growth velocities of stable hcp phase for pure Re. (e) Measured dendrite growth velocities of stable hcp and metastable fcc phases for Re95W5 alloy.

To further explore the dendrite growth feature for this stable hcp phase, theoretical calculations based on the LKT/BCT dendrite growth model have been carried out [Citation20,Citation21]. The calculation numerically solves two equations, including
(3)
(3)
(4)
(4) where equation (3) is the combination of four partial undercoolings, including curvature undercooling, solutal undercooling, thermal undercooling and kinetic undercooling. R is the dendrite tip radius, Γ is the Gibbs–Thomson coefficient, σ* represents the stability constant, ΔH is the heat of fusion, CPL is the specific heat of the liquid phase, Pt represents the thermal Peclet number, Pc is the solute Peclet number,
is the Ivantsov function of solute Peclet number,
is the actual liquidus slope under non-equilibrium conditions, C0 is the alloy composition, ξt is the solutal stability function, and ξt represents the thermal stability function. kv is the actual solute partition coefficient.
With increase of undercooling, the calculated velocities become gradually larger than the experimental velocities. This may result from that we used a constant kinetic factor of 0.12 in the calculation of kinetic undercooling since ΔTk = V/μ. The same deviation is also observed for Re95W5 alloy. In addition, the kinetic undercooling increases with undercooling and matches the value of thermal undercooling under 867 K undercooling for the Re95W5 alloy. Kinetic undercooling is the largest partial undercooling with further increased undercooling.
However, once undercooled beyond the critical undercooling of 867 K, the dendritic growth kinetics change abruptly. Metastable fcc dendrite growth dominated the first recalescence followed by a solid-state transformation in the second recalescence. The dendrite growth velocity for the metastable fcc phase is 10.9 m·s−1 at 872 K undercooling, which is considerably smaller than its value of 41.8 m·s−1 for the stable hcp phase at 867 K undercooling. For the ‘fcc to hcp’ solid-state transformation, the S/S interface migration velocity depends on its transformation temperature, ranging from 55.2 m·s−1 at 2965 K to its lowest value of around 10.1 m·s−1 at 1941K.
EBSD experiments were conducted to characterise the sample’s microstructural morphology evolution mechanism. For pure Re, an apparent grain refinement is detected with increase of undercooling as shown in . The average grain diameter decreases from 537 μm under 191 K undercooling to 171 μm at 820 K undercooling. An orientation relationship (OR) between neighbouring crystal grains is detected as the rotation around as about 20∼50°. For Re95W5 alloy solidified through stable hcp dendrite growth process, the average grain diameter decreases from 490 μm at 159 K to 51 μm at 867 K. The OR is also observed for 867 K undercooling.
Figure 3. EBSD characterisation for pure Re and refractory Re95W5 alloy solidified through stable hcp dendrite growth. (a∼c) Pure Re. (d∼f) Re95W5 alloy.

Furthermore, the microstructural morphology changes abruptly for metastable fcc dendrite growth. Firstly, the grain size and morphology change is obvious as exhibited in . For the alloy solidified with two adjacent recalescences for 872 K undercooling in a, the average grain diameter is 63 μm comparable to the alloy solidified under 867 K as presented in e, but the largest grain diameter is 484 μm, i.e. considerably larger than 193 μm for the latter. For the sample solidified with two separate recalescences for 869 K undercooling in b, the grain perimeter is very rough and the largest diameter is 184 μm. Secondly, the pole figures (PFs) for smaller grains (0∼50 μm) and larger grains (>50 μm) are compared. They show different distribution features for the sample with two adjacent recalescences but are very similar for the one with two separate recalescences. Thirdly, the misorientation angle distributions for neighbouring pairs and random pairs are also analysed. Three ORs are detected exclusively for the sample solidified with two separate recalescences, including rotations around of about 20∼50°, and around
of about 57∼73° and 80∼93°, respectively. As for the sample with two adjacent recalescences, an OR is not observed.
Figure 4. Microstructural morphology of refractory Re95W5 alloy formed through metastable fcc dendrite growth and subsequent ‘fcc to hcp’ solid-state transformation. (a) Double adjacent recalescences with time interval of 0.09 s at ΔT = 872 K. (b) Two separate recalescences with time interval of 2.32 s at ΔT = 869 K. (c) Quenching around the middle of two separate recalescences with ΔT = 869 K. Label (1) is the Inverse pole image, label (2) and (3) the pole figures, label (4) is the misorientation angles for neighbouring pairs (NP) and random pairs (RP).

4. Discussion
By combining the results above, we can make a detailed analysis on the crystal growth process for Re95W5 alloy solidified with double recalescences. For the sample with two separate recalescences, metastable fcc dendrite first grows from the undercooled alloy during the first rapid recalescence. Then, this dendrite transforms completely to the hcp phase in the second recalescence. The ORs for hcp crystal grains may come partly from the large inner stress within the alloy during the second recalescence. Since the temperature for the second recalescence is very low, slow atomic migration may also contribute to the special ORs as well as the rough perimeters. However, these ORs are not observed for Re95W5 alloy with two adjacent recalescences because the ‘fcc to hcp’ solid-state transformation temperature is higher, which is sufficient to eliminate the effects of inner stress and slow atomic migration.
ESL-aided quenching was adopted for further investigation. The sample was cooled to an undercooling of 869 K before the first recalescence. Then its temperature rose to 2985 K, considerably lower than that of the stable hcp dendrite growth, which ensured it went through the metastable solidification mode. Then the quenching was conducted around the middle of the two recalescences. The solidified alloy shares similarities with the unquenched counterpart, including the rough grain perimeters, near identical PFs for smaller and larger grains and three rotation ORs for neighbouring and random pairs. However, the largest grain diameter is 323 μm, considerably larger than the counterpart but smaller than that of the alloy with two adjacent recalescences, which mean that large inner stress and slow atomic migration still play important roles in the solid-state transformation in this quenching experiment.
The solidification of undercooled liquids usually includes a rapid growth process in the fast recalescence and a slow growth process in the thermal plateau [Citation22]. These two parts cooperate to grow stable crystals. Crystal growth with a single recalescence is usually observed for metals with only one solid phase and another respective liquid phase in an equilibrium phase diagram. With an increase of undercooling, the driving force of the Gibbs free-energy difference between liquid and solid phases becomes stronger, providing a larger driving force for S/L interface migration. Thus, the rapid dendrite growth in fast recalescence gradually dominates the solidification process. Finally, the slow growth process is replaced at the critical undercooling. Previous studies indicated that there was an undercooling limit for undercooled liquids [Citation23]. Here, we might have reached this limit for Re95W5 alloy with its high undercooling ability. Once this value is exceeded, the undercooled liquid may transform into a very unstable state with large viscosity. During this state, the stable hcp phase becomes more difficult to form than the metastable fcc phase. Further decreasing the temperature, the metastable phase needs to transform to a stable phase. Thus, two recalescences are required for the whole metastable dendrite growth process.
Furthermore, because the fcc phase is transitory [Citation18], the second recalescence normally occurs immediately just after the thermal plateau. The outer alternating electric field was changing frequently during sample levitation, which could cause some slight vibrations that may have accidentally caused immediate phase transformation in the second recalsecence. However, the rather quiescent ESL technique, which eliminates many experimental disturbances and sometimes avoided immediate triggering of nucleation and subsequent phase transformation, provided another possibility with two separate recalescences. The sudden velocity drop beyond the critical undercooling comes from the fact that this metastable fcc phase shares different dendrite growth kinetics compared with that of stable hcp phase.
In addition, no trace of the fcc phase is observed for the alloy solidified through metastable fcc dendrite growth process as verified by HRTEM in . The EDS mappings show very homogeneous distribution for rhenium and tungsten elements in the acquired region and the elemental composition is very close to the nominal one. The crystal spacings for the planes of and
and the cell parameters satisfy the following relationship.
(5)
(5)
(6)
(6)
Figure 5. HRTEM characterisation for refractory Re95W5 alloy solidified through metastable fcc dendrite growth at 869 K undercooling. (a) and (b) EDS mappings for rhenium and tungsten elements respectively. (c) and (d) SAED and high-resolution image for hcp phase in the direction of .
![Figure 5. HRTEM characterisation for refractory Re95W5 alloy solidified through metastable fcc dendrite growth at 869 K undercooling. (a) and (b) EDS mappings for rhenium and tungsten elements respectively. (c) and (d) SAED and high-resolution image for hcp phase in the direction of [112¯0].](/cms/asset/0a9cf5e1-dd6c-4dd0-bc90-e2cdec75b53d/tphl_a_2285751_f0005_oc.jpg)
Thus, a and c are determined to be 0.276 and 0.472 nm, respectively.
5. Conclusions
In summary, a distinctive transition of dendrite growth kinetics from stable hcp to metastable fcc phases was observed with the assistance from ESL experiment for pure Re and refractory Re95W5 alloy. The largest achieved undercoolings were 820 K (0.24Tm) and 872 K (0.26TL), respectively. For pure Re, with the increase of undercooling, stable hcp dendrite growth in recalescence gradually dominated the whole solidification process and its velocity increased monotonically forming a grain refined microstructure. The maximum growth velocity attained 38.3 m·s−1. For Re95W5 alloy, when the undercooling increased from 159 to 867 K, the dendrite growth process was similar to pure Re and the maximum velocity was 41.8 m·s−1. However, once exceeding this undercooling threshold, the solidification process was overtaken by the metastable fcc dendrite growth in the first recalescence and followed by a subsequent ‘fcc to hcp’ solid-state transformation in the second recalescence. Meanwhile, the dendrite growth velocity abruptly dropped down to 10.9 m·s−1 at 872 K undercooling.
Disclosure statement
No potential conflict of interest was reported by the author(s).
Additional information
Funding
References
- K. Dragnevski, R.F. Cochrane, and A.M. Mullis, Experimental evidence for dendrite tip splitting in deeply undercooled, ultrahigh purity Cu. Phys. Rev. Lett. 89 (2002), pp. 215502.
- M. Eshraghi, S.D. Felicelli, and B. Jelinek, Three dimensional simulation of solutal dendrite growth using lattice Boltzmann and cellular automaton methods. J. Cryst. Growth 354 (2012), pp. 129–134.
- C.R. Clopet, R.F. Cochrane, and A.M. Mullis, Spasmodic growth during the rapid solidification of undercooled Ag-Cu eutectic melts. Appl. Phys. Lett. 102 (2013), pp. 031906.
- M. Ben Amar, and E. Brener, Theory of pattern selection in three-dimensional nonaxisymmetric dendritic growth. Phys. Rev. Lett. 71 (1993), pp. 589–592.
- D.V. Alexandrov, and P.K. Galenko, Selection criterion of stable dendritic growth at arbitrary Peclet numbers with convection. Phys. Rev. E 87 (2013), pp. 062403.
- S.L. Wei, L.J. Huang, J. Chang, W. Zhai, S.J. Yang, and L. Geng, Primary phase growth and microstructure evolution of rapidly solidifying ternary Ti-12Al-8V alloy. Mater. Lett. 175 (2016), pp. 291–295.
- D. Granata, E. Fischer, V. Wessels, and J.F. Loffler, The detrimental effect of flux-induced boron alloying in Pd-Si-Cu bulk metallic glasses. Appl. Phys. Lett. 106 (2015), pp. 011902.
- J. Chang, H.P. Wang, K. Zhou, and B. Wei, Thermophysical properties and rapid solidification of an undercooled liquid hexabasic Ni-based alloy. Philos. Mag. Lett. 93 (2013), pp. 254–263.
- L. Hu, M.J. Lin, and B. Wei, Hypercooling limit and physical properties of liquid MoNbReTaW refractory high-entropy alloy. Philos. Mag. Lett. 101 (2021), pp. 312–319.
- M.A.B. Andrade, N. Perez, and J.C. Adamowski, Review of progress in acoustic levitation. Braz. J. Phys. 48 (2018), pp. 190–213.
- M.J. Lin, L. Hu, R.L. Xiao, Y.J. Jin, P.X. Yan, and B. Wei, Rapid crystal growth kinetics of tungsten dendrites under electrostatic levitation state. Chem. Phys. Lett. 803 (2022), pp. 139820.
- B. Wei, G. Yang, and Y. Zhou, High undercooling and rapid solidification of Ni-32.5% Sn eutectic alloy. Acta Metall. Mater. 39 (1991), pp. 1249–1258.
- A.M. Mullis, and C.R. Clopet, On the origin of anomalous eutectic growth from undercooled melts: Why re-melting is not a plausible explanation. Acta Mater. 145 (2018), pp. 186–195.
- S. Tournier, B. Vinet, A. Pasturel, I. Ansara, and P.J. Desre, Undercooling-induced metastable A15 phase in the Re-W system from drop-tube processing. Phys. Rev. B 57 (1998), pp. 3340–3344.
- E. Principi, M. Minicucci, A.D. Cicco, A. Trapananti, S.D. Panfilis, and R. Poloni, Metastable phase diagram of Bi probed by single-energy x-ray absorption detection and angular dispersive x-ray diffraction. Phys. Rev. B 74 (2006), pp. 064101.
- Z.K. Liu, and Y.A. Chang, Evaluation of the thermodynamic properties of the Re-Ta and Re-W systems. J. Alloys Compd. 299 (2000), pp. 153–162.
- B. Inet, S. Tournier, and P.J. Desre, Undercooling experiments on the Re-rich part of the Re-W system by processing in a 48 m high drop-tube. Mater. Sci. Forum 179-181 (1995), pp. 723–728.
- L. Cortella, B. Vinet, P.J. Desre, A. Pasturel, A.T. Paxton, and M. Vanschilfgaarde, Evidences of transitory metastable phases in refratory metals solidified from highly undercooled liquids in a drop tube. Phys. Rev. Lett. 70 (1993), pp. 1469–1472.
- S. Tournier, M. Barth, D.M. Herlach, and B. Vinet, Competitive formation of b.c.c.- and σ-phases in undercooled Co-V and Re-W melts. Acta Mater. 45 (1997), pp. 191–199.
- J. Lipton, W. Kurz, and R. Trivedi, Rapid dendrite growth in undercooled alloys. Acta Metall. 35 (1987), pp. 957–964.
- W.J. Boettinger, S.R. Coriell, and R. Trivedi, Rapid solidification processing: principles and technologies IV, Claitor's, Baton Rouge, 1988.
- E.G. Castle, A.M. Mullis, and R.F. Cochrane, Evidence for an extensive, undercooling-mediated transition in growth orientation, and novel dendritic seaweed microstructures in Cu-8.9 wt.% Ni. Acta Mater. 66 (2014), pp. 378–387.
- X.J. Han, C. Yang, B. Wei, M. Chen, and Z.Y. Guo, Rapid solidification of highly undercooled Ni-Cu alloys. Mater. Sci. Eng. A 307 (2001), pp. 35–41.