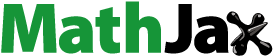
ABSTRACT
Mg-based alloys as revolutionary implantable biomaterials have increasingly attracted considerable attention, owing to their biodegradability in vivo and beneficial effects on biological systems. The degradation process and products of Mg-based alloys have been reported to exhibit significant biological effects on host-tissue responses. However, these effects have not yet been fully understood. This review systemically summarizes and analyses the current understandings and recent research progress in this area. The primary focal points are the biological effects and related mechanisms associated with the degradation behaviour of Mg-based alloys. The biological impacts of the degradation products are elucidated and the arguable or controversial issues are also discussed, providing a pathway toward a greater understanding of the biological implications of Mg-based alloys. Furthermore, based on these biological implications, the restorative potential of Mg-based alloys for applications in tissue repair and regeneration is summarized. Finally, outlooks on biosafety evaluation and design strategies for Mg-based alloy implants are briefly discussed.
Introduction
Biodegradable magnesium (Mg) and Mg-based alloys have been proposed and developed for clinical applications since the late nineteenth century [Citation1]. Owing to limitations such as fast degradation and low mechanical strength, Mg-based medical implants and devices failed to generate satisfactory clinical outcomes for decades and were even considered not appropriate for human applications. Since the late 1990s, advances in fabrication and processing techniques have led to significant improvements in the controls of the biodegradability, biocompatibility, and mechanical properties of Mg, and meanwhile, Mg-based alloys re-emerged as revolutionizing metallic biomaterials [Citation2,Citation3].
In the past two decades, potential applications of Mg-based alloys in various medical fields have been studied, including but not limited to orthopaedic implants, cardiovascular stents, sutures, surgical clips, and staples. Among these, orthopaedic implants and cardiovascular stents received more attention than other applications because Mg-based alloys have appropriate mechanical properties and biodegradability for these applications [Citation4,Citation5]. Recent human orthopaedic and cardiovascular clinical studies have shown the great translational potential of Mg-based alloys, and several commercial products have been produced in these fields. For example, a series of commercial Mg bone screws have been developed or approved for clinical use in China, Germany, and Korea. The clinical use of these bone screws has been extended to hundreds of patients in >50 countries/regions. For cardiovascular application, WE43 (a rare-earth-containing Mg alloy)-based Magmaris® sirolimus-eluting stents produced in Switzerland have reached >2000 clinical implantations in 356 hospitals across 45 countries in Europe, Asia, South America, Africa, and Oceania [Citation6]. Importantly, the clinical needs for orthopaedic and cardiovascular implants are high and increasing rapidly. For example, approximately 2.8 million cases of bone repair are performed annually worldwide [Citation7]. In 2015 coronary artery diseases cause approximately 18 million deaths globally [Citation8]. Thus, the rapidly increasing healthcare demand and market potential have attracted the persistent efforts of materials scientists, biomedical engineers, clinicians, and industry for the development and translation of Mg-based alloys.
Different applications result in varying requirements for material development of Mg-based alloys. For example, relatively high strength is a primary requirement for load-bearing orthopaedic applications, while high ductility and fatigue resistance are more critical for cardiovascular applications in which the implant undergoes significant plastic deformation during implantation, as well as cyclic elastic deformation afterward. Specifically, biodegradable metals for a load-bearing bone implant application are suggested to be comparable to those of pure Ti (ultimate tensile strength: 400∼600 MPa; elongation to failure: 10%∼20% [Citation9]), while biodegradable stent material should have a yield strength >200 MPa, ultimate tensile strength >300 MPa, elongation to failure > 15–18%, and resistance to cyclic fatigue >10–20 million cycles (under physiological-loading conditions) before failure [Citation10].
In addition to mechanical properties, the degradation or corrosion rate of Mg-based alloys is often required to match the level of tissue-healing progress in order to maintain biological and biomechanical stability surrounding the implant. For example, uniform corrosion of Mg-based alloys is usually wanted for predicable degradation rate and mechanical performance, minimizing unexpected implant failure and adverse biological responses due to irregular degradation. This is why the content of impurities in Mg-based alloys (including Cu, Ni, Fe, etc.) should be tightly controlled since they can cause increased and localized corrosion (that corrosion is much faster in some places than in other regions) [Citation11]. Meanwhile, the biological properties associated with the corrosion or biodegradation of an Mg-based alloy is of extremely importance and the biological effects of alloying elements should be carefully examined in alloy design and manufacturing.
To date, Mg-Al based, Mg-rare earth (RE) based, Mg-Zn based, Mg–Ca based, Mg-Sr based, Mg-Li based, Mg-Si based, Mg-Cu based, and Mg-Ag based binary, ternary and quaternary alloys have been proposed for biomedical applications [Citation4,Citation12,Citation13]. Among the alloying elements, Zn, Ca, Sr, Si, and Li within proper concentration ranges are considered non-toxic or possess biological functions that may benefit tissue healing or growth [Citation14–17]. Albeit Cu and Ag are also functional elements (both have antibacterial activity and Cu also has osteogenic/angiogenic capability), their contents in alloys must be strictly controlled to avoid severe toxic effects beyond the concentration threshold. Generally, Al and some RE elements (such as La and Ce [Citation18]) need to be avoided or used with great caution due to their high toxicity.
Current research directions of Mg-based alloys for biomedical applications mainly focus on the exploration of commercially-available Mg-based alloys for clinical uses, development of novel Mg-based alloys or devices, surface modification of Mg-based alloys, and development of evaluation methodologies that meet the regulatory and clinical requirements. Behind these research directions, the understandings of biological implications, and the tissue restorative potential of Mg-based alloys are key foundations and challenges. This is largely because the degradation behaviour and products of Mg-based alloys, in contrast to those of the nondegradable metals, have unique biological effects on the host tissues, and equally important the host tissue responses also significantly affect the degradation behaviour of alloys.
Unfortunately, the biological effects of Mg-based alloy during and after degradation have still yet been thoroughly investigated and understood, especially considering the inconsistent or even contradictory results and notions reported by the existing literature [Citation19–21]. Even for consistently observed biological phenomena associated with Mg-based alloys, few underlying biological mechanisms have been elucidated and widely accepted. For example, although the formation of pores within newly-formed bone has been frequently observed around different Mg-based alloys, the mechanism has not been fully revealed. In fact, this lack of understanding has impeded the rational design and development of Mg-based alloys for wider biomedical applications and has become a major hindrance to the clinical translation and commercialization of Mg-based-alloy medical implants. Even the aforementioned Mg-based alloy products that are ready for market launch demand a better understanding of the biological implications over the products’ lifetime.
This review aims to systemically summarize and analyse the current understandings of the biological effects and implications of Mg-based alloys, attempting to provide a clear and in-depth guide for future studies. In the first part of the review, the degradation behaviour and products of Mg-based alloys in the physiological environment are introduced, and the increasing demand for defining the biocompatibility of biodegradable metals is discussed. Second, the biological implications of Mg-based alloys owing to the dynamic releases of Mg2+ and other degradation products are summarized, focusing on the biological functions and their dose-dependence. Some critical but currently controversial issues (like the origin of pores in new bone) related to the biological effects of Mg-based alloys are also discussed. Third, the tissue restorative potential of Mg-based alloys based on the aforementioned biological implications is described. Finally, an outlook on the translational issues of Mg-based alloys is discussed, including the principal considerations for biocompatibility evaluation and implant design.
Biodegradation of Mg-based alloys
General degradation mechanisms
Mg is electrochemically active with a standard hydrogen potential of −2.7 V (Mg/Mg2+) [Citation22], allowing it to potently react with water in aqueous environments via the following major reactions:
(1)
(1)
(2)
(2)
(3)
(3)
The primary degradation products are Mg2+ ions, OH− ions, and H2 gas. Secondary reactions between the primary degradation products and the biological environment subsequently occur, forming various insoluble deposits such as magnesium hydroxide, magnesium phosphates (Mg2(PO4)(OH)·3H2O) and magnesium carbonates (Mg5(CO3)4(OH)2·5H2O) [Citation23]. The degradation of Mg alloys also releases alloy elements in the form of ions and insoluble deposits. Typically more stable than the α-Mg phase [Citation24], the intermetallic compounds in Mg-based alloys could be released in the form of micro-particles after the degradation of α-Mg matrix. The degradation of Mg-based alloys can also markedly change the peri-implant microenvironment through the consumption of H2O or dissolved oxygen in the host tissue. For example, the degradation of Mg-based alloys can also involve the consumption of dissolved oxygen in the host tissue via an oxygen reduction reaction[Citation25], causing a hypoxic microenvironment that interferes with the cells [Citation26] or affects angiogenesis by upregulating multiple pro-angiogenic pathways [Citation27]. A detailed description of degradation products of Mg-based alloys could be referred to a reference [Citation28].
Electrochemical theory suggests that the degradation rate of Mg-based alloys is determined by both anodic and cathodic reactions. The intrinsic degradation resistance primarily depends on the electrode potential of Mg-based alloy, oxidation layer property, and galvanic-corrosion tendency, which are determined by the physicochemical properties including impurity contents [Citation11] and alloying elements [Citation29–32], microstructure (e.g. grain size and second phase, segregation, and intragranular misorientation) [Citation22,Citation33–39], plastic deformation [Citation40], internal stress [Citation41,Citation42] and so on. Surface treatment or modification, which is common in medical implants [Citation43], can also modulate the degradation rate of the Mg-based alloys by retarding [Citation44] or accelerating [Citation45] degradation reactions.
The in vivo degradation rates of Mg-based alloys, however, depend on both the intrinsic degradation resistance and implantation environment [Citation46]. The effects of the local implantation environment on the degradation rate are so intricate that have not been well understood. This is primarily because in vivo degradation is site-specific and the water content, fluid dynamics, and circulation of body fluids adjacent to the implant site all significantly affect the reaction kinetics of Mg-based alloy during degradation [Citation46–56]. For instance, the degradation rates of a rapidly solidified Mg-Zn-Y-Ce-Zr alloy in adult rabbits were calculated to be 1.65 mm/year in bone, 5.34 mm/year in muscle, and 5.70 mm/year in subcutaneous tissue [Citation48]. The degradation rates of Mg-La-Nd-Zr alloy in rats were 0.28 mm/year for subcutaneous and intramuscular implantation, and 1.12 mm/year for intramedullary implantation [Citation49]. Secondly, the Mg-based alloys prone to degrade faster in acidic environments than alkaline environment owing to the shift of cathodic reaction potential. The microenvironments in vivo have different physiological pH values depending on the anatomical location [Citation57–59] and local host responses, such as an acidic environment induced by severe inflammation or activation of osteoclasts [Citation60,Citation61], or an alkaline environment induced by infection [Citation59,Citation62], indicating that Mg-based alloys can have different degradation rates in vivo. Thirdly, the dynamic change of ionic, molecular, and cellular microenvironments surrounding the Mg-based alloys can foster or destroy the formation of degradation layer and change the kinetics of the degradation reactions [Citation63–69]. Fourthly, mechanical stimulation in load- or shear-bearing tissues (such as blood vessel and fractured bone) also alter the degradation process of Mg-based alloys [Citation70–76]. For example, the degradation rate of pure Mg increased by up to two times under tensile stress [Citation70], and that of AZ31 (Mg-Al-Zn) and AM60B-F (Mg-Al-Mn) alloys increased by one time under fluid shear stress [Citation73,Citation76], compared to the samples without loading.
Degradation products
The direct and primary products from the degradation of Mg-based alloys are Mg2+ ions, OH− ions, and H2 gas (Equation (1)) and they each have different biological effects.
Mg2+ ions: In general, Mg-based alloy degradation creates a Mg2+-enriched microenvironment in peri-implant tissue. It has been reported that excess Mg2+ species diffuse from tissue into blood vessels and are then transported by blood flow, before being excreted into urine via kidney filtration [Citation77]. Mg2+ ions has long been known to regulate many physiological processes such as adenosine triphosphate (ATP) metabolism, muscle contraction and relaxation, neurological functions, and neurotransmitter release, vascular tone, heart rhythm, platelet-activated thrombosis, and bone formation [Citation78]. At the cellular level, a Mg2+-enriched microenvironment has also been reported to affect the functions of stem cells [Citation79], osteoblasts [Citation80–82], osteoclasts [Citation83], endothelial cells [Citation84], and immune cells [Citation85,Citation86]. Mg2+-containing material enhances the binding affinities between the integrin family on the cell surface and their ligand proteins. However, the concentration dependence of this behaviour remains to be determined [Citation87,Citation88].
OH− ions: The release of OH− ions contributes to the formation of an alkaline microenvironment or the increase in the pH value, which affects various cell activities and tissue metabolism processes such as osteoblast and osteoclast activities, tissue oxygenation, vascular smooth-muscle toning, and inflammation response [Citation58,Citation89–94]. OH− ions also contribute to the bacterial inhibitory properties of the Mg-based alloys [Citation95]. Theoretically, the pH value on the surface of pure Mg is >10 in a neutral solution [Citation96], but OH− ions can readily be buffered in a physiological environment. For example, the pH value around pure-Mg screws varies from 7.8 to 8.9 when implanted in rabbit tibia [Citation77].
H2 gas: H2 is a unique degradation product of Mg-based alloys and its biological effect has been increasingly studied in the past few years. H2 is considered nontoxic in breathing gas mixture even at high concentrations of up to 49% vol/vol [Citation97] and its role in cellular function, cell signalling, and the tissue healing process via biochemical and biomechanical (owing to gas accumulation in the tissue) pathways has been gradually uncovered in some recent studies. H2 is a well-known reductive and therapeutic gas [Citation98,Citation99] that can efficiently decrease oxidative stress [Citation100] and inflammation [Citation101]. H2 attenuated lipopolysaccharide (LPS)-induced inflammation by reducing the cytotoxic reactive oxygen species (ROS) that are produced in the inflamed tissues [Citation102]. Notably, it can selectively decrease the number of highly cytotoxic oxidative radicals (such as hydroxyl radical and peroxynitrite) in the diseased cells. Moreover, H2 does not affect the metabolic oxidation–reduction reactions in normal cells or disturbs the physiological ROS that participate in cell signalling [Citation103]. H2 gas (via inhalation with air) can also markedly suppress brain injury by buffering the effects of oxidative stress [Citation103] and H2-rich saline (via intravenous drip infusion) has therapeutic effects on myocardial and renal ischaemia and reperfusion (I/R) injury [Citation101,Citation104,Citation105]. However, solubility of H2 in water is very low, up to only 0.8 mM (1.6 ppm, wt/vol) under atmospheric pressure [Citation97], which means that the therapeutic effects of H2 released from Mg-based alloys on the surrounding tissues and cells remain questionable.
H2 gas accumulation has frequently been found in the tissue surrounding Mg-based implants (e.g. commonly in bone marrow cavity and soft tissues [Citation106,Citation107]). The accumulated H2 trapped in the tissue may induce gas pressure that disturbs tissue regeneration and remodelling processes. For instance, H2 gas cavities in the subcutaneous connective tissue developed a lining structure similar to that of the synovial membrane [Citation108]. Significant gas formation interfering with the cortical bone healing process has also been reported [Citation109]. Gas accumulation in bone marrow cavity may also lead to bone compartment syndrome with bone and marrow ischaemia and necrosis [Citation110]. However, it should be mentioned that the biological effects of cavities in tissue formed H2 accumulation should be size-dependent. Only a gas cavity with a size larger than a critical value might compromise the tissue healing process. Therefore, the diffusion behaviour of H2 and bio-effects of H2 accumulation in different tissues worth further investigation in order to understand the biological implications of the degradation rate of Mg-based alloy.
Other degradation products: In vivo degradation of Mg-based alloys also generates insoluble micro-particles including in situ formed inorganics (such as magnesium phosphate) and intermetallics (which exist in most alloys, such as Mg17Al12 in Mg-Al alloys). These insoluble degradation products have been reported to cause inflammatory responses owing to the reactive oxygen species (ROS) overproduction [Citation111], or interfere with the differentiation process of mesenchymal stem cells (MSCs) and osteo-progenitor cells [Citation112].
Degradation behaviour in specific tissues
Bone
Mg-based alloys exhibit tissue-specific degradation behaviour in vivo. Bone provides a complicated physiological environment where the water content, chemical composition, blood supply, cell type, and mechanical stress vary spatially, leading to an inhomogeneous degradation of the Mg-based alloy implant. These factors are crucial to the degradation behaviours of Mg-based alloys, and thus, should be further understood for the better design of implants. As shown in , after a Mg-based alloy screw is transversely implanted in a long bone, the screw contacts with the cortical bone, trabecular bone, bone marrows, and muscle. Substantial variations in water content, vascular anatomy (related to circulation efficiency), cellular components, matrix diffusion coefficient, and mechanical deformation among these anatomical structures creates a highly heterogeneous environment for the screw and its subsequent degradation behaviour. Referring to Equation (1), the water content is a primary determinant of the implant degradation kinetics and varies substantially in the calcified bone (5 wt-%), muscle (76 wt-%), and bone marrows (∼44 wt-%) [Citation113–115]. Moreover, there are several secondary factors affecting Mg-based alloy degradation in the bone. As a rule of thumb, Mg-based alloys tend to degrade faster in the tissue where more blood vessels exist, because the blood flow affects both the water content and transportation rates of the degradation products in the tissue nearby. In addition, existence and density of osteoclasts that create an acidic microenvironment during bone resorption also affect the degradation rates of Mg-based alloys since they degrade at a faster rate in an acidic microenvironment compared to that in a basic environment [Citation61]. For example, Mg-based alloys degraded at comparatively faster rate in the bone marrow and trabecular bone where osteoclasts are abundant, while slower in the cortical bone containing less osteoclasts [Citation116,Citation117]. Furthermore, bone implants of Mg-based alloys often undertake dynamic loads or stresses in vivo, resulting in varied stress distribution depending on the implantation locations. For example, accelerated degradation was typically observed at the site with high mechanical stress like the portion exposed to the bone-fracture gap [Citation72].
Blood vessel
Mg-based alloys have been studied for cardiovascular stent application. The effects of the biodegradability of Mg-based alloy vascular stent on surrounding tissues include the prevention of chronic inflammatory reactions, progressive neointima development, low risks of thrombosis, and side-branch blockage [Citation118]. The degradation behaviour of Mg-based alloy in blood vessel differs drastically from that in the bone since the stent is immersed in water/blood. As shown in , immediately after implantation in the vessels but before endothelialization on the surface, Mg-based alloy stent is directly exposed to blood flow with persistent shear stress and periodic loading of vascular pulsation. Fluid flow significantly affects the degradation mode (including localized, uniform, pitting and erosion modes) and rate of Mg-based stents, as well as the degradation products and local pH changes [Citation73,Citation76]. After endothelialization and the stent is covered by neointima, the degradation of Mg-based alloy stent changes to a vastly different regime where the interaction between the tissue and stent as well as diffusion of water molecules, hydrophilic solutes, and ions through the vascular tissue and arterial wall become dominant factors [Citation119].
In general, the degradation behaviour of Mg-based alloys in blood vessels is determined by the following mechanisms. First, the biochemical environment of the blood vessel influences the formation of a protective layer on the metal surface. For example, provisional matrix formed by fibrin and activated platelets during the coagulation process, and the adsorption of albumin in the inflammation phase of the healing process of vascular injury may retard the degradation of Mg-based alloys [Citation90]. CO2 in blood vessels (the contents in arterial and venous blood can be up to 4.1% and 5.3%, respectively [Citation120]) can also alter the degradation behaviour of Mg-based alloys by influencing the formation of carbonated film [Citation28]. In addition, acidic or basic environment is important for the formation and destruction of the protective layer on an Mg-based alloy [Citation28]. In vascular systems, blood circulation maintains the microenvironmental pH at an optimal level and this stable condition is unique compared to that in other systems like bone or muscle.
Second, blood flow and vascular pulsation induce a periodic biomechanical environment that can also alter the degradation behaviour of the Mg-based alloy stent. It has been reported that the intensity and direction of fluid shear stress have strong effects on the degradation of Mg-based alloys [Citation73,Citation74,Citation76], primarily by affecting the formation and destruction of the degradation products on the alloy surface. Fluid shear stress at a low level appears to protect the alloy surface from localized corrosion but enhance the rate of uniform corrosion, while high-level shear stress promotes localized corrosion [Citation76]. Also, degradation product detachment and strut fracture occur in the areas facing the flow direction [Citation73]. Specifically in the coronary arteries, the implanted stents can simultaneously experience large variations in the fluid shear stress and tensile stress in multiple directions, so the shear or tensile stress distributions of the stent is not uniform [Citation74]. Meanwhile, the stent is designed to undergo large plastic deformation during implantation and withstand cyclic elastic deformations due to vascular pulsation. Therefore, the ductility and fatigue strength should be considered in stent design. And corrosion fatigue resistance of material is also important to prevent rapid decay of mechanical characteristics. To date, the effects of the biomechanical environment produced by vessel beating, particularly the combined effects of different mechanical conditions, on the degradation of the Mg-based alloy (e.g. WE43 alloy) remains unclear [Citation75].
Third, the specific location where the Mg-based stent is implanted in the blood vessel may also affect the material degradation. The site-dependent degradation of pure-Mg wires (attempt to mimic the stents made of woven wires) has been investigated after implantation in different locations within an artery and results showed increased degradation when the wires were placed in the arterial wall but minimal degradation when they were exposed to blood in the arterial lumen for 3 weeks [Citation121]. Understanding the degradation factors mentioned above can provide the necessary knowledge for expanding the applications of Mg-based implants in cardiovascular field.
Impact of tissue healing progression
Changes in water content, chemical composition, and mechanical conditions of various tissues create varying and complicated microenvironments for the degradation of Mg-based alloy implants. Tissue healing progression also imposes dynamic temporal and spatial variables to the degradation equations, adding more challenges to understand the degradation of Mg-based alloys in vivo.
Generally, bone-fracture healing process includes three stages of inflammation, repair, and remodelling [Citation122]. Each stage involves different cellular responses (e.g. variations in cell type, density, phenotype, activity, and differentiation potential), blood vessel and nerve reconstruction (e.g. changes in type, density, configuration, and functions of vessels or nerves), bone extracellular matrix (ECM) rebuild (e.g. changes in the porosity, composition, molecular/crystal structure and alignment), and stress responses (e.g. the type, magnitude, and direction of stress on the bone implant varies). These complex variations dynamically affect the degradation of Mg-based alloys in different manners. For instance, inflammation may accelerate the degradation due to an acidic environment at this stage [Citation60]. After inflammation, fibrosis tissue formation may kick in and subsequently inhibit the degradation of Mg-based alloys by decreasing blood flow and protecting the corrosion surfaces [Citation70,Citation123]. In the remodelling phase, the activation of osteoclasts may again accelerate the degradation by creating an acidic environment [Citation61].
These understandings, however, are preliminary and based on individual test conditions, so need further investigation. But it is relatively clear that the degradation of Mg-based alloys and tissue host responses affect each other alternately. For example, the generation of degradation products involves chemical reactions on the surface of bone implant, creating a dynamic peri-implant microenvironment with changes in the pH value, Mg2+ ion concentration, and gas cavity in the bone marrow [Citation77]. The subsequent inflammation or an osteolysis response caused by these changes or degradation debris in turn may accelerate the degradation process, as suggested by previous reports [Citation124–126].
The wound-healing process of injured blood vessels is also composed of three overlapping phases of inflammation, granulation, and remodelling [Citation127] which are key to the degradation behaviour of Mg-based alloy stent [Citation128]. For example, in the remodelling phase, the Mg-based alloy stent contacts the vessel ECM, resulting in accelerated degradation [Citation90]. While normal arterial wall ECM is reported to impede the formation of the protective phosphate layer on the alloy surface [Citation121], fibrosis of vascular wall can change the alloy degradation behaviour compared to that in a normal healing process. In addition, the stiffness of vascular wall can be changed by host responses and remodelling process, causing a different mechanical environment for the Mg-based alloy stent. Also, when the degradation progresses, there are changes in the geometry and hemodynamics of the artery, which significantly alter the loading conditions and the stress distribution in the Mg-based alloy stent. All these factors affect further degradation of the stent.
It is worthy to emphasize that the tissue healing effects on the degradation of Mg-based alloy are highly dependent on the alloying elements of the material. First, the alloying element in Mg-based alloys plays a significant role in modulating host tissue responses [Citation17,Citation129–131], which in turn affects the degradation behaviour of the Mg-based alloy as discussed above. For instance, yttrium (∼4 wt-%) and rare earth element (∼3 wt-%) in Mg-based alloy showed strong inhibition of smooth muscle cell (SMC) proliferation but moderate reduction of SMC viability [Citation129]. In contrast, the endothelial cells were viable and showed proliferation in the alloy extracts [Citation129]. Second, the alloying element of Mg-based alloys influences their susceptibility to stress corrosion cracking and resistance to corrosion fatigue [Citation132–134], which largely determines the adaption-ability of implant to biomechanical changes during tissue healing. Third, the alloying elements affect the degradation behaviour of Mg-based alloy, such as the tendency to chemicals-induced local corrosion and the ability to form a protective layer [Citation18,Citation29–32,Citation135,Citation136].
Degradation and biocompatibility of Mg-based alloys
Prior to human applications, medical products require a series of preclinical and clinical evaluations for the assurance of their safety and efficacies. In these evaluations, biocompatibility is one of the core indicators throughout all evaluation processes. Biocompatibility, by one of the accepted definitions, is the ability of a material to serve in a specific biomedical application with an appropriate host response [Citation137]. The concept of biocompatibility is initially formed for non-degradable materials and consists of many convoluted factors that become complicated even confusing in the case of biodegradable materials. The biocompatibility requirements make the materials design of degradable materials different from that of the non-degradable counterparts. For example, some alloying elements allowed to be used in nondegradable metallic biomaterials may not be suitable for the preparation of biodegradable metals, such as Ni, Cr, Mo, and Co, considering their toxic and allergic effects associated with the ion release during material degradation. However, there is a lack of consensus so far on the biocompatibility of Mg-based alloys and its evaluation methods, as the degradation behaviour dynamically alters the host responses to the material, and the conventional criterion and evaluation methodology for the nondegradable materials do not fit to these scenarios.
At the cellular level, the cellular reactions to soluble degradation products (i.e. OH− and Mg2+ ions) are concentration dependent [Citation17,Citation79–82,Citation112,Citation138–141]. Comparative studies directly demonstrate the varied cellular responses (such as the myotube formation of myoblasts, proliferation of fibroblasts, and proliferation and alkaline phosphatase (ALP) activity of osteoblastic cells [Citation35,Citation52,Citation142,Citation143]) to the extracts of Mg-based alloys with different degradation kinetics. At the tissue level, the degradation kinetics and mode of Mg-based alloys have been demonstrated to cause vastly different host tissue responses. For example, it was found in diverse animal bone-defect models that the peri-implant bone response is typically mild with good osseointegration when the Mg-based alloy degrades slowly [Citation53,Citation77,Citation109,Citation144–149]. However, the formation of cavities in bone, which are described as ‘lysis’, ‘shading’, ‘bone resorption’, or ‘bone damage’, frequently occur near the fast-degrading Mg-based alloys, including pure Mg, LAE442 (a Mg-Li��Al-RE alloy), Mg-Gd, Mg-Zn-Ca, and Mg-Zn-Zr alloys [Citation144,Citation145,Citation150–156]. Degradation rate-dependent bone reactions have also been reported in comparative studies [Citation30,Citation109,Citation131,Citation144–146,Citation157–168]. These studies collectively demonstrate that the formation of temporary cavities is a function of corrosion rate, which means that the corrosion rate is too high when cavities can be observed around the implants. A better understanding of the relationship between the host response and degradation behaviours of the Mg-based alloys and a more rational description of the various host responses are the basis for defining the in vivo biocompatibility of the Mg-based alloy. For example, if the aforementioned temporary cavities do not impair the mechanical function of bone, there should be no adverse effects on long-term healing. However, the degradation rate-dependence of tissue responses to Mg-based alloy is hard to elucidate, and also has individual variance. Filling the gap between the in vivo and in vitro degradation behaviours and a better description of the cellular responses to Mg-based alloys (or their degradation products) are important prerequisites to define the biocompatibility of a Mg-based alloy.
Due to the reason mentioned above, there is an urgent need to establish the biocompatibility evaluation methodology for biodegradable materials like Mg-based alloys. Although ISO 10993 sets up a series of methods to evaluate the biocompatibilities of biomaterials that are applicable to the traditional nondegradable metallic biomaterials, some of them are apparently not appropriate for biodegradable metals, as also suggested in previous reports [Citation13,Citation169]. In fact, most of the evaluation methods described in ISO 10993 lack in the consideration of material degradation and the inconsistency in the degradation behaviour between in vitro and in vivo.
Modification of some ISO 10993 articles to evaluate potential health risks of Mg-based alloys have been proposed recently. For example, the modification of the in vitro cytotoxicity testing standard (ISO 10993 part 5) has been recently justified by fully considering the differences in the sensitivities of the cells to Mg2+ ions in vitro and in vivo, and the capability of the in vivo circulation system to dilute the local degradation products [Citation169]. It is recommended a minimum 6 times to a maximum 10 times dilution of the extracts for the in vitro cytotoxicity evaluation of pure Mg as orthopaedic implant based on specifically designed experiments. Several criteria regarding the biocompatibility evaluation of biodegradable metals at the cellular, tissue, and organ levels have also been proposed [Citation13], including IC50 (50% inhibitory concentration) and LD50 (50% lethal dose) data of the metallic ions, inflammatory reactions, and element aggregation in the tissues and organs. Although these modifications are reasonable adjustments to the current biocompatibility evaluation methods, they are still not adequate to complete the jigsaw puzzles of biocompatibility of a specific Mg-based alloy. Therefore, there is still an urgent need to establish the biocompatibility evaluation methods and in vitro/in vivo models specifically for Mg-based alloys based on certain application scenarios (including orthopaedic, cardiovascular, and neural applications).
Recently, biological evaluation of medical devices within a risk management process was suggested in ISO 10993-01-2018, in order to minimize the number and exposure of test animals. Accordingly, in vitro evaluation methods are preferred ‘in situations where these methods yield equally relevant information to that obtained from in vivo models’. Meanwhile, it is suggested that ‘biological testing is usually not necessary, if material characterization (e.g. physical and chemical) demonstrates equivalence to a previously assessed medical device or material with established safety’, and that ‘for device extractables and leachable that have sufficient toxicological data relevant to the expected exposure (quantity, route and frequency), further testing need not be required.’ For Mg-based-alloy-comprising devices, a reliable description or prediction of in vivo degradation behaviour is critical to their biocompatibility evaluation. An effective approach is to develop in vitro degradation experimental models that can resemble application scenarios in vivo and reflect the degradation mechanisms, progression and outcomes. This should provide necessary information for predicting the in vivo degradation behaviour of an Mg-based alloy in comparison to that of a material that has been safely applied in the intended clinical use. This information includes but not limited to degradation rates and modes, release of degradation products and their physicochemical nature (e.g. size distribution and shape of particulate degradation products, etc.). Thus, the establishment of an in vitro evaluation methodological system of degradation behaviour, especially one that closely mimicks the in vivo microenvironment, is of great value [Citation76,Citation170]. In addition to the experimental approaches, bio-physical theories, mathematical models, and mechanical simulation strategies [Citation171–174] of Mg-based alloy degradation are also important supplements to the evaluation methodological system.
Biological implications of Mg-based alloys
The degradation (or corrosion)-related biological responses to Mg-based alloys at both cellular and tissue levels are largely determined by the biological effects of degradation products. Based on the relevant biological effects of degradation products, the main biological processes at cellular or tissue levels that can be affected by Mg-based alloys are summarized in . By taking advantage of these biological effects, there are many clinical opportunities for tissue repair and regeneration. Therefore, an in-depth understanding of such biological implications associated with metal degradation behaviour becomes critical to realizing a ‘biocompatible and biofunctional’ application of Mg-based alloys.
Table 1. Summary of biological processes at cellular and tissue levels that can be mediated by the degradation products of Mg-based alloys.
Implications for bone homeostasis
Modulation of osteoblast and osteoclast activities
Bone continuously undergoes remodelling including reversed activities of bone resorption and bone formation and its homeostasis largely depends on the balance between the activities of the osteoblasts (bone forming cells) and osteoclasts (bone resorbing cells). The bone marrow stromal stem cells (BMSCs) also play an essential role in bone homeostasis since they can potently differentiate into osteoblasts. Mg2+ ions have been found to regulate BMSC functions in a dose-dependent manner. For example, BMSCs cultured in medium with 2.5–10 mM Mg2+ ions showed significantly increased proliferation and enhanced expressions of ALP and integrins α2 and α3 [Citation79]. Cell culture medium containing 10 mM Mg2+ ions also resulted in the up-regulation of collagen type 10A1 and vascular endothelial growth factor (VEGF, critical to vessel formation in bone [Citation236]) expression in the BMSCs and enhanced ECM mineralization [Citation138]. The mRNA expression levels of Collagen I and ALP in the BMSCs cultivated in solutions with 5 and 10 mM Mg2+ ions were significantly higher than those in 0.8 mM solution [Citation139]. Stimulated osteogenic differentiation of the BMSCs has been found to involve magnesium transporter 1 (MagT1)-dependent Mg2+ ion influx [Citation20,Citation183]. However, high Mg2+ ion concentration (5 mM) was also reported to cause disordered differentiation osteoblasts [Citation80]. Besides Mg2+ ions, H2 gas released by Mg degradation was also reported to decrease the ultraviolet radiation-induced BMSC apoptosis by increasing the Bcl-2/Bax ratio, elevating the mitochondrial membrane potential, and blocking cytochrome release [Citation237].
The degradation of a Mg-based alloy generally forms a basic environment surrounding the implantation site. There is evidence suggesting that bone formation can occur in a relatively higher pH environment compared to neutral physiological pH environment (7.35–7.45 for blood and the interstitial fluid) [Citation59,Citation140,Citation218]. ALP activity, collagen gene expression, and collagen synthesis of the BMSCs were significantly increased at pH 7.5 compared to those in the cells cultured at pH 6.6 [Citation219]. The growth and mineralization of human BMSCs at a pH range of 6.3–8.5 were investigated and the optimal activity of BMSCs were found at pH 8.0 [Citation220]. Differentiation of the osteoporotic rat BMSCs was enhanced at pH 7.57∼7.73 compared to that at pH 7.40 [Citation221]. Similarly, collagen synthesis, ALP activity, and thymidine incorporation in human osteoblasts were enhanced when the pH is increased from 7.0 to 7.6 [Citation222]. Moreover, the activity and ALP expression of the osteoblasts improved at pH 8∼8.5 compared to those at pH 7.3 [Citation223,Citation224].
As reported, the Mg2+/OH−-induced activation of the BMSCs and osteoblasts might be via specific signalling pathways, including hypoxia-inducible factor [Citation138], canonical Wnt signalling [Citation238], MAPK/ERK [Citation183], and PI3K/Akt signalling pathways [Citation239], as well as integrin-mediated mechanisms such as modulation of the orientation of adsorbed fibronectin to exhibit enhanced receptor binding affinity and upregulate integrin α5β1 expression of the BMSCs [Citation88].
Osteoclasts are end-differentiated multinucleated cells of monocyte/macrophage lineage and are key players in bone remodelling [Citation240] and periosteal bone formation [Citation241]. Osteoclasts are typically extremely rare cells in the bone with only two to three per cubic micrometer [Citation116]. Osteoclast numbers increase at the sites of active bone turnover such as in the metaphysis of growing bone [Citation116]. When culturing bone marrow cells in a medium containing different concentrations of Mg2+ ions (0.8, 0.4, 0.08, and 0 mM), it was found that osteoclastogenesis increased, while the activity of osteoclasts deceased at low Mg2+ ion concentrations [Citation184]. When less than 25 mM of Mg2+ ions were supplemented in the cell culture medium, low concentrations enhanced while high concentrations inhibited the proliferation and differentiation of the osteoclasts (peaking between 10 and 15 mM) [Citation83]. A recent study showed that high extracellular Mg2+ ion levels (3∼10 mM) promoted vitamin D3-induced osteoclastic differentiation [Citation185]. Insoluble degradation products from Mg-based alloys may also activate osteoclasts [Citation124,Citation125].
The formation of osteoclasts from RAW 264.7 cells (a mouse leukemic monocyte macrophage cell line) was suppressed when the pH was increased from 7.4 to 8.0 [Citation221]. Also, osteoclastic beta-glucuronidase release was suppressed when the osteoclasts were cultured at pH 7.6 compared to those at pH 7.4, which potentially decreased osteoclastic resorption [Citation91]. In addition, H2 gas likely inhibits osteoclast formation as reported by a few studies. For example, 50 vol.% H2 (50 vol. % H2, 20 vol. % O2, 5 vol. % CO2, balance air) decreased proliferation, promoted apoptosis, and inhibited the expression of osteoclast-related proteins of the bone marrow mononuclear cells (BMMCs) in the presence of an osteoclast-inducing medium [Citation235].
Mediation of bone remodelling and quality
At tissue level, bone homeostasis and quality (including porosity and mechanical properties) can be significantly affected by the degradation behaviours of the Mg-based implant. First, a tight control of serum Mg level is beneficial for bone health. Both extremely low or high Mg levels have harmful effects on bone homeostasis [Citation175]. Mg deficiency induces the uncoupling of bone formation and bone resorption, increases osteoclast density at the endosteal bone surface, and decreases the bone mass and osteoblast number [Citation176], trabecular thickness [Citation177], bone mineral content and bone volume (BV) [Citation178], and growth plate width [Citation242]. In contrast, abnormally high serum- and bone-Mg levels also cause mineralization defects and contribute to bone disorders like osteomalacia, renal osteodystrophy and abnormalities [Citation179,Citation180]. Second, Mg-based alloys with proper degradation behaviour can stimulate new bone formation around the implant and the mechanism at cellular level will be discussed later. But numerous studies [Citation21,Citation131,Citation139,Citation157,Citation243–251] have also demonstrated that Mg-based alloys can also simultaneously activate the surrounding osteoclasts, which might lead to bone resorption. Third, Mg-based alloy-induced bone remodelling is also regulated by the immune, vascular, and nervous systems, whose interplays together contribute to bone homeostasis [Citation252–256]. For example, Mg-element-containing biomaterials promoted macrophage polarization to the M2 phase, which supports osteoblast mineralization [Citation86,Citation190,Citation191] and enhances the osteogenic capacity of BMSCs [Citation192]. Mg2+-ion-induced local secretion of neuronal calcitonin gene-related polypeptide-α (CGRP) from the sensory nerve fibres has been shown to improve bone-fracture healing [Citation20]. Meanwhile, degradation products of pure Mg could modulate osteoclastogenesis and platelet-derived growth factor-BB (PDGF-BB) secretion [Citation139,Citation257], which affect bone angiogenesis and H-type vessel formation [Citation254,Citation258,Citation259]. H-type vessels have been recently found to couple with angiogenesis and osteogenesis [Citation260,Citation261]. Angiogenesis is also coupled with the bone modelling and remodelling processes for appropriate bone homeostasis [Citation262]. For example, a Mg5Ca1Zn alloy accelerated bone healing by releasing anabolic metallic ions into the surrounding tissues to promote the angiogenesis-coupled osteogenesis via vascular-mediated pro-osteogenic mechanism [Citation263]. In addition, a Mg-enriched microenvironment (e.g. local Mg2+ ion concentration of 5 mM) has been found to promote neovascularization [Citation84,Citation183].
Dynamic variations in bone remodelling and bone homeostasis unavoidably change the bone quality, which is a generalized description of several concepts such as bone mass, porosity, mineralization content or bone mineral density, and biomechanical properties. However, the effect of Mg-based alloys on the bone quality remains to be determined because of controversial issues regarding the clinical implications of Mg-based alloys. First, although most of the in vivo studies report the increase in overall bone mass around the Mg-based alloys [Citation21,Citation52,Citation148,Citation243,Citation264–267], local osteolysis or bone resorption has also been reported in several Mg-based alloys such as ZEK100, AX30, and MgYREZr alloy [Citation144,Citation150–155,Citation249,Citation268], indicating a possible risk of specific alloys. Balancing bone formation and resorption by the implanted Mg-based alloys is challenging to control the bone mass and quality on demand.
Second, an increase in bone porosity near the bone-implant interface or in the newly-formed bone has been observed in a large number of studies (representative images shown in (a,b), but origin of the increased porosity or cavities has not been comprehensively investigated [Citation21,Citation55,Citation72,Citation77,Citation150,Citation155,Citation243,Citation248,Citation249,Citation269–273]. There is a debate regarding the origin of cavities, which have been speculated to be a result from H2 formation [Citation35,Citation72,Citation77,Citation150,Citation155,Citation243,Citation248,Citation272] or increased bone resorption by osteoclasts [Citation21,Citation81,Citation144,Citation150–154,Citation274,Citation275], but neither has been systematically investigated. The emergence of cavities or pores in newly formed bone tissue with sizes greater than a specific value can compromise the mechanical properties of the host bone and therefore should be better understood, particularly for a rational implant design. Our preliminary animal studies showed that a large number of osteoclasts existed at the cavities in bone tissue around Mg implant ((c)), indicating that the cavities were more likely induced by the activation of osteoclasts.
Figure 3. Representative images showing the formation of porosity in the bone near Mg-based alloy implants. (a) Ex vivo micro-CT 2-D images of bone implant complexes after implantation of LAE442 alloy in NZW rabbit tibia for 12 months [Citation21]. T: tibia; F: fibula; E: endosteal bone formation; C: cavities. (b) Micro-CT images of rat femora implanted with pure Mg or SS rod for 2 weeks [Citation20]. (c) Tartrate-resistant acid phosphatase (TRAP)-stained histological slices of mouse femora cortical bone on the 14th days after implantation with Mg or Ti rods.
![Figure 3. Representative images showing the formation of porosity in the bone near Mg-based alloy implants. (a) Ex vivo micro-CT 2-D images of bone implant complexes after implantation of LAE442 alloy in NZW rabbit tibia for 12 months [Citation21]. T: tibia; F: fibula; E: endosteal bone formation; C: cavities. (b) Micro-CT images of rat femora implanted with pure Mg or SS rod for 2 weeks [Citation20]. (c) Tartrate-resistant acid phosphatase (TRAP)-stained histological slices of mouse femora cortical bone on the 14th days after implantation with Mg or Ti rods.](/cms/asset/add899c8-d81b-427a-a2fd-a5cd6f2b7c79/yimr_a_2079367_f0003_oc.jpg)
Third, although many studies have demonstrated that bone mineralization surrounding the implants could be enhanced after the implantation of Mg-based alloys [Citation19,Citation51,Citation131,Citation139,Citation157,Citation244–246,Citation249,Citation276–282], decreased mineralization has been reported near pure Mg and MgYREZr alloy [Citation267,Citation271]. The mineral apposition rate varies among different Mg-based alloys and appears to be positively correlated to alloy degradation rate [Citation51,Citation249,Citation276]. The varying mineralization rate can be correlated to Mg2+ ion concentration released into the surrounding tissue, since an appropriate amount of Mg2+ ions can promote bone mineralization [Citation138], while a high concentration of Mg2+ ions may inhibit the mineralization process [Citation80–82,Citation179,Citation180]. Furthermore, degradation of Mg-based alloys can affect the alignment and orientation of the nanosized bone mineral platelets, as well as the crystallite structure, crystal size, lattice spacing, and crystalline order of hydroxyapatite in the bone matrix[Citation159,Citation160,Citation283,Citation284].
It is important to point out that Mg-based alloy-induced changes in the bone biomechanical properties remains unclear. At the microscale level, bone stiffness and hardness can be altered by Mg-based alloys [Citation146,Citation159,Citation160]. However, considering the aforementioned Mg-induced bone porosity, the mechanical properties at the microscale could not reflect the overall bone mechanical properties, which remains to be determined [Citation84]. Importantly, the effect of the porosity or cavities on bone mechanics and its dependence on cavity-size should be investigated through experimental and/or simulation approaches in order to determine the critical cavity size for biomechanical robustness. If the temporary cavities do not hamper the mechanical function of the bone, there may be no adverse effects on long-term healing.
Impact on vascular endothelialization and remodelling
Ideally, vascular regeneration and remodelling mediated by a biodegradable stent like Mg-based or PLGA-based stent should afford a complete re-endothelialization, elimination of permanent irritation to the blood vessel and blood flow, positive vessel remodelling, and restoration of vasomotor function to prevent the development of neointimal hyperplasia and restenosis [Citation285]. The increased Mg2+ ion content, pH value and formation of hydrogen gas resulted from the degradation of the Mg-based stent contribute to the dynamic microenvironment surrounding vascular tissue, which is important for promoting healing response and preventing neointimal hyperplasia and restenosis. At the cellular level, fibroblasts, endothelial cells, and SMCs are involved in vessel endothelialization and vascular remodelling. Poor endothelialization and overproliferation of the fibroblasts and SMCs around the stent should be avoided after stent implantation. After the degradation of stent, the endothelialization by the endothelial cells must take precedence over the fibroblast-involved fibrosis tissue formation.
Extracellular pH and Mg2+ ions can regulate the responses of these cells and subsequent vascular endothelialization and remodelling via several mechanisms. First, both extracellular Mg2+ ions and pH can regulate the fibroblast migration rate by affecting the function of integrin (such as α2β1) and E-cadherin [Citation181,Citation182,Citation225]. For example, cell migration rate and DNA synthesis of fibroblasts decreased almost linearly with an increase in pH values between 7.2 and 8.4 [Citation225]. Second, Mg2+ ions play a significant role in regulating the homeostasis and function of endothelial cells. A low Mg2+ ion concentration (e.g. 0.1–0.5 mM) resulted in the senescent features and dysfunction of the endothelial cells, further causing atherosclerosis, inflammation, arterial stiffening, and thrombosis [Citation186–189,Citation286,Citation287]. It was observed that an extracellular Mg2+ ion concentration of approximately 10 mM promoted endothelial cell proliferation and migration, and further upregulated angiogenesis-related gene expression [Citation17]. An additional study reported that Mg2+ ion concentration of 10 mM stimulated endothelial cell proliferation and enhanced the mitogenic response to angiogenic factors and nitric oxide synthesis compared to that of 1 mM [Citation288]. A Mg2+ ion concentration of 1 mM also promoted the spreading of endothelial cells on multiple ECM substrata compared to that of Mg-free medium [Citation289]. Mg2+ ions induced both the chemokinetic and chemotactic migration of endothelial cells peaking at 0.1 and 10 mM [Citation289], respectively, while the viability and proliferation of human-coronary-artery endothelial cells increased at MgCl2 concentrations from 1.6 mM to 25 mM [Citation198]. Mg also plays a key role in preventing calcification in vascular SMCs [Citation199,Citation290–294]. Extracellular alkalization from pH 7.4 to pH 7.8–8.0 induced a rapid and large vasoconstriction of the SMCs [Citation215]. The intracellular pH and nitric oxide concentration of the endothelial cells and vascular SMCs increased at an extracellular pH of 8.5 [Citation216]. The intracellular pH regulates the intracellular Ca of the vascular SMCs and vascular smooth muscle tone [Citation217]. Third, SMC proliferation was found to be restrained by relatively high concentrations of Mg2+ ions [Citation141,Citation198,Citation200]. A possible explanation is that endothelial cells have a higher tolerance to metallic ions compared to that of the SMCs. For example, SMCs were studied to reveal an upper tolerance limit of approximately 20 mM Mg2+ ions, which was significantly lower than that of the endothelial cells (approximately 40 mM) [Citation17,Citation141,Citation295]. Also, the degradation of Mg-based alloys can cause a shift of the contractile vascular SMCs to an inflammatory phenotype. Vascular SMCs became more proliferative and migratory but undergo apoptosis when exposed to the degradation products of pure Mg, while the AZ31 extracts caused less division and more apoptosis of the vascular SMCs, thus decelerating cell movement and growth [Citation201].
Effect on inflammation
Mg2+ ions have also exhibited anti-inflammatory effect [Citation189,Citation193,Citation194,Citation210,Citation296]. Mg2+ ions (including solution extracts of Mg-based alloys [Citation297]) have been reported to decrease pro-inflammatory markers (CCR7, CD86, CD11c, and iNOS) and pro-inflammatory cytokines (TNF-α, IL-6 and IL-1β), and increase anti-inflammatory molecules (CD163 and CD206) of macrophages under non-stimulated or LPS-stimulated conditions [Citation190,Citation195–197,Citation298,Citation299], indicating that Mg2+ ions could switch the macrophage phenotype from M1 to M2. Besides the Mg2+-enriched ionic environment, Mg-based alloys could modulate inflammation reaction by regulating the physiological pH environment, since many cytokines and proteases that are associated with extracellular alkalosis are involved in the activation of inflammatory cells[Citation92,Citation93]. For example, the inflammation-related p38-MAPK signalling pathway was activated at pH 8.5∼9.5 [Citation94]. Furthermore, H2, being a unique degradation product of Mg-based alloys, is considered a therapeutic medical gas with anti-inflammatory effect [Citation102,Citation232,Citation300] and its effectiveness against several inflammatory disorders has been demonstrated in animal models [Citation232–234].
The modulatory effects of Mg-based alloys on tissue inflammation response are important for tissue lesion repair and regeneration [Citation85,Citation301]. For instance, degradation products of pure Mg showed beneficial effects on promoting MSC proliferation and osteogenic differentiation at appropriate concentrations (e.g., Mg2+ ion concentration of approximately 5 mM) [Citation302]. Mg-2Ag and Mg-10Gd extracts (5 mM Mg2+ ions), pure-Mg scaffold, and Mg-doped titanium showed alteration of macrophage polarization to the M2 phase, which supports osteoblast mineralization [Citation86,Citation190,Citation191]. Additionally, Mg2+ doped in calcium phosphate cement affected the crosstalk between immune cells and osteogenesis-related cells that facilitates bone healing [Citation192]. Most recently, the central role of immunomodulation in Mg2+-induced bone regeneration was revealed [Citation303]. During the early inflammation phase, Mg2+ ions lead to the formation of a pro-osteogenic immune microenvironment. In the later remodelling phase, however, the continued exposure of Mg2+ ions not only causes the overactivation of NF-κB signalling in macrophages and increased number of osteoclastic-like cells but also decelerates bone maturation through the suppression of hydroxyapatite precipitation [Citation303]. Besides bone repair, the immunomodulation effect is essential for successful healing of muscle [Citation304–306]. In the past few years, several strategies have been proposed to modulate the inflammatory response to enhance skeletal muscle repair [Citation307,Citation308], particularly through promoting a M2-balanced response [Citation309]. Therefore, the potential of Mg-based alloys in muscle repair is worth being investigated. Most recently, Mg-based alloys were proposed for skeletal muscle repair in a Chinese patent (application number: CN202110860093.5) based on the observed healing effect of Mg2+ ions on skeletal muscle loss.
Microbial inhibitory effect
The microbial inhibitory effect of Mg-based alloys has been revealed recently [Citation310], which is probably related to the release of OH− and Mg2+ ions that have anti-bacterial effects. A microenvironment with a pH > 9.0 inhibited the growth of most bacteria [Citation226] because abundant OH− species continuously react with H+ from the bacterial ECM and decrease the ATP level of the bacteria [Citation227]. Consumption of H+ around the biomaterial surface can effectively restrain or even kill bacteria [Citation311]. Although Mg2+ ions are well-tolerated by bacteria [Citation95], the synergetic effects of alkalinity and Mg2+ ions contribute to a better bacterial killing capability compared to that with only alkalinity [Citation312]. Recently, hydrogen gas has also shown efficacy in antibacterial and antibiofilm applications [Citation231], and a mechanistic study has revealed that the diffusion of active hydrogen into bacteria upregulated bacterial metabolism genes, which encode a high expression of oxidative metabolic enzymes to generate substantial ROS and cause severe DNA damage. Moreover, Mg(OH)2 and MgO nanoparticles, which are the products of Mg-based alloy degradation, have been reported to exhibit antibacterial activities against Escherichia coli, Staphylococcus epidermidis, Pseudomonas aeruginosa, and Streptococcus mutans [Citation313–316]. Mechanistically, nano-MgO exhibits antimicrobial activity through acid–base reaction between the MgO surface and bacterial wall [Citation313,Citation315], while nano-Mg(OH)2 destroys the integrity of the cell walls after adsorption on bacteria [Citation314].
Pure Mg showed antibacterial effects against Escherichia coli, Pseudomonas aeruginosa, and Streptococcus aureus, with efficacies similar to those of fluoroquinolone antibiotics [Citation317]. The therapeutic effect of pure Mg on osteomyelitis caused by Streptococcus aureus was also been described [Citation318]. Similarly, pure Mg was reported to have the capability to prevent methicillin-resistant Streptococcus aureus (MRSA)-associated osteomyelitis [Citation281]. Furthermore, Mg-based alloys containing well-known antibacterial elements (such as Ag, Cu, and Zn) exhibited better antibacterial activities compared to those of pure Mg [Citation319–322]. For example, a Mg-Nd-Zn-Zr alloy (JDBM) deceased a higher number of bacteria in the MC, prevented abscess-lesions, and supported new bone formation compared to the results with pure Mg in the Streptococcus aureus-induced rat femoral osteomyelitis model [Citation323]. A Mg-Cu alloy intramedullary nail (IMN) repaired bone defect caused by infection in a MRSA-induced rabbit osteomyelitis model [Citation324].
Despite encouraging results, inconsistencies in the antibacterial efficacies of Mg-based alloys have been reported between the in vitro and in vivo studies. For example, an AZ91 alloy exhibited no antimicrobial properties in vivo but showed clear effects in vitro [Citation325]. Similar findings have been reported for pure Mg [Citation95,Citation326]. The inconsistencies may be due to the variations in animal experimental protocols, uneven dispersion of bacteria in the surrounding tissue and so on. Meanwhile, the possible lower degradation rate of the Mg-based alloy in vivo compared to that in the in vitro antibacterial test, and buffering capacity of the living tissue can decrease Mg2+ ion concentration and pH environment near the implant, compromising the antibacterial efficacy. Therefore, filling the gap between the in vivo and in vitro antibacterial properties of Mg-based alloys is important for the development and application of anti-infectiousMg-based alloys. Another challenge in this direction is to develop Mg-based alloys simultaneously with antibacterial and pro-regenerative capabilities. Improved materials research, mechanistic understanding of tissue cell and bacterial functions, and effective evaluation methods are basis to resolve the two challenges of Mg-based alloys for simultaneously promoting tissue repair and inhibiting infection.
Impact on nerve regeneration
Mg supplements have been widely used to treat the nervous system injuries. Successful repair of the nervous system requires either the replacement of neurons that have been damaged by injury or the promotion of axon growth to the original targets. Prior studies, including those by our research groups, have shown that Mg-based alloys show good biocompatibility with the dorsal root ganglion sensory neurons (Mg-10Li and ZN20 alloys) [Citation327] and Schwann cells (Mg70Zn26Ca4 metallic glass) [Citation328]. Recently, it has been reported that the addition of Mg2+ ions in the cultures of neonatal murine neural progenitor cells (NPCs) resulted in increased cell survival [Citation202]. Additionally, the increase in Mg2+ ions by adding magnesium sulfate or magnesium chloride to the culture medium also significantly promoted the differentiation of NPCs into postmitotic neurons and suppression of glial cell differentiation [Citation203].
Mg-based alloy wires/filaments coupled with polymeric conduits have been demonstrated to promote the repair of peripheral nervous system injuries in vivo [Citation329–331]. For example, pure-Mg filament that was placed inside the poly (caprolactone) conduits was used to repair the segmental sciatic nerve defects [Citation329]. Numerous regenerating axons were observed after the complete degradation of pure Mg with only a mild inflammation response in the surrounding tissues. Functionally, in comparison to the control, better recovery of the gastrocnemius muscle was observed in the Mg-treated group. Another study also demonstrated that the pure-Mg filaments placed inside hollow nerve conduits supported long distance axonal regeneration [Citation330].
Rats treated with magnesium L-threonate (MgT) revealed the enhancement of synaptic plasticity and memory by increasing the Mg2+ ion level in rat brains [Citation204]. The application of MgT also reduced Aβ-plaque and prevented synapse loss and memory decline in Alzheimer's disease model mice through elevating brain magnesium [Citation205]. In summary, the biological functions of Mg2+ ions in the nervous system have demonstrated the therapeutic potential of Mg-based alloys for the treatment of nervous system diseases [Citation332,Citation333].
Prevention of cartilage degeneration
Degradation products of Mg-based alloys exhibited clear beneficial effects on cartilage regeneration and protection of cartilage from degeneration [Citation193]. High concentrations of Mg2+ ions (10∼20 mM) supported chondrocyte proliferation (peaking at 10 mM), redifferentiation, and glycosaminoglycan (GAG) production (peaking at 20 mM) [Citation206]. An in vivo study also confirmed that an intra-articular injection of magnesium chloride (20 nM in 20 μl saline) protected knee cartilage by inhibiting autophagy formation [Citation334]. Mg2+ ions promoted chondrogenesis of human synovial MSCs (5 mM) in vitro, ex vivo (human osteochondral defects filled with synovial MSCs in 8 ml PBS with 10 mM Mg2+ ions) and in vivo (rabbit knee osteochondral defects filled with synovial MSC suspension in 30 ml of PBS with 5 mM Mg2+ ions) synthesis of cartilage matrix by the possible mechanism of enhanced adherence of synovial MSCs through integrins [Citation335]. Importantly, Mg microspheres with fine-tuned degradation rate (Mg2+ ion of solution extracts reached 10 mM at day 3) showed the potential to facilitate the formation of layered structures of articular cartilage [Citation207].
H2 and Mg2+ ions also exhibit anti-inflammatory and anti-oxidative effects that can potentially mitigate tissue inflammation and prevent cartilage destruction [Citation193,Citation232,Citation234]. Mg2+ ions (2.2 mM) enhanced the chondrogenic differentiation of mesenchymal stem cells by inhibiting activated macrophage-induced inflammation [Citation197]. In addition to the aforementioned anti-inflammatory effects, Mg2+ ions can inhibit Ca2+-stimulated mitochondrial ROS generation and permeability transition (nonspecific inner mitochondrial membrane permeabilization) [Citation210]. H2 can also efficiently reduce oxidative stress [Citation100] and restore cell death and transcriptional alterations induced by the selective removal of ONOO- derived from NO• in chondrocytes [Citation234]. Notably, Mg-based alloys may potentially prevent osteoarthritis (OA) by mitigating tissue inflammation. For example, poly(lactic-co-glycolic acid) microparticles containing pure-Mg powder, which were intra-muscularly injected to the OA knee as an in situ depot for continuously evolving H2 gas, could effectively mitigate tissue inflammation and prevent cartilage from destruction, thereby arresting the progression of OA [Citation336].
Furthermore, the antagonism effect of Mg2+ ions and pH on Ca2+ ions involves many physiological processes including blood coagulation [Citation337], inflammation response [Citation338–341], collagen accumulation [Citation342], muscle contraction [Citation343], probably through competing for the same binding sites on protein molecules [Citation211,Citation344] or regulating the intracellular Ca2+ concentration and binding to the internal anionic sites [Citation345,Citation346]. Recent studies suggest that the pathological calcification of articular cartilage plays a critical role in OA pathology as a disease initiator of OA progression [Citation347,Citation348]. Therefore, it is highly possible that high extracellular Mg2+ ion concentration and high pH resulted from Mg-based alloy degradation are expected to have an antagonistic effect against Ca2+, which may be beneficial in treating OA induced by the pathological calcification of articular cartilage. Specifically, an imbalance in Ca homeostasis is the underlying cause of calcification in OA, and calcium phosphate crystal deposition is a hallmark of OA, and directly contributes to joint degeneration [Citation349]. The inhibition of pathological crystallite deposition within the joint tissues therefore represents a potential therapeutic target in the management of OA. Mineralization in the matrix vesicles and mitochondria of chondrocytes stems from single mitochondrial granules generated by the combination of Ca and phosphorus in the mitochondria [Citation350]. Mg2+ ion as an antagonist of Ca2+ ion can inhibit spontaneous ATP release as well as the frequency and amplitude of Ca oscillations, to ultimately inhibit mineralization [Citation212]. Meanwhile, the cytosolic Mg2+ ions may attenuate mitochondrial Ca2+ ion uptake [Citation351], which can also possibly prevent pathological crystallite deposition.
Antagonizing effect against calcium-induced cell death
Ca2+ ions are a powerful ‘death trigger’ to cells that cellular Ca2+ overload, or perturbation of intracellular Ca2+ compartmentalization, can cause cytotoxicity and trigger either apoptotic or necrotic cell death [Citation352]. In contrast, Mg is anti-apoptotic in mitochondrial permeability transition and antagonizes Ca-overload-triggered apoptosis [Citation210,Citation211,Citation213]. For instance, Mg2+ ions can inhibit Ca2+-stimulated mitochondrial ROS generation and permeability transition [Citation210]. This potential antagonizing effect against Ca-induced cell death is of considerable values for tissue repair.
For instance, a traumatic injury to skeletal muscle leads to the rupture of sarcolemma, which results in Ca influx, thereby triggering the activation of Ca-dependent proteases and initiation of myofiber necrosis [Citation353,Citation354]. Owing to the antagonizing effect against Ca, a high Mg2+ ion concentration and high pH of the microenvironment surrounding the degraded Mg-based alloy are expected to alleviate Ca-induced myofiber necrosis. This is supported by a recent finding that Mg depletion caused structural damage to the muscle cells due to disrupted Ca homeostasis and oxidative stress [Citation214]. In turn, free-radical-induced membrane damage that results in Ca overload may be the origin of skeletal muscle lesions that occur when Mg is deficient [Citation355]. A recent Chinese patent (application number: CN202110860093.5) proposed to use Mg2+ ions for the treatment of Ca-induced skeletal muscle necrosis.
Similarly for cartilage, the overstimulation of N-methyl-d-aspartate (NMDA) receptors results in a high Ca2+ influx leading to Ca overload, which is an important cause of mitochondrial ROS generation, decrease in ATP, and shortage of cellular energy [Citation356,Citation357]. Ca2+ overload also causes cytomembrane injury by hydrolyzing phospholipids and subsequent cell death. The inhibition of NMDA receptor by Mg2+ ions sequentially decreased the Ca2+ influx, leading to the prevention of chondrocyte damage in OA [Citation208,Citation209].
Anti-tumour activity
Mg2+ ion is a beneficial supplement for the prevention and treatment of osteosarcoma [Citation358], and an increase in the local pH value has been found to damage cytoskeletal F-actin of the bone cancer cells [Citation359,Citation360]. Recently, the tumour inhibitory effect of H2 has been revealed [Citation228,Citation229,Citation361], which could be correlated to the effect of hydrogen on intratumoural ROS levels owing to its anti-oxidation property[Citation362,Citation363]. For instance, H2 scavenged free radicals in bone cancer cells with an efficacy proportional to the rate of H2 release [Citation230].
Recent studies have confirmed the antitumour effects of Mg-based alloys on cancer cells, demonstrating their potential use in clinical oncology as biodegradable implants with antitumour activities [Citation230,Citation364–367]. In a mice transplantable tumour model [Citation364], surface-treated pure Mg inhibited tumour growth, and the anti-tumour property was associated with the H2 release rate. Pure-Mg wires significantly inhibited the growth of SKOV3 cells (human ovarian cancer cell line) in an ovarian tumour model created by the subcutaneous injection of SKOV3 cells in nude mice, indicating their potential for the treatment of ovarian tumours [Citation366]. In vitro tests of this study showed that the inhibitory effect could be due to the degradation products such as Mg2+ ions and H2, which induced SKOV3 cell apoptosis. In another study, pure Mg and Mg–1Ag–1Y alloy both exhibited inhibitory effects against local tumour growth and pulmonary metastasis in nude mice (BALB/c), probably due to the changes in the extracellular acidosis microenvironment, increase in Mg concentration, suppression of C-X-C chemokine receptor type 4 (CXCR4) levels, and increase in prostacyclin (PGI2) synthesis [Citation368]. A WE43 alloy (Mg-3.56%Y-2.20%Nd-0.47%Zr, composition provided in weight percent) also showed inhibitory activity against tumour cells, indicating that the WE43 alloy could be a promising candidate with local antitumour activity for application in orthopaedic implants in clinical oncology [Citation365]. Several studies have revealed that the alloy composition, surface treatment, and degradation rate of Mg-based alloys can affect their antitumour activities [Citation230,Citation368–372], but the efficacy and mechanism of antitumour activity and their correlation to these material properties remain unclear.
Restorative opportunities and applications of Mg-based alloys
Orthopaedic implants and fixation devices
Mg-based alloys have an elastic modulus (∼45 GPa) that more comparable to that of bone tissues than do nondegradable bio-metals, and simultaneously have a relatively high ultimate tensile strength (100∼400 MPa [Citation13]), which enables their applications in biodegradable orthopaedic implants [Citation154,Citation373,Citation374]. Recent studies have reported that Mg-based alloys can accelerate bone-fracture healing [Citation19,Citation72,Citation239,Citation277,Citation375–379]. For example, pure-Mg plates and screws induced peri-implant bone formation with mature osteocytes and active osteoid at the ulna fracture gap ((a)) [Citation375,Citation376]. Pure-Mg screws increased bone growth and bone mineral density at the femoral intracondylar fracture gap compared to those observed with the poly L-lactic acid (PLLA) control ((b)) [Citation72,Citation277]. WE43 plate and screw systems also promoted fracture healing in a porcine craniomaxillofacial osteotomy fixation model [Citation377]. A SrHPO4-coated Mg-Nd-Zn-Zr alloy IMN induced substantial bone formation in the femoral fracture sites ((c)) [Citation239]. Mg-Nd-Zn-Zr IMN solely enhanced osteoporotic fracture (OPF) repair by promoting callus formation in comparison to that of stainless steel (SS) IMN [Citation251]. A Mg2Ag IMN augmented callus formation with accelerated mineralization at the beginning of femur fracture healing and enhanced cartilage turnover at the end of the remodelling phase [Citation19].
Figure 4. Examples of Mg-alloy-based bone-fixation implants. (a) Mg plate and screws for fractured ulna fixation. μCT and toluidine-blue staining of cortical bone after 8 and 16 weeks [Citation376]. (b) Pure-Mg and PLLA screws for femoral intracondylar fracture fixation. μCT scanning of rabbit femur with screws after 4 weeks (red arrows: fracture gap) [Citation277]; (c) SrHPO4-coated JDBM (a Mg–Nd–Zn–Zr alloy) IMNs. Undecalcified sections with Von Gieson staining and μCT 3D reconstruction of rat femurs with IMNs after 8 weeks (red boxes: fracture gap region) [Citation239]; (d) Pure-Mg screw for goat femoral neck fracture fixation, and preoperative and 48-week postoperative μCT 3D reconstruction [Citation380]; (e) Ti/Mg hybrid plate screw system for the fractured tibia fixation (red or blue arrows: Mg screw with PLA coating) [Citation278]; (f) SS/Mg hybrid IMN made by inserting a Mg rod into a hollow stainless steel needle with drilled holes [Citation20].
![Figure 4. Examples of Mg-alloy-based bone-fixation implants. (a) Mg plate and screws for fractured ulna fixation. μCT and toluidine-blue staining of cortical bone after 8 and 16 weeks [Citation376]. (b) Pure-Mg and PLLA screws for femoral intracondylar fracture fixation. μCT scanning of rabbit femur with screws after 4 weeks (red arrows: fracture gap) [Citation277]; (c) SrHPO4-coated JDBM (a Mg–Nd–Zn–Zr alloy) IMNs. Undecalcified sections with Von Gieson staining and μCT 3D reconstruction of rat femurs with IMNs after 8 weeks (red boxes: fracture gap region) [Citation239]; (d) Pure-Mg screw for goat femoral neck fracture fixation, and preoperative and 48-week postoperative μCT 3D reconstruction [Citation380]; (e) Ti/Mg hybrid plate screw system for the fractured tibia fixation (red or blue arrows: Mg screw with PLA coating) [Citation278]; (f) SS/Mg hybrid IMN made by inserting a Mg rod into a hollow stainless steel needle with drilled holes [Citation20].](/cms/asset/f45c5713-657b-43c3-a48d-f1d7d3ff6b23/yimr_a_2079367_f0004_oc.jpg)
Mg-based load-bearing fixation devices showed acceptable mechanical stability for applications such as bone-fracture fixation and osteosynthesis [Citation380–382]. A standard-sized Mg-Zn-Ca alloy plate screw afforded considerable stability for fixating facial bone fractures of beagle dogs in the early bone-healing process [Citation381]. Similarly, the mechanical strength of an extruded WE43 alloy plate screw was sufficient for repairing LeFort I osteotomy in beagle dogs [Citation382]. Large pure-Mg screws had sufficient mechanical strength for repairing femoral neck fractures in goats ((d)) [Citation380]. Despite these results, the mechanical strength of Mg-based alloys remains a major concern in load-bearing applications as degradation proceeds, especially when Mg-based alloys degrade quickly [Citation272]. One solution to this problem is to develop Mg-based hybrid implants with partial or decelerated degradability and accelerated bone-healing ability, such as a Mg-Ti plate-screw system ((e)) [Citation278] and a Mg-SS IMN ((f)) [Citation20]. For instance, a Mg-SS IMN accelerated the bone-healing process of rat OPF in comparison to that with SS IMN by increasing callus- and endochondral-tissue formation [Citation20]. Most recently, a Mg-containing IMN has demonstrated the ability to rescue bisphosphonates-impaired fracture healing via elevating CGRP synthesis and release [Citation383]. Fast osseointegration is critical to the success of bone-fixation devices by establishing bone/implant interfacial mechanical stability. Surface coating is an effective strategy to improve the osseointegration ability of Mg-based alloys [Citation154,Citation273]. In addition, porous screws afford improved osteointegration due to enhanced mineralization and vascularization [Citation384].
Mg-based alloys have also shown promising results in anterior cruciate ligament reconstruction [Citation139,Citation250,Citation279,Citation385–388]. For example, as-extruded pure-Mg hollow interference screws increased the levels of secreted PDGF-BB in the bone marrow, the formation of osteoid at the tendon graft/bone interface, bone mineralization and the hardness of the interface, and improved the migration of BMSCs towards the peri-implant tissues [Citation139]. The authors also revealed that Mg-6Zn-0.5Sr screws enhanced bone formation in the peri-tunnel region and the mechanical stability of the femur-tendon graft-tibia complex, as well as the preservation of peri-tunnel bone mass compared to those observed with poly-lactic acid (PLA) screws [Citation250]. Similarly, in the rabbit anterior cruciate ligament reconstruction model, it was revealed that the tendon-bone healing, the relative area of fibrocartilage at the interface, and the fibrocartilage transition zones in the pure Mg group were superior to those of the Ti group [Citation385].
Nevertheless, it is important to mention that bone union was inhibited by WE43 alloys compared to those with the Ti controls in some animal studies [Citation154,Citation273]. Thus, the detailed mechanisms of Mg-based alloy-mediated bone-fracture healing, including endochondral and intramembranous ossification, should be further investigated. For example, the interaction of Mg-based alloys with periosteal stem cells should be further explored, since it is essential for the repair of atrophic nonunion fractures that are caused by severe damage to periosteal mesenchymal progenitors [Citation389,Citation390].
Fusion cages and bone cement are widely used in spinal surgery for intervertebral and vertebral augmentation. The potential of Mg-based alloy cages for intervertebral fusion has recently been explored. However, the feasibility of Mg-based alloys in spinal fusion remains unclear due to negative results in several preliminary studies. For example, a hybrid cage with AZ31 alloy skeleton which was covered and infiltrated by poly-ϵ-caprolactone (PCL) ((a)) showed no signs of fusion, but progressive encapsulation over time in a sheep cervical spine interbody fusion model [Citation391]. The authors speculated that the PCL prevented excessively fast degradation of the AZ31 alloy, and hindered bony growth through or around the cage. Similarly, spine fusion was not achieved when using a Mg–Zn alloy ((b) [Citation392]) or AZ31 alloy cages ((c) [Citation393]). The authors suggested that intervertebral excessive-Mg accumulation could be responsible for the failure of interbody fusion [Citation393]. Because of these unsatisfactory results, more rational design of Mg-based cages to ensure suitable biological functions and mechanical stability becomes critical. Mg-based alloys have also been used in the fabrication of novel bone cement. To avoid fast degradation and excessive Mg accumulation, surface-degradable bone cement (SdBC) was developed for spinal surgery by incorporating pure-Mg particles into clinically-used poly(-methylmethacrylate) (PMMA) bone cement [Citation394,Citation395]. The SdBCs maintain mechanical robustness of the PMMA bone cement, while possessing enhanced osseointegrative, angiogenic, and anti-infection properties that are lacking in the PMMA cements. Animal studies reveal this new bone cement may improve the treatment outcomes of various orthopaedic surgeries from kyphoplasty to joint arthroplasty.
Figure 5. Spinal cage made of Mg-based alloys. (a) A hybrid cage consisting of the AZ31 alloy and PCL [Citation391]. Left: AZ31 alloy skeleton of the cage, Right: Cage after infiltration and covering with the PCL; (b) A micro-arc oxidation (MAO)-coated Mg–Zn alloy cage [Citation392]; (c) An MAO-coated AZ31 alloy cage [Citation393].
![Figure 5. Spinal cage made of Mg-based alloys. (a) A hybrid cage consisting of the AZ31 alloy and PCL [Citation391]. Left: AZ31 alloy skeleton of the cage, Right: Cage after infiltration and covering with the PCL; (b) A micro-arc oxidation (MAO)-coated Mg–Zn alloy cage [Citation392]; (c) An MAO-coated AZ31 alloy cage [Citation393].](/cms/asset/a9936794-899f-4813-95eb-710d89172454/yimr_a_2079367_f0005_oc.jpg)
Although there are concerns about the efficacy and safety of Mg-based alloys, clinical studies have been conducted to validate the feasibility of Mg-based alloy fixation devices in the treatment of bone deformities and fractures, including mild hallux valgus deformities [Citation268,Citation396–398], distal radius fractures [Citation156], lateral malleolus fractures [Citation399], medial malleolar fractures [Citation400], tibial spine avulsion fractures [Citation401], osteochondral lesions of the talus [Citation402], and mandibular condyle fractures [Citation403]. The feasibility of pure-Mg screw-fixed bone flap transplantation for the treatment of femoral head osteonecrosis was also investigated [Citation280,Citation404]. Notably, MgYReZr screws (Syntellix AG, Germany) were approved with the Communauté Européenne (CE) mark in 2013. MgCaZn screws (U & I company, Korea) were approved for clinical use by the Korea Ministry of Food and Drug Safety in April 2015. Pure-Mg screws (Eontec, China) were officially approved for multicenter clinical trials for the treatment of steroid-induced osteonecrosis by the China National Medical Products Administration in July 2019 and were approved with the CE mark in May 2020.
Cardiovascular stent
The limitations of permanent metallic cardiovascular stents include long-term endothelial dysfunction, delayed re-endothelialization, thrombogenicity, permanent physical irritation, and chronic inflammatory local reactions [Citation405]. Besides, the mismatch in the mechanical behaviours of the stented and non-stented vessel areas, inability to adapt to growth in young patients, and non-permissive characteristics for later surgical revascularization are also potential problems [Citation405]. In 2000, the possibility of using Mg-based alloys for cardiovascular stents was investigated for the first time [Citation2,Citation128], revealing that neointima formation was decreased significantly when the stent started to degrade. However, complete degradation of the stent occurred after 89 days, which is much faster than expected. After implantation into the coronary artery of minipigs [Citation406], the WE43-alloy-based ‘Lekton Magic Coronary Stent’ resulted in a larger minimum luminal diameter (MLD) compared to that observed for the 316 L SS stent-treated group within 12 weeks, indicating less neointima formation and positive remodelling [Citation406]. Recently, a comparative study demonstrated that use of a WE43 wire resulted in a minimal inflammation response in the arterial wall until its complete degradation, while both the Zn- and SS-treated groups experienced similar and persistent inflammation responses which induced the formation of fibrotic capsules and necrosis [Citation141]. Despite these positive results, the long-term degradation mechanism of Mg-based stents in arterial walls is still unclear and remains a major concern in terms of their biosafety. The degradation mechanism of a bare Mg–Nd–Zn–Zr stent prototype in the common carotid artery of New Zealand white (NZW) rabbits was studied during a 20-month implantation ((a)) [Citation407]. The stent had good safety and efficacy with a complete re-endothelialization within 28 days. The stent struts were mostly replaced in situ by degradation products in 4 months, during which the volume and Ca concentration decreased gradually without observation of enrichment of the alloying elements Mg and Zn in any of the main organs [Citation407]. Based on the positive results, the authors developed a shape-optimized Mg–Nd–Zn–Zr alloy stent with a rapamycin-eluting polymer coating [Citation408], and evaluated it in the iliac artery of NZW rabbits. The coated stent resulted in neither thrombus nor early restenosis, supported the vessel effectively until degradation after 5 months, and allowed arterial healing [Citation408].
Figure 6. Animal studies and clinical case analysis of Mg-based alloy vascular stents. (a) Haematoxylin-eosin (H&E) staining images and μCT 3D reconstructed images of JDBM (a Mg–Nd–Zn–Zr alloy) stents in rabbit common carotid artery at different time points [Citation407]; (b) Optical coherence tomography 2D images of Magmaris® WE43-alloy-based stent in human coronary artery demonstrated excellent strut apposition with some struts covering the side branch post-implantation; no visible strut remnants exist; and struts initially covering the side branch being resorbed after 12 months [Citation6].
![Figure 6. Animal studies and clinical case analysis of Mg-based alloy vascular stents. (a) Haematoxylin-eosin (H&E) staining images and μCT 3D reconstructed images of JDBM (a Mg–Nd–Zn–Zr alloy) stents in rabbit common carotid artery at different time points [Citation407]; (b) Optical coherence tomography 2D images of Magmaris® WE43-alloy-based stent in human coronary artery demonstrated excellent strut apposition with some struts covering the side branch post-implantation; no visible strut remnants exist; and struts initially covering the side branch being resorbed after 12 months [Citation6].](/cms/asset/a4dc1480-647b-45d3-b3b1-a83bb0c2e9a5/yimr_a_2079367_f0006_oc.jpg)
The first successful implantation of an Mg-based stent in a human was performed in the left pulmonary artery of a preterm baby suffering from a congenital heart disease [Citation409]. A follow-up study showed its complete degradation after 5 months and no in-stent obstruction or neointimal hypertrophy was observed. This clinical trial showed that Mg-based stents may be widely applicable in clinics. Subsequent clinical studies have been performed in both peripheral and coronary vessels using the WE43-alloy-based ‘Lekton Magic Coronary Stent’ [Citation406,Citation410,Citation411] and the stents were completely degraded during the 4-month follow-up, with an overall performance comparable to that of SS stents. However, a short follow-up does not allow any conclusions to be made regarding late, low-incidence events such as thrombosis. Modifications of the stent characteristics with prolonged degradation and drug elution are still required. The BIOSOLVE-I clinical trials confirmed the safety of a drug-eluting WE43 stent (DREAMS), affording promising results [Citation412]. The subsequent BIOSOLVE-II trial showed that the DREAMS 2G stent had comparable target lesion failure and target lesion revascularization rates to those of the degradable polymer-based stents and permanent drug-eluting stents after 6–12 months, which led to CE approval of the WE43-alloy-based Magmaris® sirolimus-eluting stent (Biotronik AG, Bülach, Switzerland) in June 2016 [Citation413]. One particularly encouraging finding of the clinical premarket studies on the WE43-alloy-based stents is that no definite or probable scaffold thrombosis was observed [Citation406,Citation410,Citation411]. In a Magmaris® postmarket program, the intraprocedural data of over 2000 patients was reviewed ((b)) [Citation6]. Generally, the theoretical advantages of Mg-based stents (compared to polymeric stents) in terms of deliverability and conformability have translated into practice with good intraprocedural performance outcomes.
Despite of the promising results of clinical applications of Mg-based stents, there is a consensus that a better understanding of the interaction mechanism of the local vessel tissue and circulation system with the degradable stent is necessary for the further improvement of stent safety and efficacy. In addition to the alteration of mechanical properties of stents during degradation, the overall biomechanical properties of blood vessels also change during tissue remodelling/growth after stent implantation due to the changes in wall thickness, fibre stretching, maximum wall stress, and material properties (stiffness, anisotropy, and viscoelastic characteristics) [Citation414–416]. Biomechanical changes in the stent and blood vessels during stent degradation and vascular remodelling/growth are yet to be understood [Citation416]. This knowledge is essential to determine the optimal supporting stress for different sites and remodelling stages of blood vessels and prevent side effects by mechanical disturbance. The long-term effects of degradation of Mg-based alloys on vessel repair and remodelling is also unclear, although it is well accepted that a better understanding of the long-term degradation mechanism (including loss of biomechanical function and metabolism of the degradation products) is the prerequisite for answering the above questions.
Surgical instruments for hemostasis and anastomosis
Biodegradable Mg-based alloy clips, staples, and rings have been developed for soft-tissue anastomosis such as skin wound closure and hemostasis, which can potentially reduce the risk of postoperative bleeding due to device shift and persistent inflammation, compared to traditional nondegradable counterparts. The secondary surgery to remove the devices from dense tissues after recovery, which may result in secondary injuries and pain to the patient, can also be avoided. On the canine cholecystectomy, the Mg-0.2Zn-0.1Ca hemostasis clip showed a sufficient sealing capability and excellent biocompatibility, and also prevented the formation of artefacts in the CT scan [Citation417]. The Mg–Zn–Ca and Mg–Zn–Ca–Y alloy clips successfully ligated the carotid artery without blood leakage after surgery [Citation56,Citation418]. Histological analysis and biochemical parameters of blood showed no tissue inflammation around the clips. The Mg–Zn–Ca–Y clip degraded completely after eight months. Coated Mg-2.8Zn-0.8Ca clips exhibited good biocompatibility when applied on rabbit uterine tubes for five weeks ((a)) [Citation419,Citation420]. Notably, a pure-Mg clip was recognized as an innovative medical device by CFDA in 2018 (https://www.cmde.org.cn/xwdt/shpgzgg/cxyxgsh/20180308143746124.html).
Figure 7. Animal studies of Mg-based surgical instruments for hemostasis and anastomosis. (a) Mg-Zn-Ca-Y alloy clip occluded the proximal and telecentric stump of rat carotid blood vessels (i), and cuts the blood vessel from the middle of the two hemostatic clips (ii) [Citation420]; (b) Pure-Mg staples for anastomose small intestine (i), and no bleeding and intestinal fluid exudation after anastomosis (ii) [Citation421]; (c) Mg–Zn–Sr intestinal anastomosis ring (i) and surgical procedure of intestinal anastomosis(ii) [Citation422].
![Figure 7. Animal studies of Mg-based surgical instruments for hemostasis and anastomosis. (a) Mg-Zn-Ca-Y alloy clip occluded the proximal and telecentric stump of rat carotid blood vessels (i), and cuts the blood vessel from the middle of the two hemostatic clips (ii) [Citation420]; (b) Pure-Mg staples for anastomose small intestine (i), and no bleeding and intestinal fluid exudation after anastomosis (ii) [Citation421]; (c) Mg–Zn–Sr intestinal anastomosis ring (i) and surgical procedure of intestinal anastomosis(ii) [Citation422].](/cms/asset/3231035e-cf62-446d-9c80-285f6ebc09bd/yimr_a_2079367_f0007_oc.jpg)
Mg-based alloy staples also showed high efficacy and biocompatibility for soft tissue closure. For example, porcine stomach was successfully closed using the pure-Mg staples ((b)) [Citation421,Citation423]. These staples kept good closure of the anastomosis site without leakage and obvious inflammatory response. The staples also exhibited no fracture or severe corrosion cracks during degradation. In the porcine intestinal anastomoses, Mg–Nd–Y alloy staples did not cause any severe complications such as anastomotic leakage, haematoma, adhesion, necrosis, and serious inflammation reactions [Citation424]. Similar findings were obtained with Mg–6Zn staples in intestinal anastomosis [Citation425]. Pure-Mg staples inhibited the inflammatory response in rectal anastomoses through TLR4/NF-κB and VEGF signalling [Citation426], providing a biological basis for prospective applications in soft tissue repair. Additionally, Mg-based alloys are promising candidates for the fabrication of biodegradable intestinal anastomosis rings [Citation422,Citation427]. For example, after being implanted into the intestinal tracts of Bama miniature pigs ((c)) [Citation422], Mg–Zn–Sr alloy anastomosis rings induced only a mild inflammatory response, mediated the healing of intestinal muscular and mucosal layers similar to their healthy counterparts, and did not cause pathological changes to important organs.
The investigation of Mg-based alloys for hemostasis and anastomosis of soft tissues is still at an early stage, and device optimization remains a challenge. Biological effects of Mg-based alloys on soft-tissue healing processes remain to be determined [Citation143,Citation426], and are highly dependent on the alloy composition and degradation nature [Citation143]. Moreover, the degradation behaviour should be optimized to match the requirements of soft tissue repair [Citation419].
Tissue engineering scaffolds
Repair of segmental bone defects greater than critical sizes is a significant challenge in orthopaedics [Citation429]. Porous Mg-based alloys are promising materials for fabricating bone tissue engineering scaffolds [Citation429,Citation430]. The potential of Mg-based alloys scaffolds has been evaluated in large bone defect models. Porous Mg-based alloys assist bone defect repair by facilitating bone and vascular ingrowth. A porous Mg–Nd–Zn–Zr alloy scaffold coated with brushite perfectly repaired large bone defects in rat and rabbit models by effectively stimulating angiogenesis, osteogenesis, and bone remodelling ((a)) [Citation431]. Open-porous pure-Mg scaffolds induced high levels of bone and blood vessel formation, as well as collagen type I and OPN expression in the lateral epicondyle defect of NZW rabbits ((b)) [Citation167]. Porous pure Mg promoted vascularization in the critical-size murine calvarial bone defects [Citation432]. Tubular Mg–Zn–Ca and Mg–Sr alloy scaffolds showed potential in the repair of ulna segmental defects ((c)) [Citation433,Citation434]. Structurally biomimetic Mg-based alloy scaffolds have been evaluated for simultaneous repair of cortical and cancellous bone. A biomimetic porous pure Mg improved the bone formation in a rabbit femoropatellar bone defect model ((d)) [Citation435]. A biomimetic Mg3Zn1Mn alloy implant with an open-cell porous interior and an outer solid wall expedited the healing process in a segmental defect model of the NZW rabbit ((e)) [Citation436].
Figure 8. Mg-based tissue engineering scaffolds for bone defect repair. (a) Digital and scanning electron microscope (SEM) images of Mg/JDBM-MgF2 scaffold without and with brushite (DCPD) coating. μCT images showing the new bone and vascular formation in the defect area after 4 and 8 weeks (red or white line: bone defect area) [Citation431]; (b) SEM and 2D μCT images of Mg scaffolds; 2D μCT images (red arrows: new bone) and alizarin red S and calcein sequential labels of new bone after 16 weeks [Citation167]; (c) MAO-coated tubular Mg–Zn–Ca scaffold for ulna bone defect repair and X-ray observation after 2, 4, 8 and 12 weeks [Citation433]; (d) A biomimetic-porous Mg material coated with HA/(PEI-SiO2) hybrid layer for femoropatellar defect repair, and a μCT image of femoropatellar bone and the porous Mg [Citation435]; (e) A biomimetic-Mg scaffold for humerus defect repair and X-ray images at various periods [Citation436]; (f) μCT and SEM images of porous alginate/gelatin hydrogel with Mg powder. μCT images, sequential fluorescence staining, H&E staining, and OSX/CD31 immunofluorescence staining of bone formation in defects after 3 weeks, and μCT images of 3D vasculature in defects after 2 weeks, in Blank, Mg free (−Mg) and Mg containing (+Mg) hydrogel groups [Citation437].
![Figure 8. Mg-based tissue engineering scaffolds for bone defect repair. (a) Digital and scanning electron microscope (SEM) images of Mg/JDBM-MgF2 scaffold without and with brushite (DCPD) coating. μCT images showing the new bone and vascular formation in the defect area after 4 and 8 weeks (red or white line: bone defect area) [Citation431]; (b) SEM and 2D μCT images of Mg scaffolds; 2D μCT images (red arrows: new bone) and alizarin red S and calcein sequential labels of new bone after 16 weeks [Citation167]; (c) MAO-coated tubular Mg–Zn–Ca scaffold for ulna bone defect repair and X-ray observation after 2, 4, 8 and 12 weeks [Citation433]; (d) A biomimetic-porous Mg material coated with HA/(PEI-SiO2) hybrid layer for femoropatellar defect repair, and a μCT image of femoropatellar bone and the porous Mg [Citation435]; (e) A biomimetic-Mg scaffold for humerus defect repair and X-ray images at various periods [Citation436]; (f) μCT and SEM images of porous alginate/gelatin hydrogel with Mg powder. μCT images, sequential fluorescence staining, H&E staining, and OSX/CD31 immunofluorescence staining of bone formation in defects after 3 weeks, and μCT images of 3D vasculature in defects after 2 weeks, in Blank, Mg free (−Mg) and Mg containing (+Mg) hydrogel groups [Citation437].](/cms/asset/2fc078f0-361f-4390-91f0-fbd74c314142/yimr_a_2079367_f0008_oc.jpg)
Mg-based-alloy-comprising composite scaffolds reveals well-controled release profile of degradation products [Citation438]. Poly (lactide-coglycolide) (PLGA)/tricalcium phosphate (TCP)/pure Mg porous scaffolds induced more newly mineralized bone than PLGA/TCP scaffolds in 15 mm rabbit radii bone defects [Citation439]. Additionally, the PLGA/TCP/pure Mg porous scaffold showed synergistic osteogenic and angiogenic effects, which enhanced new bone formation and strengthened the newly formed bone quality in a rabbit steroid-associated osteonecrosis (SAON) model [Citation84]. The effects could be due to Mg2+-enhanced blood perfusion after SAON [Citation440]. Notably, this novel scaffold was approved for clinical study as an innovative medical device by CFDA in 2018 (https://www.cmde.org.cn/xwdt/shpgzgg/cxyxgsh/20180613133900434.html). Although the mechanism remains to be revealed, Mg-containing materials can induce vascularized bone regeneration [Citation84,Citation183,Citation263,Citation437,Citation438]. For instance, pure-Mg-particle-modified hydrogel scaffolds induced extensive vascularized bone regeneration in femoral-defect repair in rats ((f)) [Citation437].
Owing to their robust mechanical properties and osteogenic capability, Mg-based alloys have been widely used in the engineering of bone tissue. However, the biological implications of Mg-based alloys in inflammation modulation, angiogenesis, microbial inhibition, and Ca antagonism afford potential applications in soft and connective tissue engineering for the treatment of challenging conditions such as diabetic wounds, volumetric muscle loss, and cartilage damage. For example, Mg-Cu-alloy-particle-containing hydrogel demonstrated great potential in the treatment of severe skin wounds by accelerating wound contraction and improving tissue-repair quality [Citation174]. Composite techniques simultaneously benefit the development of a scaffold with soft-tissue-biomimetic structural and biomechanical properties, as well as optimized degradation rate and degradation product release behaviour, to allow effective tissue repair by restoring biological/mechanical homeostasis at the defect and optimizing the tissue regeneration paradigm.
Summary and outlooks
Biosafety evaluation of Mg-based alloys
As discussed in the previous sections, ideal Mg-based implants are expected to degrade at an optimal rate in vivo with appropriate host responses and eventually degrade completely or partially upon fulfilling their mission to assist with tissue healing. Meanwhile, the released degradation products or implant residues should be metabolized or assimilated by human or animal body. The biocompatibility requirements of Mg-based alloys in a specific application include: (1) complete dissolution with the passage of corrosion products or metabolization/assimilation by the cells and/or tissues, and (2) induction of an appropriate host response during degradation period toward fully degradation. Also as mentioned above, the biocompatibility of Mg-based alloys is more complex than that of traditional nondegradable metals. There is evidence supporting the fulfilment of the first requirement, but achieving the ‘appropriate host response’ is a great challenge currently faced by researchers. First, host responses to Mg-based alloys are complicated and intertwined. Second, the host responses during the degradation process of Mg-based alloys change dynamically depending on at least degradation rate, metabolic rate of degradation products, and the concentration-dependent tissue/cellular reaction to the degradation products. These factors all vary with the material composition and structure, and implantation site-specific conditions. Third, the biological mechanisms underlying some host responses specific to Mg-based alloys remain unclear.
Therefore, one of the key issues for re-defining the biocompatibility concept of Mg-based alloys is the identification of the ‘appropriate host response’. Degradation of Mg-based alloys and its host responses exhibit spatial–temporal dynamic changes in vivo. Thus, the biocompatibility evaluation methodology should also be re-considered together with the following dynamic characteristics.
Biological impacts of Mg-based alloy degradation process and products. The progressive degradation of Mg-based alloys causes temporary or permanent changes in the microenvironment of surrounding tissues, causing constantly changing biological effects (either beneficial or adverse). The definition of adverse effects (mainly including pain, mechanical weakness and dysfunction of tissue, bone lysis, and H2 gas accumulation [Citation441]) and their severity levels are critical to the biocompatibility evaluation and clinical translation of Mg-based alloys. However, the definition of adverse side effects varies significantly among scientists. For example, in an animal study, ZEK100 (0.96 wt.% zinc, 0.21 wt. % zirconium, 0.3 wt. % RE, Mg in balance) alloy induced temporary changes in the bone mass and microstructure, but there was no pathological alteration in important organs (liver, spleen and kidney) or serum Mg concentration [Citation21]. The authors suggested excluding ZEK100 from further biomedical investigation because of the severe bone morphological changes. In a clinical study, the MgYREZr alloy-based screw showed signs of radiolucency, screw loosening, osteolysis, and bone resorption or demineralization in 40% of the cases. However, none of patients exhibited obvious clinical symptoms like pain [Citation268]. Therefore, there is an arguable opinion that surgeons, radiologists, and patients should not be concerned about these mild side effects, as they are an inherent part of the Mg degradation process.
The metabolism process and pathways of degradation products. The decomposition, delivery assimilation and excretion mechanisms of degradation products are related to the temporary alteration of element concentration in local tissue and blood serum, as well as the temporary element accumulation and potential pathological alteration in important organs [Citation442,Citation443]. Meanwhile, metabolic mechanism of degradation products is very different from those from oral-administration analogues [Citation443]. The metabolic process and pathway, which determine the biosafety of Mg-based alloys in specific tissues, varies with the implantation site and species [Citation77,Citation407,Citation443] due to the differences in the anatomical and physical properties of tissue (such as H2 permeability [Citation444,Citation445]). Although the metabolic mechanism of implant Mg has received attention, studies rarely reveal the metabolic pathway and efficiency of alloy elements, which are substantially different from those of Mg element [Citation442,Citation443]. Generation of H2 and OH− are unique for Mg-based alloys, for which the metabolism in specific tissues requires more investigation because of the risk to damage physiological system when they overburden the organism’s regulating mechanisms [Citation443].
The long-term effects on tissues/organs during and after the degradation of Mg-based alloys. There are numerous studies showing that major organs have a high tolerance to the degradation products of Mg-based alloys, without permanent Mg2+ ion accumulation, damage or dysfunction [Citation21,Citation77,Citation84,Citation147,Citation272,Citation280,Citation407,Citation446–450], even for the rapidly corroding Mg-based alloys or in a renal failure model [Citation451]. In addition, there are animal and clinical studies reporting the recovery of bones [Citation145,Citation156,Citation401,Citation446] and blood vessels [Citation407] after the complete degradation of several Mg-based alloys. Nevertheless, both short- and long-term studies on animals and humans are required, particularly on rapidly corroding Mg-based alloys [Citation168]. In addition, the biological consequence of accumulated alloying elements of Mg-based alloys in organs require further investigations [Citation145,Citation272,Citation447,Citation452].
Based on current understandings, the ‘appropriate host responses’ refers to at least three aspects. First, the tissue repair process is not inhibited or compromised by the use of Mg-based alloys. Second, the temporal tissue responses during Mg-based alloy degradation do not cause adverse effects with clinical manifestation or significantly increase the risk of tissue/organ damage and implant failure. Third, the tissue/organ can fully recover or return to a homeostatic state after the degradation of Mg-based alloys. There are reports revealing that Mg-based alloys (and their insoluble degradation products) are not completely degraded or absorbed in vivo for up to 1 year [Citation21,Citation77,Citation149,Citation448]. Also, severe inflammation and osteolysis are likely to occur at the later stages of degradation [Citation124–126]. Therefore, it is suggested that the host responses of a Mg-based alloy should be evaluated over the entire life cycle of the degradable medical device if possible, or at least for a reasonably prolonged period after degradation.
Implant design based on the biological implications of Mg-based alloys
The biological implications of Mg-based alloys indicate their rich restorative opportunities for biomedical applications. Thanks to great advances in material fabrication and processing techniques, Mg-based alloys with tailored composition, geometry, mechanical properties, and 3-D structure have been developed. Further understanding of the biological implications of Mg-based alloys is becoming an important guide for more rational designs of Mg-based alloy comprising implants such as hybrid orthopedic fixation systems, neural conduit, and tissue engineering scaffolds.
However, the degradation- and implantation-site-dependent biological responses, multifaceted effects of Mg-based alloys on tissue quality (including volume, chemical composition, micro/nanostructures, and mechanical properties), and limited understanding of underlying mechanisms clearly indicate that it is still challenging to take the advantages of Mg-based alloys for extensive human applications. Obviously, understanding the biological implications of Mg-based alloys in therapeutic contexts is one of the key prerequisites for exploring the potential applications of Mg-based alloys and optimizing the implant design accordingly.
In a rational design of Mg-based implants, the material composition, intrinsic degradation behaviour including degradation products, and physicochemical properties (e.g. volume, morphology, surface chemistry and topography, porosity, residue stress, etc.) should be first considered. The selection criteria of the material parameters mentioned above depend on the characteristics of the implantation site and target applications, such as the cause and severity of tissue lesion, the tissue intrinsic-repair capacity and mechanism, the tolerance and metabolic capacity of local tissue to the degradation products, as well as the local blood circulation and mechanical conditions. The Mg-based alloys can also incorporate with other biodegradable or non-degradable materials (i.e. to form composite or hybrid materials) to achieve suitable mechanical and biological properties or to control the spatio-temporal release profile of degradation products. But new problems may arise from the biological interference between degradation products from different types of materials. For example, a study on hyaluronic acid/polygalacturonic acid hydrogel containing Mg–Ca alloy cannulated screws reported thatthe inappropriate elution kinetics of Ca and HA from the hydrogel may inhibit the positive effect of the alloys on osteoblast differentiation and proliferation [Citation453].
In summary, unique biological implications of Mg-based alloys provide both opportunities and challenges for clinical applications and have attracted significant interest from both material engineers and clinicians. Material development and device optimization based on these biological implications can provide more possibilities for tissue repair and regeneration. Great efforts are required to deepen the understanding of the biological implications of Mg-based alloys. Meanwhile, a more rational biocompatibility evaluation method and criteria based on these biological implications are in urgent need. Advances in these aspects would significantly promote further translation of Mg-based alloys from bench to bed.
Disclosure statement
No potential conflict of interest was reported by the author(s).
Additional information
Funding
References
- Witte F. The history of biodegradable magnesium implants: a review. Acta Biomater. 2010;6(5):1680–1692. doi:10.1016/j.actbio.2010.02.028
- Heublein B, Rohde R, Niemeyer M, et al. Degradation of metallic alloys - A new principle in stent technology? J Am Coll Cardiol. 2000;35(2):14A–15A.
- Staiger MP, Pietak AM, Huadmai J, et al. Magnesium and its alloys as orthopedic biomaterials: a review. Biomaterials. 2006;27(9):1728–1734. doi:10.1016/j.biomaterials.2005.10.003
- Yang Y, He C, Dianyu E, et al. Mg bone implant: features, developments and perspectives. Mater Des. 2020;185:108259. doi:10.1016/j.matdes.2019.108259
- Wang J-L, Xu J-K, Hopkins C, et al. Biodegradable magnesium-based implants in orthopedics – a general review and perspectives. Adv Sci. 2020;7(8):1902443. doi:10.1002/advs.201902443
- Wlodarczak A, Garcia LAI, Karjalainen PP, et al. Magnesium 2000 postmarket evaluation: guideline adherence and intraprocedural performance of a sirolimus-eluting resorbable magnesium scaffold. Cardiovasc Revasc Med. 2019;20(12):1140–1145. doi:10.1016/j.carrev.2019.02.003
- Mailoo VJ, Srinivas V, Turner J, et al. Beware of bone pain with bisphosphonates. Case Reports. 2019;12(3):e225385.
- Roth GA, Johnson CO, Abajobir AA, et al. Global, regional, and national burden of cardiovascular diseases for 10 causes, 1990 to 2015. J Am Coll Cardiol. 2017;70(1):1–25. doi:10.1016/j.jacc.2017.04.052
- ISO 5832-2: 2018 Implants for surgery – metallic materials – Part 2: Unalloyed titanium, 2018. Available from: December 2018. https://www.iso.org/standard/69907.html.
- Bowen PK, Shearier ER, Zhao S, et al. Biodegradable metals for cardiovascular stents: from clinical concerns to recent Zn-alloys. Adv Healthcare Mater. 2016;5(10):1121–1140. doi:10.1002/adhm.201501019
- Hofstetter J, Martinelli E, Pogatscher S, et al. Influence of trace impurities on the in vitro and in vivo degradation of biodegradable Mg-5Zn-0.3Ca alloys. Acta Biomater. 2015;23:347–353. doi:10.1016/j.actbio.2015.05.004
- Li N, Zheng Y. Novel Magnesium Alloys developed for Biomedical application: A review. J Mater Sci Technol. 2013;29(6):489–502. doi:10.1016/j.jmst.2013.02.005
- Liu Y, Zheng Y, Chen X, et al. Fundamental theory of biodegradable metals—definition, criteria, and design. Adv Funct Mater. 2019;29(18):1805402. doi:10.1002/adfm.201805402
- Habibovic P, Barralet JE. Bioinorganics and biomaterials: bone repair. Acta Biomater. 2011;7(8):3013–3026. doi:10.1016/j.actbio.2011.03.027
- Dalisson B, Barralet J. Bioinorganics and wound healing. Adv Healthcare Mater. 2019;8(18):1900764. doi:10.1002/adhm.201900764
- Šalandová M, van Hengel IAJ, Apachitei I, et al. Inorganic Agents for enhanced angiogenesis of orthopedic biomaterials. Adv Healthcare Mater. 2021;10(12):2002254. doi:10.1002/adhm.202002254
- Zhao N, Zhu D. Endothelial responses of magnesium and other alloying elements in magnesium-based stent materials. Metallomics. 2015;7(1):118–128. doi:10.1039/C4MT00244J
- Li H, Wang P, Lin G, et al. The role of rare earth elements in biodegradable metals: A review. Acta Biomater. 2021;129:33–42. doi:10.1016/j.actbio.2021.05.014
- Jahn K, Saito H, Taipaleenmaki H, et al. Intramedullary Mg2Ag nails augment callus formation during fracture healing in mice. Acta Biomater. 2016;36:350–360. doi:10.1016/j.actbio.2016.03.041
- Zhang Y, Xu J, Ruan YC, et al. Implant-derived magnesium induces local neuronal production of CGRP to improve bone-fracture healing in rats. Nat Med. 2016;22:1160–1169. doi:10.1038/nm.4162
- Dziuba D, Meyer-Lindenberg A, Seitz JM, et al. Long-term in vivo degradation behaviour and biocompatibility of the magnesium alloy ZEK100 for use as a biodegradable bone implant. Acta Biomater. 2013;9(10):8548–8560. doi:10.1016/j.actbio.2012.08.028
- Song G, Atrens A. Understanding magnesium corrosion—A framework for improved alloy performance. Adv Eng Mater. 2003;5(12):837–858. doi:10.1002/adem.200310405
- Ma M, Pokharel DB, Dong J, et al. In vivo corrosion behavior of pure magnesium in femur bone of rabbit. J Alloys Compd. 2020;848:156506. doi:10.1016/j.jallcom.2020.156506
- Liu Y, Liu X, Zhang Z, et al. Comparative, real-time in situ monitoring of galvanic corrosion in Mg-Mg2Ca and Mg-MgZn2 couples in Hank’s solution. Corros Sci. 2019;161:108185.
- Silva EL, Lamaka SV, Mei D, et al. The reduction of dissolved oxygen during magnesium corrosion. ChemistryOpen. 2018;7(8):664–668. doi:10.1002/open.201800076
- Xu L, Willumeit-Römer R, Luthringer-Feyerabend B. Hypoxia influences the effects of magnesium degradation products on the interactions between endothelial and mesenchymal stem cells. Acta Biomater. 2020;101:624–636. doi:10.1016/j.actbio.2019.10.018
- Krock BL, Skuli N, Simon MC. Hypoxia-Induced angiogenesis: good and evil. Genes Cancer. 2011;2(12):1117–1133. doi:10.1177/1947601911423654
- Gonzalez J, Hou RQ, Nidadavolu EPS, et al. Magnesium degradation under physiological conditions – best practice. Bioactive Mater. 2018;3(2):174–185. doi:10.1016/j.bioactmat.2018.01.003
- Pan H, Pang K, Cui F, et al. Effect of alloyed Sr on the microstructure and corrosion behavior of biodegradable Mg-Zn-Mn alloy in Hanks’ solution. Corros Sci. 2019;157:420–437. doi:10.1016/j.corsci.2019.06.022
- Cihova M, Martinelli E, Schmutz P, et al. The role of zinc in the biocorrosion behavior of resorbable Mg-Zn-Ca alloys. Acta Biomater. 2019;100:398–414. doi:10.1016/j.actbio.2019.09.021
- Zeng R-C, Qi W-C, Cui H-Z, et al. In vitro corrosion of as-extruded Mg–Ca alloys—The influence of Ca concentration. Corros Sci. 2015;96:23–31. doi:10.1016/j.corsci.2015.03.018
- Ding Y, Wen C, Hodgson P, et al. Effects of alloying elements on the corrosion behavior and biocompatibility of biodegradable magnesium alloys: a review. J Mater Chem B. 2014;2(14):1912–1933. doi:10.1039/C3TB21746A
- Zeng R-C, Sun L, Zheng Y-F, et al. Corrosion and characterisation of dual phase Mg–Li–Ca alloy in Hank’s solution: The influence of microstructural features. Corros Sci. 2014;79:69–82. doi:10.1016/j.corsci.2013.10.028
- Soderlind J, Cihova M, Schaublin R, et al. Towards refining microstructures of biodegradable magnesium alloy WE43 by spark plasma sintering. Acta Biomater. 2019;98:67–80. doi:10.1016/j.actbio.2019.06.045
- Li W, Shen Y, Shen J, et al. In vitro and in vivo studies on pure Mg, Mg–1Ca and Mg–2Sr alloys processed by equal channel angular pressing. Nano Mater Sci. 2020;2:96–108. doi:10.1016/j.nanoms.2020.03.004
- Parfenov EV, Kulyasova OB, Mukaeva VR, et al. Influence of ultra-fine grain structure on corrosion behaviour of biodegradable Mg-1Ca alloy. Corros Sci. 2020;163:108303. doi:10.1016/j.corsci.2019.108303
- Wang W, Wu H, Sun Y, et al. Local intragranular misorientation accelerates corrosion in biodegradable Mg. Acta Biomater. 2020;101:575–585. doi:10.1016/j.actbio.2019.10.036
- Pulido-González N, Torres B, García-Rodríguez S, et al. Mg–1Zn–1Ca alloy for biomedical applications. influence of the secondary phases on the mechanical and corrosion behaviour. J Alloys Compd. 2020;831:154735. doi:10.1016/j.jallcom.2020.154735
- Wang W, Wu H, Zan R, et al. Microstructure controls the corrosion behavior of a lean biodegradable Mg–2Zn alloy. Acta Biomater. 2020;107:349–361. doi:10.1016/j.actbio.2020.02.040
- Chen K, Lu Y, Tang H, et al. Effect of strain on degradation behaviors of WE43, Fe and Zn wires. Acta Biomater. 2020;113:627–645. doi:10.1016/j.actbio.2020.06.028
- Wu S-x, Wang S-r, Wang G-q, et al. Microstructure, mechanical and corrosion properties of magnesium alloy bone plate treated by high-energy shot peening. Trans Nonferrous Metals Soc China. 2019;29(8):1641–1652. doi:10.1016/S1003-6326(19)65071-5
- Hou R, Victoria-Hernandez J, Jiang P, et al. In vitro evaluation of the ZX11 magnesium alloy as potential bone plate: degradability and mechanical integrity. Acta Biomater. 2019;97:608–622. doi:10.1016/j.actbio.2019.07.053
- Wan P, Tan L, Yang K. Surface modification on Biodegradable Magnesium Alloys as orthopedic implant materials to improve the Bio-adaptability: a review. J Mater Sci Technol. 2016;32(9):827–834. doi:10.1016/j.jmst.2016.05.003
- Lin X, Tan L, Zhang Q, et al. The in vitro degradation process and biocompatibility of a ZK60 magnesium alloy with a forsterite-containing micro-arc oxidation coating. Acta Biomater. 2013;9(10):8631–8642. doi:10.1016/j.actbio.2012.12.016
- Yin Z-Z, Huang W, Song X, et al. Self-catalytic degradation of iron-bearing chemical conversion coating on magnesium alloys — influence of Fe content. Front Mater Sci. 2020;14:296–313. doi:10.1007/s11706-020-0512-x
- Witte F, Hort N, Vogt C, et al. Degradable biomaterials based on magnesium corrosion. Curr Opin Solid State Mater Sci. 2008;12(5-6):63–72. doi:10.1016/j.cossms.2009.04.001
- Sato T, Shimizu Y, Odashima K, et al. In vitro and in vivo analysis of the biodegradable behavior of a magnesium alloy for biomedical applications. Dent Mater J. 2019;38(1):11–21. doi:10.4012/dmj.2017-324
- Willbold E, Kalla K, Bartsch I, et al. Biocompatibility of rapidly solidified magnesium alloy RS66 as a temporary biodegradable metal. Acta Biomater. 2013;9(10):8509–8517. doi:10.1016/j.actbio.2013.02.015
- Reifenrath J, Marten AK, Angrisani N, et al. In vitro and in vivo corrosion of the novel magnesium alloy Mg-La-Nd-Zr: influence of the measurement technique and in vivo implant location. Biomed Mater. 2015;10(4):045021. doi:10.1088/1748-6041/10/4/045021
- Miura C, Shimizu Y, Imai Y, et al. In vivo corrosion behaviour of magnesium alloy in association with surrounding tissue response in rats. Biomed Mater. 2016;11(2):025001. doi:10.1088/1748-6041/11/2/025001
- Huehnerschulte TA, Angrisani N, Rittershaus D, et al. In vivo corrosion of two novel magnesium alloys ZEK100 and AX30 and their mechanical suitability as biodegradable implants. Materials. 2011;4(6):1144–1167. doi:10.3390/ma4061144
- Gu XN, Xie XH, Li N, et al. In vitro and in vivo studies on a Mg-Sr binary alloy system developed as a new kind of biodegradable metal. Acta Biomater. 2012;8(6):2360–2374. doi:10.1016/j.actbio.2012.02.018
- Willbold E, Kaya AA, Kaya RA, et al. Corrosion of magnesium alloy AZ31 screws is dependent on the implantation site. Mater Sci Eng: B. 2011;176(20):1835–1840. doi:10.1016/j.mseb.2011.02.010
- Zheng YF, Gu XN, Witte F. Biodegradable metals. Mater Sci Eng R Reports. 2014;77:1–34. doi:10.1016/j.mser.2014.01.001
- Zhang S, Zhang X, Zhao C, et al. Research on an Mg-Zn alloy as a degradable biomaterial. Acta Biomater. 2010;6(2):626–640. doi:10.1016/j.actbio.2009.06.028
- Ding P, Liu Y, He X, et al. In vitro and in vivo biocompatibility of Mg–Zn–Ca alloy operative clip. Bioactive Mater. 2019;4:236–244. doi:10.1016/j.bioactmat.2019.07.002
- Juel C. Regulation of pH in human skeletal muscle: adaptations to physical activity. Acta Physiol. 2008;193(1):17–24. doi:10.1111/j.1748-1716.2008.01840.x
- Schneider LA, Korber A, Grabbe S, et al. Influence of pH on wound-healing: a new perspective for wound-therapy? Arch Dermatol Res. 2007;298(9):413–420. doi:10.1007/s00403-006-0713-x
- Proksch E. Ph in nature, humans and skin. J Dermatol. 2018;45(9):1044–1052. doi:10.1111/1346-8138.14489
- Gautam M, Benson CJ, Sluka KA. Increased response of muscle sensory neurons to decreases in pH after muscle inflammation. Neuroscience. 2010;170(3):893–900. doi:10.1016/j.neuroscience.2010.08.003
- Blair HC. How the osteoclast degrades bone. BioEssays. 1998;20(10):837–846. doi:10.1002/(SICI)1521-1878(199810)20:10<837::AID-BIES9>3.0.CO;2-D
- Dargaville TR, Farrugia BL, Broadbent JA, et al. Sensors and imaging for wound healing: A review. Biosens Bioelectron. 2013;41:30–42. doi:10.1016/j.bios.2012.09.029
- Hou R-Q, Scharnagl N, Willumeit-Römer R, et al. Different effects of single protein vs. protein mixtures on magnesium degradation under cell culture conditions. Acta Biomater. 2019;98:256–268. doi:10.1016/j.actbio.2019.02.013
- Hou R-Q, Scharnagl N, Feyerabend F, et al. Exploring the effects of organic molecules on the degradation of magnesium under cell culture conditions. Corros Sci. 2018;132:35–45. doi:10.1016/j.corsci.2017.12.023
- Wang Y, Ding B-H, Gao S-Y, et al. In vitro corrosion of pure Mg in phosphate buffer solution – influences of isoelectric point and molecular structure of amino acids. Mater Sci Eng: C. 2019;105:110042. doi:10.1016/j.msec.2019.110042
- Mei D, Lamaka SV, Gonzalez J, et al. The role of individual components of simulated body fluid on the corrosion behavior of commercially pure Mg. Corros Sci. 2019;147:81–93. doi:10.1016/j.corsci.2018.11.011
- Zhang Z-Q, Wang L, Zeng M-Q, et al. Biodegradation behavior of micro-arc oxidation coating on magnesium alloy-from a protein perspective. Bioactive Mater. 2020;5(2):398–409. doi:10.1016/j.bioactmat.2020.03.005
- Gnedenkov AS, Lamaka SV, Sinebryukhov SL, et al. Electrochemical behaviour of the MA8 Mg alloy in minimum essential medium. Corros Sci. 2020;168:108552. doi:10.1016/j.corsci.2020.108552
- Yan W, Lian Y-J, Zhang Z-Y, et al. In vitro degradation of pure magnesium―the synergetic influences of glucose and albumin. Bioactive Mater. 2020;5(2):318–333. doi:10.1016/j.bioactmat.2020.02.015
- Gao Y, Wang L, Li L, et al. Effect of stress on corrosion of high-purity magnesium in vitro and in vivo. Acta Biomater. 2019;83:477–486. doi:10.1016/j.actbio.2018.11.019
- Li X, Wang Y, Chu C, et al. A study on Mg wires/poly-lactic acid composite degradation under dynamic compression and bending load for implant applications. J Mech Behav Biomed Mater. 2020;105:103707. doi:10.1016/j.jmbbm.2020.103707
- Han P, Cheng P, Zhao C, et al. Comparative study about degradation of high-purity magnesium screw in intact femoral intracondyle and in fixation of femoral intracondylar fracture. J Mater Sci Technol. 2017;33(3):305–310. doi:10.1016/j.jmst.2016.04.013
- Wang J, Giridharan V, Shanov V, et al. Flow induced corrosion behavior of absorbable magnesium-based stent. Acta Biomater. 2014;10(12):5213–5223. doi:10.1016/j.actbio.2014.08.034
- Liu D, Hu S, Yin X, et al. Degradation mechanism of magnesium alloy stent under simulated human micro-stress environment. Mater Sci Eng: C. 2018;84:263–270. doi:10.1016/j.msec.2017.12.001
- Gu X-N, Lu Y, Wang F, et al. The effect of tensile and fluid shear stress on the in vitro degradation of magnesium alloy for stent applications. Bioactive Mater. 2018;3(4):448–454. doi:10.1016/j.bioactmat.2018.08.002
- Lévesque J, Hermawan H, Dubé D, et al. Design of a pseudo-physiological test bench specific to the development of biodegradable metallic biomaterials. Acta Biomater. 2008;4(2):284–295. doi:10.1016/j.actbio.2007.09.012
- Yu Y, Lu H, Sun J. Long-term in vivo evolution of high-purity Mg screw degradation - local and systemic effects of Mg degradation products. Acta Biomater. 2018;71:215–224. doi:10.1016/j.actbio.2018.02.023
- Jahnen-Dechent W, Ketteler M. Magnesium basics. Clin Kidney J. 2012;Suppl_1:i3–i14. doi:10.1093/ndtplus/sfr163
- Leem Y-H, Lee K-S, Kim J-H, et al. Magnesium ions facilitate integrin alpha 2- and alpha 3-mediated proliferation and enhance alkaline phosphatase expression and activity in hBMSCs. J Tissue Eng Regen Med. 2014;10(10):E527–E536. doi:10.1002/term.1861
- Marzia L, Federica D, Massimo M, et al. High magnesium inhibits human osteoblast differentiation in vitro. Magnes Res. 2011;24(1):1–6. doi:10.1684/mrh.2011.0271
- Lindtner RA, Castellani C, Tangl S, et al. Comparative biomechanical and radiological characterization of osseointegration of a biodegradable magnesium alloy pin and a copolymeric control for osteosynthesis. J Mech Behav Biomed Mater. 2013;28:232–243. doi:10.1016/j.jmbbm.2013.08.008
- Abed E, Moreau R. Importance of melastatin-like transient receptor potential 7 and cations (magnesium, calcium) in human osteoblast-like cell proliferation. Cell Prolif. 2007;40(6):849–865. doi:10.1111/j.1365-2184.2007.00476.x
- Wu L, Luthringer BJC, Feyerabend F, et al. Effects of extracellular magnesium on the differentiation and function of human osteoclasts. Acta Biomater. 2014;10(6):2843–2854. doi:10.1016/j.actbio.2014.02.010
- Lai Y, Li Y, Cao H, et al. Osteogenic magnesium incorporated into PLGA/TCP porous scaffold by 3D printing for repairing challenging bone defect. Biomaterials. 2019;197:207–219. doi:10.1016/j.biomaterials.2019.01.013
- Bessa-Gonçalves M, Silva AM, Brás JP, et al. Fibrinogen and magnesium combination biomaterials modulate macrophage phenotype, NF-kB signaling and crosstalk with mesenchymal stem/stromal cells. Acta Biomater. 2020;114:471–484. doi:10.1016/j.actbio.2020.07.028
- Costantino MD, Schuster A, Helmholz H, et al. Inflammatory response to magnesium-based biodegradable implant materials. Acta Biomater. 2020;101:598–608. doi:10.1016/j.actbio.2019.10.014
- Zreiqat H, Howlett CR, Zannettino A, et al. Mechanisms of magnesium-stimulated adhesion of osteoblastic cells to commonly used orthopaedic implants. J Biomed Mater Res. 2002;62(2):175–184. doi:10.1002/jbm.10270
- Zhang J, Ma X, Lin D, et al. Magnesium modification of a calcium phosphate cement alters bone marrow stromal cell behavior via an integrin-mediated mechanism. Biomaterials. 2015;53:251–264. doi:10.1016/j.biomaterials.2015.02.097
- Bartsch I, Willbold E, Rosenhahn B, et al. Non-invasive pH determination adjacent to degradable biomaterials in vivo. Acta Biomater. 2014;10(1):34–39. doi:10.1016/j.actbio.2013.08.047
- Ma J, Zhao N, Betts L, et al. Bio-Adaption between magnesium alloy stent and the blood vessel: A review. J Mater Sci Technol. 2016;32(9):815–826. doi:10.1016/j.jmst.2015.12.018
- Bushinsky DA. Metabolic alkalosis decreases bone calcium efflux by suppressing osteoclasts and stimulating osteoblasts. Am J Physiol-Renal Physiol. 1996;271(1):F216–F222. doi:10.1152/ajprenal.1996.271.1.F216
- Menkin V. Studies on inflammation: X. The cytological picture of an inflammatory exudate in relation to its hydrogen Ion concentration*. Am J Pathol. 1934;10(2):193–210
- Corbel M, Belleguic C, Boichot E, et al. Involvement of gelatinases (MMP-2 and MMP-9) in the development of airway inflammation and pulmonary fibrosis. Cell Biol Toxicol. 2002;18(1):51–61. doi:10.1023/A:1014471213371
- Stathopoulou K, Gaitanaki C, Beis I. Extracellular pH changes activate the p38-MAPK signalling pathway in the amphibian heart. J Exp Biol. 2006;209(7):1344–1354. doi:10.1242/jeb.02134
- Rahim MI, Eifler R, Rais B, et al. Alkalization is responsible for antibacterial effects of corroding magnesium. J Biomed Mater Res A. 2015;103(11):3526–3532. doi:10.1002/jbm.a.35503
- Song G. Control of biodegradation of biocompatable magnesium alloys. Corrosion Ence. 2007;49(4):1696–1701. doi:10.1016/j.corsci.2007.01.001
- Shigeo O. Recent progress toward hydrogen medicine: potential of molecular hydrogen for preventive and therapeutic applications. Curr Pharm Des. 2011;17(22):2241–2252. doi:10.2174/138161211797052664
- Ohta S. Molecular hydrogen as a preventive and therapeutic medical gas: initiation, development and potential of hydrogen medicine. Pharmacol Ther. 2014;144(1):1–11. doi:10.1016/j.pharmthera.2014.04.006
- Huang C-S, Kawamura T, Toyoda Y, et al. Recent advances in hydrogen research as a therapeutic medical gas. Free Radical Res. 2010;44(9):971–982. doi:10.3109/10715762.2010.500328
- Ohta S. Molecular hydrogen is a novel antioxidant to efficiently reduce oxidative stress with potential for the improvement of mitochondrial diseases. Biochim Biophys Acta. 2012;1820(5):586–594. doi:10.1016/j.bbagen.2011.05.006
- Wang C, Li J, Liu Q, et al. Hydrogen-rich saline reduces oxidative stress and inflammation by inhibit of JNK and NF-κB activation in a rat model of amyloid-beta-induced Alzheimer's disease. Neurosci Lett. 2011;491(2):127–132. doi:10.1016/j.neulet.2011.01.022
- Wan W-L, Tian B, Lin Y-J, et al. Photosynthesis-inspired H2 generation using a chlorophyll-loaded liposomal nanoplatform to detect and scavenge excess ROS. Nat Commun. 2020;11(1):534. doi:10.1038/s41467-020-14413-x
- Ohsawa I, Ishikawa M, Takahashi K, et al. Hydrogen acts as a therapeutic antioxidant by selectively reducing cytotoxic oxygen radicals. Nat Med. 2007;13:688. doi:10.1038/nm1577
- Zhang Y, Sun Q, He B, et al. Anti-inflammatory effect of hydrogen-rich saline in a rat model of regional myocardial ischemia and reperfusion. Int J Cardiol. 2011;148(1):91–95. doi:10.1016/j.ijcard.2010.08.058
- Wang F, Yu G, Liu S-Y, et al. Hydrogen-rich saline protects against renal ischemia/reperfusion injury in rats. J Surg Res. 2011;167(2):e339–e344. doi:10.1016/j.jss.2010.11.005
- Zhao D, Brown A, Wang T, et al. In vivo quantification of hydrogen gas concentration in bone marrow surrounding magnesium fracture fixation hardware using an electrochemical hydrogen gas sensor. Acta Biomater. 2018;73:559–566. doi:10.1016/j.actbio.2018.04.032
- Lin X, Tan L, Wang Q, et al. In vivo degradation and tissue compatibility of ZK60 magnesium alloy with micro-arc oxidation coating in a transcortical model. Mater Sci Eng C Mater Biol Appl. 2013;33(7):3881–3888. doi:10.1016/j.msec.2013.05.023
- Edwards JCW, Sedgwick AD, Willoughby DA. The formation of a structure with the features of synovial lining by subcutaneous injection of air: An in vivo tissue culture system. J Pathol. 1981;134(2):147–156. doi:10.1002/path.1711340205
- Kraus T, Fischerauer SF, Hänzi AC, et al. Magnesium alloys for temporary implants in osteosynthesis: in vivo studies of their degradation and interaction with bone. Acta Biomater. 2012;8(3):1230–1238. doi:10.1016/j.actbio.2011.11.008
- Sobakin AS, Wilson MA, Lehner CE, et al. Oxygen pre-breathing decreases dysbaric diseases in UW sheep undergoing hyperbaric exposure. UHM. 2008;35(1):61–67.
- Jin L, Chen C, Jia G, et al. The bioeffects of degradable products derived from a biodegradable Mg-based alloy in macrophages via heterophagy. Acta Biomater. 2020;106:428–438. doi:10.1016/j.actbio.2020.02.002
- Maradze D, Musson D, Zheng Y, et al. High magnesium corrosion rate has an effect on osteoclast and mesenchymal stem cell role during bone remodelling. Sci Rep. 2018;8(1):10003. doi:10.1038/s41598-018-28476-w
- Reinoso RF, Telfer BA, Rowland M. Tissue water content in rats measured by desiccation. J Pharmacol Toxicol Methods. 1997;38(2):87–92. doi:10.1016/S1056-8719(97)00053-1
- Amano Y, Kumazaki T. Correlation between water content and magnetization transfer ratio of the water component in bone marrow using gradient-echo imagings: normal case study. Skeletal Radiol. 1998;27(9):484–487. doi:10.1007/s002560050424
- Sommerfeldt D, Rubin C. Biology of bone and how it orchestrates the form and function of the skeleton. Eur Spine J. 2001;10(2):S86–S95.
- Roodman GD. Advances in bone biology: the osteoclast*. Endocr Rev. 1996;17(4):308–332.
- Datta HK, Ng WF, Walker JA, et al. The cell biology of bone metabolism. J Clin Pathol. 2008;61(5):577. doi:10.1136/jcp.2007.048868
- Obrien B, Carroll WM. The evolution of cardiovascular stent materials and surfaces in response to clinical drivers: a review. Acta Biomater. 2009;5(4):945–958. doi:10.1016/j.actbio.2008.11.012
- Yang N, Vafai K. Modeling of low-density lipoprotein (LDL) transport in the artery—effects of hypertension. Int J Heat Mass Transfer. 2006;49(5/6):850–867. doi:10.1016/j.ijheatmasstransfer.2005.09.019
- Xin Y, Hu T, Chu PK. In vitro studies of biomedical magnesium alloys in a simulated physiological environment: A review. Acta Biomater. 2011;7(4):1452–1459. doi:10.1016/j.actbio.2010.12.004
- Pierson D, Edick J, Tauscher A, et al. A simplified in vivo approach for evaluating the bioabsorbable behavior of candidate stent materials. J Biomed Mater Res Part B: Appl Biomater. 2012;100B(1):58–67. doi:10.1002/jbm.b.31922
- Rüedi TP, Murphy WM. AO principles of fracture management. New York: Thieme; 2000.
- Hiromoto S, Inoue M, Taguchi T, et al. In vitro and in vivo biocompatibility and corrosion behaviour of a bioabsorbable magnesium alloy coated with octacalcium phosphate and hydroxyapatite. Acta Biomater. 2015;11:520–530. doi:10.1016/j.actbio.2014.09.026
- Heymann D, Guicheux J, Gouin F, et al. Cytokines, growth factors and osteoclasts. Cytokine. 1998;10(3):155–168. doi:10.1006/cyto.1997.0277
- Hallab NJ, Jacobs JJ. Biologic effects of implant debris. Bull NYU Hosp Jt Dis. 2009;67(2):182–188.
- Erdmann N, Bondarenko A, Hewicker-Trautwein M, et al. Evaluation of the soft tissue biocompatibility of MgCa0.8 and surgical steel 316L in vivo: a comparative study in rabbits. Biomed Eng Online. 2010;9(1):63. doi:10.1186/1475-925X-9-63
- Forrester JS, Fishbein MC, Helfant RH, et al. A paradigm for restenosis based on cell biology: clues for the development of new preventive therapies. J Am Coll Cardiol. 1991;17(3):758–769. doi:10.1016/S0735-1097(10)80196-2
- Heublein B, Rohde R, Kaese V, et al. Biocorrosion of magnesium alloys: a new principle in cardiovascular implant technology? Heart. 2003;89(6):651–656. doi:10.1136/heart.89.6.651
- Loos A, Rohde R, Haverich A, et al. In vitro and in vivo biocompatibility testing of absorbable metal stents. Macromol Symp. 2007;253(1):103–108. doi:10.1002/masy.200750715
- Liu Y, Li H, Xu J, et al. Biodegradable metal-derived magnesium and sodium enhances bone regeneration by angiogenesis aided osteogenesis and regulated biological apatite formation. Chem Eng J. 2021;410:127616. doi:10.1016/j.cej.2020.127616
- Mushahary D, Sravanthi R, Li Y, et al. Zirconium, calcium, and strontium contents in magnesium based biodegradable alloys modulate the efficiency of implant-induced osseointegration. Int J Nanomed. 2013;8:2887–2902.
- Chen L, Bin Y, Zou W, et al. The influence of Sr on the microstructure, degradation and stress corrosion cracking of the Mg alloys – ZK40xSr. J Mech Behav Biomed Mater. 2017;66:187–200. doi:10.1016/j.jmbbm.2016.11.014
- Yu Z, Chen J, Yan H, et al. Degradation, stress corrosion cracking behavior and cytocompatibility of high strain rate rolled Mg-Zn-Sr alloys. Mater Lett. 2020;260:126920. doi:10.1016/j.matlet.2019.126920
- Bian D, Zhou W, Liu Y, et al. Fatigue behaviors of HP-Mg, Mg–Ca and Mg–Zn–Ca biodegradable metals in air and simulated body fluid. Acta Biomater. 2016;41:351–360. doi:10.1016/j.actbio.2016.05.031
- Jana A, Das M, Balla VK. In vitro and in vivo degradation assessment and preventive measures of biodegradable Mg alloys for biomedical applications. J Biomed Mater Res A. 2022;110(2):462–487. doi:10.1002/jbm.a.37297
- Krüger D, Galli S, Zeller-Plumhoff B, et al. High-resolution ex vivo analysis of the degradation and osseointegration of Mg-xGd implant screws in 3D. Bioactive Mater. 2022;13:37–52. doi:10.1016/j.bioactmat.2021.10.041
- Vert M, Doi Y, Hellwich K, et al. Terminology For biorelated polymers And applications (iupac recommendations 2012). Pure Appl Chem. 2012;84(2):377–410. doi:10.1351/PAC-REC-10-12-04
- Yoshizawa S, Brown A, Barchowsky A, et al. Magnesium ion stimulation of bone marrow stromal cells enhances osteogenic activity, simulating the effect of magnesium alloy degradation. Acta Biomater. 2014;10(6):2834–2842. doi:10.1016/j.actbio.2014.02.002
- Wang J, Xu J, Song B, et al. Magnesium (Mg) based interference screws developed for promoting tendon graft incorporation in bone tunnel in rabbits. Acta Biomater. 2017;63:393–410. doi:10.1016/j.actbio.2017.09.018
- Liu W, Dan X, Lu WW, et al. Importance of biomaterials In vivo microenvironment pH (μe-pH) in the regeneration process of osteoporotic bone defects. In: C Liu, H He, editor. Developments and applications of calcium phosphate bone cements. Singapore: Springer Singapore; 2018. p. 473–495.
- Fu J, Su Y, Qin Y, et al. Evolution of metallic cardiovascular stent materials: A comparative study among stainless steel, magnesium and zinc. Biomaterials. 2020;230:119641. doi:10.1016/j.biomaterials.2019.119641
- Gu X, Zheng Y, Cheng Y, et al. In vitro corrosion and biocompatibility of binary magnesium alloys. Biomaterials. 2009;30(4):484–498. doi:10.1016/j.biomaterials.2008.10.021
- Maradze D, Capel A, Martin N, et al. In vitro investigation of cellular effects of magnesium and magnesium-calcium alloy corrosion products on skeletal muscle regeneration. J Mater Sci Technol. 2019;35(11):2503–2512. doi:10.1016/j.jmst.2019.01.020
- Henderson SE, Verdelis K, Maiti S, et al. Magnesium alloys as a biomaterial for degradable craniofacial screws. Acta Biomater. 2014;10(5):2323–2332. doi:10.1016/j.actbio.2013.12.040
- Amerstorfer F, Fischerauer SF, Fischer L, et al. Long-term in vivo degradation behavior and near-implant distribution of resorbed elements for magnesium alloys WZ21 and ZX50. Acta Biomater. 2016;42:440–450. doi:10.1016/j.actbio.2016.06.025
- Meischel M, Hormann D, Draxler J, et al. Bone-implant degradation and mechanical response of bone surrounding Mg-alloy implants. J Mech Behav Biomed Mater. 2017;71:307–313. doi:10.1016/j.jmbbm.2017.03.025
- Zhang E, Xu L, Yu G, et al. In vivo evaluation of biodegradable magnesium alloy bone implant in the first 6 months implantation. J Biomed Mater Res A. 2008;90A(3):882–893. doi:10.1002/jbm.a.32132
- Grün NG, Holweg P, Tangl S, et al. Comparison of a resorbable magnesium implant in small and large growing-animal models. Acta Biomater. 2018;78:378–386. doi:10.1016/j.actbio.2018.07.044
- Oshibe N, Marukawa E, Yoda T, et al. Degradation and interaction with bone of magnesium alloy WE43 implants: A long-term follow-up in vivo rat tibia study. J Biomater Appl. 2019;33(9):1157–1167. doi:10.1177/0885328218822050
- Wolters L, Angrisani N, Seitz J, et al. Applicability of degradable magnesium LAE442 alloy plate-screw-systems in a rabbit model. Biomed Tech (Berl). 2013;58:000010151520134059.
- Myrissa A, Braeuer S, Martinelli E, et al. Gadolinium accumulation in organs of Sprague-Dawley(R) rats after implantation of a biodegradable magnesium-gadolinium alloy. Acta Biomater. 2017;48:521–529. doi:10.1016/j.actbio.2016.11.024
- Cho SY, Chae SW, Choi KW, et al. Biocompatibility and strength retention of biodegradable Mg-Ca-Zn alloy bone implants. J Biomed Mater Res B Appl Biomater. 2013;101(2):201–212. doi:10.1002/jbm.b.32813
- Wong HM, Zhao Y, Tam V, et al. In vivo stimulation of bone formation by aluminum and oxygen plasma surface-modified magnesium implants. Biomaterials. 2013;34(38):9863–9876. doi:10.1016/j.biomaterials.2013.08.052
- Marukawa E, Tamai M, Takahashi Y, et al. Comparison of magnesium alloys and poly-l-lactide screws as degradable implants in a canine fracture model. J Biomed Mater Res B Appl Biomater. 2016;104(7):1282–1289. doi:10.1002/jbm.b.33470
- Wang J, Jiang H, Bi Y, et al. Effects of gas produced by degradation of Mg-Zn-Zr alloy on cancellous bone tissue. Mater Sci Eng C Mater Biol Appl. 2015;55:556–561. doi:10.1016/j.msec.2015.05.082
- Lee J-W, Han H-S, Han K-J. Long-term clinical study and multiscale analysis of in vivo biodegradation mechanism of Mg alloy. Proc Natl Acad Sci. 2016;113(3):716.doi:10.1073/pnas.1518238113.
- Li Y, Wen C, Mushahary D, et al. Mg-Zr-Sr alloys as biodegradable implant materials. Acta Biomater. 2012;8(8):3177–3188. doi:10.1016/j.actbio.2012.04.028
- Bondarenko A, Angrisani N, Meyer-Lindenberg A, et al. Magnesium-based bone implants: immunohistochemical analysis of peri-implant osteogenesis by evaluation of osteopontin and osteocalcin expression. J Biomed Mater Res A. 2013;102(5):1449–1457. doi:10.1002/jbm.a.34828
- Grünewald TA, Ogier A, Akbarzadeh J, et al. Reaction of bone nanostructure to a biodegrading magnesium WZ21 implant – A scanning small-angle X-ray scattering time study. Acta Biomater. 2016;31:448–457. doi:10.1016/j.actbio.2015.11.049
- Grunewald TA, Rennhofer H, Hesse B, et al. Magnesium from bioresorbable implants: distribution and impact on the nano- and mineral structure of bone. Biomaterials. 2016;76:250–260. doi:10.1016/j.biomaterials.2015.10.054
- Reifenrath J, Krause A, Bormann D, et al. Profound differences in the in-vivo-degradation and biocompatibility of two very similar rare-earth containing Mg-alloys in a rabbit model. Materialwiss Werkstofftech. 2011;41(12):1054–1061. doi:10.1002/mawe.201000709
- Hampp C, Angrisani N, Reifenrath J, et al. Evaluation of the biocompatibility of two magnesium alloys as degradable implant materials in comparison to titanium as non-resorbable material in the rabbit. Mater Sci Eng C Mater Biol Appl. 2013;33(1):317–326. doi:10.1016/j.msec.2012.08.046
- Thomann M, Krause C, Bormann D, et al. Comparison of the resorbable magnesium alloys LAE442 and MgCa0.8 concerning their mechanical properties, their progress of degradation and the bone-implant-contact after 12 months implantation duration in a rabbit model. Materialwiss Werkstofftech. 2009;40(1-2):82–87. doi:10.1002/mawe.200800412
- Krause A, von der Höh N, Bormann D, et al. Degradation behaviour and mechanical properties of magnesium implants in rabbit tibiae. J Mater Sci. 2009;45(3):624–632. doi:10.1007/s10853-009-3936-3
- von der Höh N, von Rechenberg B, Bormann D, et al. Influence of different surface machining treatments of resorbable magnesium alloy implants on degradation – EDX-analysis and histology results. Materialwiss Werkstofftech. 2009;40(1-2):88–93. doi:10.1002/mawe.200800378
- Sun J, Wang J, Jiang H, et al. In vivo comparative property study of the bioactivity of coated Mg-3Zn-0.8Zr alloy. Mater Sci Eng C Mater Biol Appl. 2013;33(6):3263–3272. doi:10.1016/j.msec.2013.04.006
- Cheng M-q, Wahafu T, Jiang G-f, et al. A novel open-porous magnesium scaffold with controllable microstructures and properties for bone regeneration. Sci Rep. 2016;6:24134. doi:10.1038/srep24134
- Kraus T, Fischerauer S, Treichler S, et al. The influence of biodegradable magnesium implants on the growth plate. Acta Biomater. 2018;66:109–117. doi:10.1016/j.actbio.2017.11.031
- Wang J, Witte F, Xi T, et al. Recommendation for modifying current cytotoxicity testing standards for biodegradable magnesium-based materials. Acta Biomater. 2015;21:237–249. doi:10.1016/j.actbio.2015.04.011
- Wang J, Smith C, Sankar J, et al. Absorbable magnesium-based stent: physiological factors to consider for in vitro degradation assessments. Regen Biomater. 2015;2(1):59–69. doi:10.1093/rb/rbu015
- Grogan JA, Leen SB, McHugh PE. A physical corrosion model for bioabsorbable metal stents. Acta Biomater. 2014;10(5):2313–2322. doi:10.1016/j.actbio.2013.12.059
- Sanz-Herrera JA, Reina-Romo E, Boccaccini AR. In silico design of magnesium implants: macroscopic modeling. J Mech Behav Biomed Mater. 2018;79:181–188. doi:10.1016/j.jmbbm.2017.12.016
- Bajger P, Ashbourn JMA, Manhas V, et al. Mathematical modelling of the degradation behaviour of biodegradable metals. Biomech Model Mechanobiol. 2017;16(1):227–238. doi:10.1007/s10237-016-0812-3
- Yin J, Xu P, Wu K, et al. Macroporous and antibacterial Hydrogels enabled by incorporation of Mg-Cu alloy particles for accelerating skin wound healing. Acta Metall Sin (Engl Lett). 2022;35(5):853–866. doi:10.1007/s40195-021-01335-w
- Castiglioni S, Cazzaniga A, Albisetti W, et al. Magnesium and osteoporosis: current state of knowledge and future research directions. Nutrients. 2013;5(8):3022–3033. doi:10.3390/nu5083022
- Rude RK, Kirchen ME, Gruber HE, et al. Magnesium deficiency-induced osteoporosis in the rat: uncoupling of bone formation and bone resorption. Magnes Res. 1999;12(4):257–267.
- Rude RK, Gruber HE, Norton HJ, et al. Dietary magnesium reduction to 25% of nutrient requirement disrupts bone and mineral metabolism in the rat. Bone. 2005;37(2):211–219. doi:10.1016/j.bone.2005.04.005
- Rude RK, Gruber HE, Norton HJ, et al. Reduction of dietary magnesium by only 50% in the rat disrupts bone and mineral metabolism. Osteoporos Int. 2006;17(7):1022–1032. doi:10.1007/s00198-006-0104-3
- Navarro-González JF, Mora-Fernández C, García-Pérez J. Reviews: clinical implications of disordered magnesium homeostasis in chronic renal failure and dialysis. Semin Dial. 2009;22(1):37–44. doi:10.1111/j.1525-139X.2008.00530.x
- Yokoyama K, Takahashi N, Yada Y, et al. Prolonged maternal magnesium administration and bone metabolism in neonates. Early Hum Dev. 2010;86(3):187–191. doi:10.1016/j.earlhumdev.2010.02.007
- Grzesiak JJ, Davis GE, Kirchhofer D, et al. Regulation of alpha 2 beta 1-mediated fibroblast migration on type I collagen by shifts in the concentrations of extracellular Mg2 + and Ca2+. J Cell Biol. 1992;117(5):1109–1117. doi:10.1083/jcb.117.5.1109
- Grzesiak JJ, Pierschbacher MD. Shifts in the concentrations of magnesium and calcium in early porcine and rat wound fluids activate the cell migratory response. J Clin Invest. 1995;95(1):227–233. doi:10.1172/JCI117644
- Lin S, Yang G, Jiang F, et al. Bone regeneration: A magnesium-enriched 3D culture system that mimics the bone development microenvironment for vascularized bone regeneration. Adv Sci. 2019;6(12):1970069. doi:10.1002/advs.201970069
- Belluci MM, Schoenmaker T, Rossa-Junior C, et al. Magnesium deficiency results in an increased formation of osteoclasts. J Nutr Biochem. 2013;24(8):1488–1498. doi:10.1016/j.jnutbio.2012.12.008
- Mammoli F, Castiglioni S, Parenti S, et al. Magnesium is a key regulator of the balance between osteoclast and osteoblast differentiation in the presence of vitamin D3. Int J Mol Sci. 2019;20(2):385. doi:10.3390/ijms20020385
- Maier JAM, Malpuechbrugere C, Zimowska W, et al. Low magnesium promotes endothelial cell dysfunction: implications for atherosclerosis, inflammation and thrombosis. Biochim Biophys Acta. 2004;1689(1):13–21. doi:10.1016/j.bbadis.2004.01.002
- Maier JAM. Endothelial cells and magnesium: implications in atherosclerosis. Clin Sci. 2012;122(9):397–407. doi:10.1042/CS20110506
- Ferre S, Baldoli E, Leidi M, et al. Magnesium deficiency promotes a pro-atherogenic phenotype in cultured human endothelial cells via activation of NFkB. Biochim Biophys Acta. 2010;1802(11):952–958. doi:10.1016/j.bbadis.2010.06.016
- Rochelson B, Dowling O, Schwartz N, et al. Magnesium sulfate suppresses inflammatory responses by human umbilical vein endothelial cells (HuVECs) through the NFκB pathway. J Reprod Immunol. 2007;73(2):101–107. doi:10.1016/j.jri.2006.06.004
- Li B, Cao H, Zhao Y, et al. In vitro and in vivo responses of macrophages to magnesium-doped titanium. Sci Rep. 2017;7(1):42707. doi:10.1038/srep42707
- Chen Z, Mao X, Tan L, et al. Osteoimmunomodulatory properties of magnesium scaffolds coated with β-tricalcium phosphate. Biomaterials. 2014;35(30):8553–8565. doi:10.1016/j.biomaterials.2014.06.038
- Wang M, Yu Y, Dai K, et al. Improved osteogenesis and angiogenesis of magnesium-doped calcium phosphate cement via macrophage immunomodulation. Biomater Sci. 2016;4(11):1574–1583. doi:10.1039/C6BM00290K
- Li Y, Yue J, Yang C. Unraveling the role of Mg++ in osteoarthritis. Life Sci. 2016;147:24–29. doi:10.1016/j.lfs.2016.01.029
- Welch AA. Nutritional influences on age-related skeletal muscle loss. Proc Nutr Soc. 2014;73(1):16–33. doi:10.1017/S0029665113003698
- Sugimoto J, Romani A, Valentintorres A, et al. Magnesium decreases inflammatory cytokine production: a novel Innate immunomodulatory mechanism. J Immunol. 2012;188(12):6338–6346. doi:10.4049/jimmunol.1101765
- Gao F, Ding B, Zhou L, et al. Magnesium sulfate provides neuroprotection in lipopolysaccharide-activated primary microglia by inhibiting NF-κB pathway. J Surg Res. 2013;184(2):944–950. doi:10.1016/j.jss.2013.03.034
- Hu T, Xu H, Wang C, et al. Magnesium enhances the chondrogenic differentiation of mesenchymal stem cells by inhibiting activated macrophage-induced inflammation. Sci Rep. 2018;8(1):3406. doi:10.1038/s41598-018-21783-2
- Sternberg K, Gratz M, Koeck K, et al. Magnesium used in bioabsorbable stents controls smooth muscle cell proliferation and stimulates endothelial cells in vitro. J Biomed Mater Res Part B. 2012;100(1):41–50. doi:10.1002/jbm.b.31918
- Herencia C, Rodriguezortiz ME, Munozcastaneda JR, et al. Angiotensin II prevents calcification in vascular smooth muscle cells by enhancing magnesium influx. Eur J Clin Investig. 2015;45(11):1129–1144. doi:10.1111/eci.12517
- Shi Y, Pei J, Zhang L, et al. Understanding the effect of magnesium degradation on drug release and anti-proliferation on smooth muscle cells for magnesium-based drug eluting stents. Corros Sci. 2017;123:297–309. doi:10.1016/j.corsci.2017.04.016
- Zhou Y, Liu X, Huang N, et al. Magnesium ion leachables induce a conversion of contractile vascular smooth muscle cells to an inflammatory phenotype. J Biomed Mater Res Part B. 2019;107(4):988–1001. doi:10.1002/jbm.b.34192
- Vennemeyer JJ, Hopkins T, Kuhlmann J, et al. Effects of elevated magnesium and substrate on neuronal numbers and neurite outgrowth of neural stem/progenitor cells in vitro. Neurosci Res. 2014;84:72–78. doi:10.1016/j.neures.2014.05.001
- Liao W, Jiang M, Li M, et al. Magnesium elevation promotes neuronal differentiation while suppressing glial differentiation of primary cultured adult mouse neural progenitor cells through ERK/CREB activation. Front Neurosci. 2017;11(87):87.
- Slutsky I, Abumaria N, Wu L, et al. Enhancement of learning and memory by elevating brain magnesium. Neuron. 2010;65(2):165–177. doi:10.1016/j.neuron.2009.12.026
- Li W, Yu J, Liu Y, et al. Elevation of brain magnesium prevents synaptic loss and reverses cognitive deficits in Alzheimer’s disease mouse model. Mol Brain. 2014;7(1):65–65. doi:10.1186/s13041-014-0065-y
- Feyerabend F, Witte F, Kammal M, et al. Unphysiologically high magnesium concentrations support chondrocyte proliferation and redifferentiation. Tissue Eng. 2006;12(12):3545–3556. doi:10.1089/ten.2006.12.3545
- Dou Y, Li N, Zheng Y, et al. Effects of fluctuant magnesium concentration on phenotype of the primary chondrocytes. J Biomed Mater Res A. 2014;102(12):4455–4463.
- Raymond LA, Tingley WG, Blackstone C, et al. Glutamate receptor modulation by protein phosphorylation. J Physiol-Paris. 1994;88(3):181–192. doi:10.1016/0928-4257(94)90004-3
- Lee CH, Wen ZH, Chang YC, et al. Intra-articular magnesium sulfate (MgSO4) reduces experimental osteoarthritis and nociception: association with attenuation of N-methyl-d-aspartate (NMDA) receptor subunit 1 phosphorylation and apoptosis in rat chondrocytes. Osteoarthr Cartilage. 2009;17(11):1485–1493. doi:10.1016/j.joca.2009.05.006
- Kowaltowski AJ, Naiadasilva ES, Castilho RF, et al. Ca2+-stimulated mitochondrial reactive oxygen species generation and permeability transition are inhibited by dibucaine or Mg2+. Arch Biochem Biophys. 1998;359(1):77–81. doi:10.1006/abbi.1998.0870
- Hunter DR, Haworth RA, Southard JH. Relationship between configuration, function, and permeability in calcium-treated mitochondria. J Biol Chem. 1976;251(16):5069–5077. doi:10.1016/S0021-9258(17)33220-9
- Zhang L, Yang C, Li J, et al. High extracellular magnesium inhibits mineralized matrix deposition and modulates intracellular calcium signaling in human bone marrow-derived mesenchymal stem cells. Biochem Biophys Res Commun. 2014;450(4):1390–1395. doi:10.1016/j.bbrc.2014.07.004
- Reynolds JL, Joannides AJ, Skepper JN, et al. Human vascular smooth muscle cells undergo vesicle-mediated calcification in response to changes in extracellular calcium and phosphate concentrations: a potential mechanism for accelerated vascular calcification in ESRD. J Am Soc Nephro. 2004;15(11):2857–2867. doi:10.1097/01.ASN.0000141960.01035.28
- Rock E, Astier C, Lab C, et al. Dietary magnesium deficiency in rats enhances free radical production in skeletal muscle. J Nutr. 1995;125(5):1205–1210.
- Heppner TJ, Bonev AD, Santana LF, et al. Alkaline pH shifts Ca2 + sparks to Ca2 + waves in smooth muscle cells of pressurized cerebral arteries. Am J Physiol-Heart Circulatory Physiol. 2002;283(6):H2169–H2176. doi:10.1152/ajpheart.00603.2002
- Capellini VK, Restini CBA, Bendhack LM, et al. The effect of extracellular pH changes on intracellular pH and nitric oxide concentration in endothelial and smooth muscle cells from rat aorta. PLoS One. 2013;8(5):e62887. doi:10.1371/journal.pone.0062887
- Siskind MS, Mccoy CE, Chobanian AV, et al. Regulation of intracellular calcium by cell pH in vascular smooth muscle cells. Am J Physiol Cell Physiol. 1989;256(2):C234–C240. doi:10.1152/ajpcell.1989.256.2.C234
- Kaunitz JD, Yamaguchi DT. TNAP, TrAP, ecto-purinergic signaling, and bone remodeling. J Cell Biochem. 2008;105(3):655–662. doi:10.1002/jcb.21885
- Kohn DH, Sarmadi M, Helman JI, et al. Effects of pH on human bone marrow stromal cells in vitro: implications for tissue engineering of bone. J Biomed Mater Res. 2002;60(2):292–299. doi:10.1002/jbm.10050
- Fliefel R, Popov C, Tröltzsch M, et al. Mesenchymal stem cell proliferation and mineralization but not osteogenic differentiation are strongly affected by extracellular pH. J Cranio-Max Surg. 2016;44(6):715–724. doi:10.1016/j.jcms.2016.03.003
- Liu W, Wang T, Yang C, et al. Alkaline biodegradable implants for osteoporotic bone defects—importance of microenvironment pH. Osteoporos Int. 2016;27(1):93–104. doi:10.1007/s00198-015-3217-8
- Kaysinger KK, Ramp WK. Extracellular pH modulates the activity of cultured human osteoblasts. J Cell Biochem. 1998;68(1):83–89. doi:10.1002/(SICI)1097-4644(19980101)68:1<83::AID-JCB8>3.0.CO;2-S
- Pan H, Shen Y, Wen C, et al. Role of pH-The essential step for osteoporotic bone regeneration. Bone. 2010;47:S444. doi:10.1016/j.bone.2010.09.298
- Shen Y, Liu W, Lin K, et al. Interfacial pH: a critical factor for osteoporotic bone regeneration. Langmuir. 2011;27(6):2701–2708. doi:10.1021/la104876w
- Lengheden A, Jansson L. PH effects on experimental wound healing of human fibroblasts in vitro. Eur J Oral Sci. 1995;103(3):148–155. doi:10.1111/j.1600-0722.1995.tb00016.x
- Padan E, Bibi E, Ito M, et al. Alkaline pH homeostasis in bacteria: New insights. Biochim Biophys Acta Biomembr. 2005;1717(2):67–88. doi:10.1016/j.bbamem.2005.09.010
- Tan J, Wang D, Cao H, et al. Effect of local alkaline microenvironment on the behaviors of bacteria and osteogenic cells. ACS Appl Mater Interfaces. 2018;10(49):42018–42029. doi:10.1021/acsami.8b15724
- Zhao P, Jin Z, Chen Q, et al. Local generation of hydrogen for enhanced photothermal therapy. Nat Commun. 2018;9(1):4241. doi:10.1038/s41467-018-06630-2
- Yang T, Jin Z, Wang Z, et al. Intratumoral high-payload delivery and acid-responsive release of H2 for efficient cancer therapy using the ammonia borane-loaded mesoporous silica nanomedicine. Appl Mater Today. 2018;11:136–143. doi:10.1016/j.apmt.2018.01.008
- Nan M, Yangmei C, Bangcheng Y. Magnesium metal – a potential biomaterial with antibone cancer properties. J Biomed Mater Res A. 2014;102(8):2644–2651. doi:10.1002/jbm.a.34933
- Yu S, Li G, Zhao P, et al. NIR-laser-controlled hydrogen-releasing PdH nanohydride for synergistic hydrogen-photothermal antibacterial and wound-healing therapies. Adv Funct Mater. 2019;29(50):1905697. doi:10.1002/adfm.201905697
- Wan W-L, Lin Y-J, Chen H-L, et al. In situ nanoreactor for photosynthesizing H2 gas to mitigate oxidative stress in tissue inflammation. J Am Chem Soc. 2017;139(37):12923–12926. doi:10.1021/jacs.7b07492
- He Y, Zhang B, Chen Y, et al. Image-guided hydrogen gas delivery for protection from myocardial ischemia–reperfusion injury via microbubbles. ACS Appl Mater Interfaces. 2017;9(25):21190–21199. doi:10.1021/acsami.7b05346
- Hanaoka T, Kamimura N, Yokota T, et al. Molecular hydrogen protects chondrocytes from oxidative stress and indirectly alters gene expressions through reducing peroxynitrite derived from nitric oxide. Med Gas Res. 2011;1(1):18. doi:10.1186/2045-9912-1-18
- Liu Y, Wang D-L, Huang Y-C, et al. Hydrogen inhibits the osteoclastogenesis of mouse bone marrow mononuclear cells. Mater Sci Eng: C. 2020;110:110640. doi:10.1016/j.msec.2020.110640
- Liu WC, Chen S, Zheng L, et al. Angiogenesis assays for the evaluation of angiogenic properties of orthopaedic biomaterials – A general review. Adv Healthcare Mater. 2017;6(5):1600434. doi:10.1002/adhm.201600434
- Chen Y, Xiong S, Zhao F, et al. Effect of magnesium on reducing the UV-induced oxidative damage in marrow mesenchymal stem cells. J Biomed Mater Res A. 2019;0(0):1253–1263. doi:10.1002/jbm.a.36634
- Hung CC, Chaya A, Liu K, et al. The role of magnesium ions in bone regeneration involves the canonical Wnt signaling pathway. Acta Biomater. 2019;98:246–255. doi:10.1016/j.actbio.2019.06.001
- Wang Z, Wang X, Tian Y, et al. Degradation and osteogenic induction of a SrHPO4-coated Mg–Nd–Zn–Zr alloy intramedullary nail in a rat femoral shaft fracture model. Biomaterials. 2020;247:119962. doi:10.1016/j.biomaterials.2020.119962
- Chen X, Wang Z, Duan N, et al. Osteoblast–osteoclast interactions. Connect Tissue Res. 2018;59(2):99–107. doi:10.1080/03008207.2017.1290085
- Gao B, Deng R, Chai Y, et al. Macrophage-lineage TRAP+ cells recruit periosteum-derived cells for periosteal osteogenesis and regeneration. J Clin Invest. 2019;129(6):2578–2594. doi:10.1172/JCI98857
- Rude RK, Gruber HE, Wei LY, et al. Magnesium deficiency: effect on bone and mineral metabolism in the mouse. Calcif Tissue Int. 2003;72(1):32–41. doi:10.1007/s00223-001-1091-1
- Berglund IS, Jacobs BY, Allen KD, et al. Peri-implant tissue response and biodegradation performance of a Mg–1.0Ca–0.5Sr alloy in rat tibia. Mater Sci Eng: C. 2016;62:79–85. doi:10.1016/j.msec.2015.12.002
- Witte F, Ulrich H, Rudert M, et al. Biodegradable magnesium scaffolds: part 1: appropriate inflammatory response. J Biomed Mater Res A. 2007;81(3):748–756. doi:10.1002/jbm.a.31170
- Witte F, Ulrich H, Palm C, et al. Biodegradable magnesium scaffolds: part II: peri-implant bone remodeling. J Biomed Mater Res A. 2007;81(3):757–765. doi:10.1002/jbm.a.31293
- Witte F, Reifenrath J, Müller PP, et al. Cartilage repair on magnesium scaffolds used as a subchondral bone replacement. Materialwiss Werkstofftech. 2006;37(6):504–508. doi:10.1002/mawe.200600027
- Bobe K, Willbold E, Morgenthal I, et al. In vitro and in vivo evaluation of biodegradable, open-porous scaffolds made of sintered magnesium W4 short fibres. Acta Biomater. 2013;9(10):8611–8623. doi:10.1016/j.actbio.2013.03.035
- Thormann U, Alt V, Heimann L, et al. The biocompatibility of degradable magnesium interference screws: an experimental study with sheep. BioMed Res Int. 2015;2015:15. doi:10.1155/2015/943603
- Huehnerschulte TA, Reifenrath J, von Rechenberg B, et al. In vivo assessment of the host reactions to the biodegradation of the two novel magnesium alloys ZEK100 and AX30 in an animal model. Biomed Eng Online. 2012;11(1):14. doi:10.1186/1475-925X-11-14
- Wang J, Wu Y, Li H, et al. Magnesium alloy based interference screw developed for ACL reconstruction attenuates peri-tunnel bone loss in rabbits. Biomaterials. 2018;157:86–97. doi:10.1016/j.biomaterials.2017.12.007
- Li G, Zhang L, Wang L, et al. Dual modulation of bone formation and resorption with zoledronic acid-loaded biodegradable magnesium alloy implants improves osteoporotic fracture healing: an in vitro and in vivo study. Acta Biomater. 2018;65:486–500. doi:10.1016/j.actbio.2017.10.033
- Sivaraj KK, Adams RH. Blood vessel formation and function in bone. Development. 2016;143(15):2706. doi:10.1242/dev.136861
- Chen H, Hu B, Lv X, et al. Prostaglandin E2 mediates sensory nerve regulation of bone homeostasis. Nat Commun. 2019;10(1):181. doi:10.1038/s41467-018-08097-7
- Grüneboom A, Hawwari I, Weidner D, et al. A network of trans-cortical capillaries as mainstay for blood circulation in long bones. Nat Metab. 2019;1(2):236–250. doi:10.1038/s42255-018-0016-5
- Trajanoska K, Rivadeneira F, Kiel DP, et al. Genetics of bone and muscle interactions in humans. Curr Osteoporos Rep. 2019;17(2):86–95. doi:10.1007/s11914-019-00505-1
- Bonewald L. Use it or lose it to age: a review of bone and muscle communication. Bone. 2019;120:212–218. doi:10.1016/j.bone.2018.11.002
- Zhai Z, Qu X, Li H, et al. The effect of metallic magnesium degradation products on osteoclast-induced osteolysis and attenuation of NF-kappaB and NFATc1 signaling. Biomaterials. 2014;35(24):6299–6310. doi:10.1016/j.biomaterials.2014.04.044
- Cackowski FC, Anderson JL, Patrene KD, et al. Osteoclasts are important for bone angiogenesis. Blood. 2010;115(1):140–149. doi:10.1182/blood-2009-08-237628
- Xie H, Cui Z, Wang L, et al. PDGF-BB secreted by preosteoclasts induces angiogenesis during coupling with osteogenesis. Nat Med. 2014;20(11):1270–1278. doi:10.1038/nm.3668
- Kusumbe AP, Ramasamy SK, Adams RH. Coupling of angiogenesis and osteogenesis by a specific vessel subtype in bone. Nature. 2014;507(7492):323–328. doi:10.1038/nature13145
- Ramasamy SK, Kusumbe AP, Wang L, et al. Endothelial notch activity promotes angiogenesis and osteogenesis in bone. Nature. 2014;507(7492):376–380. doi:10.1038/nature13146
- Portal-Núñez S, Lozano D, Esbrit P. Role of angiogenesis on bone formation. Histol Histopathol. 2012;27(5):559–566.
- Han HS, Jun I, Seok HK, et al. Biodegradable magnesium alloys promote angio-osteogenesis to enhance bone repair. Advanced Science. 2020;7(15):12.
- Li HF, Xie XH, Zhao K, et al. In vitro and in vivo studies on biodegradable CaMgZnSrYb high-entropy bulk metallic glass. Acta Biomater. 2013;9(10):8561–8573. doi:10.1016/j.actbio.2013.01.029
- Chen K, Xie X, Tang H, et al. In vitro and in vivo degradation behavior of Mg–2Sr–Ca and Mg–2Sr–Zn alloys. Bioactive Mater. 2020;5(2):275–285. doi:10.1016/j.bioactmat.2020.02.014
- Gu X, Wang F, Xie X, et al. In vitro and in vivo studies on as-extruded Mg- 5.25wt.%Zn-0.6wt.%Ca alloy as biodegradable metal. Sci China Mater. 2018;61(4):619–628. doi:10.1007/s40843-017-9205-x
- Cheng P, Zhao C, Han P, et al. Site-Dependent osseointegration of biodegradable high-purity magnesium for orthopedic implants in femoral shaft and femoral condyle of New Zealand rabbits. J Mater Sci Technol. 2016;32(9):883–888. doi:10.1016/j.jmst.2016.03.012
- Klauser H. Internal fixation of three-dimensional distal metatarsal I osteotomies in the treatment of hallux valgus deformities using biodegradable magnesium screws in comparison to titanium screws. Foot Ankle Surg. 2019;25(3):398–405. doi:10.1016/j.fas.2018.02.005
- Thomann M, Krause C, Angrisani N, et al. Influence of a magnesium-fluoride coating of magnesium-based implants (MgCa0.8) on degradation in a rabbit model. J Biomed Mater Res A. 2010;93A(4):1609–1619.
- Castellani C, Lindtner RA, Hausbrandt P, et al. Bone-implant interface strength and osseointegration: biodegradable magnesium alloy versus standard titanium control. Acta Biomater. 2011;7(1):432–440. doi:10.1016/j.actbio.2010.08.020
- Naujokat H, Seitz JM, Acil Y, et al. Osteosynthesis of a cranio-osteoplasty with a biodegradable magnesium plate system in miniature pigs. Acta Biomater. 2017;62:434–445. doi:10.1016/j.actbio.2017.08.031
- Imwinkelried T, Beck S, Schaller B. Pre-clinical testing of human size magnesium implants in miniature pigs: implant degradation and bone fracture healing at multiple implantation sites. Mater Sci Eng: C. 2020;108:110389. doi:10.1016/j.msec.2019.110389
- Schaller B, Saulacic N, Beck S, et al. Osteosynthesis of partial rib osteotomy in a miniature pig model using human standard-sized magnesium plate/screw systems: effect of cyclic deformation on implant integrity and bone healing. J Cranio-Max Surg. 2017;45(6):862–871. doi:10.1016/j.jcms.2017.03.018
- Dong J, Tan L, Yang J, et al. In vitro and in vivo studies on degradation and bone response of Mg-Sr alloy for treatment of bone defect. Mater Technol. 2018;33(6):387–397. doi:10.1080/10667857.2018.1452587
- Delmas PD, Stenner D, Wahner HW, et al. Increase in serum bone gamma-carboxyglutamic acid protein with aging in women. implications for the mechanism of age-related bone loss. J Clin Invest. 1983;71(5):1316–1321. doi:10.1172/JCI110882
- Witte F, Kaese V, Haferkamp H, et al. In vivo corrosion of four magnesium alloys and the associated bone response. Biomaterials. 2005;26(17):3557–3563. doi:10.1016/j.biomaterials.2004.09.049
- Han P, Cheng P, Zhang S, et al. In vitro and in vivo studies on the degradation of high-purity Mg (99.99wt.%) screw with femoral intracondylar fractured rabbit model. Biomaterials. 2015;64:57–69. doi:10.1016/j.biomaterials.2015.06.031
- Tian L, Sheng Y, Huang L, et al. An innovative Mg/Ti hybrid fixation system developed for fracture fixation and healing enhancement at load-bearing skeletal site. Biomaterials. 2018;180:173–183. doi:10.1016/j.biomaterials.2018.07.018
- Wang J, Xu J, Fu W, et al. Biodegradable magnesium screws accelerate fibrous tissue mineralization at the tendon-bone insertion in anterior cruciate ligament reconstruction model of rabbit. Sci Rep. 2017;7(1):40369. doi:10.1038/srep40369
- Zhao D, Huang S, Lu F, et al. Vascularized bone grafting fixed by biodegradable magnesium screw for treating osteonecrosis of the femoral head. Biomaterials. 2016;81:84–92. doi:10.1016/j.biomaterials.2015.11.038
- Li Y, Liu G, Zhai Z, et al. Antibacterial properties of magnesium in vitro and in an in vivo model of implant-associated methicillin-resistant Staphylococcus aureu infection. Antimicrob Agents Chemother. 2014;58(12):7586–7591. doi:10.1128/AAC.03936-14
- Mushahary D, Wen C, Kumar JM, et al. Collagen type-I leads to in vivo matrix mineralization and secondary stabilization of Mg-Zr-Ca alloy implants. Colloids Surf B Biointerfaces. 2014;122:719–728. doi:10.1016/j.colsurfb.2014.08.005
- Zeller-Plumhoff B, Malich C, Krüger D, et al. Analysis of the bone ultrastructure around biodegradable Mg–xGd implants using small angle X-ray scattering and X-ray diffraction. Acta Biomater. 2020;101:637–645. doi:10.1016/j.actbio.2019.11.030
- Liebi M, Lutz-Bueno V, Guizar-Sicairos M, et al. 3D nanoscale analysis of bone healing around degrading Mg implants evaluated by X-ray scattering tensor tomography. Acta Biomater. 2021;134:804–817. doi:10.1016/j.actbio.2021.07.060
- Colombo A, Karvouni E. Biodegradable stents “fulfilling the mission and stepping away”. Circulation. 2000;102(4):371–373. doi:10.1161/01.CIR.102.4.371
- Ferre S, Mazur A, Maier JAM. Low-magnesium induces senescent features in cultured human endothelial cells. Magnes Res. 2007;20(1):66–71.
- Kostov K, Halacheva L. Role of magnesium deficiency in promoting atherosclerosis, endothelial dysfunction, and arterial stiffening as risk factors for hypertension. Int J Mol Sci. 2018;19(6):1724. doi:10.3390/ijms19061724
- Maier JAM, Bernardini D, Rayssiguier Y, et al. High concentrations of magnesium modulate vascular endothelial cell behaviour in vitro. Biochim Biophys Acta. 2004;1689(1):6–12. doi:10.1016/j.bbadis.2004.02.004
- Lapidos KA, Woodhouse EC, Kohn EC, et al. Mg++-induced endothelial cell migration: substratum selectivity and receptor-involvement. Angiogenesis. 2001;4(1):21–28. doi:10.1023/A:1016619414817
- Louvet L, Bazin D, Buchel J, et al. Characterisation of calcium phosphate crystals on calcified human aortic vascular smooth muscle cells and potential role of magnesium. PLOS ONE. 2015;10(1):e0115342. doi:10.1371/journal.pone.0115342
- Oca AMD, Guerrero F, Martinez-Moreno JM, et al. Magnesium inhibits Wnt/β-catenin activity and reverses the osteogenic transformation of vascular smooth muscle cells. PLoS ONE. 2014;9(2):e89525. doi:10.1371/journal.pone.0089525
- Kircelli F, Peter M, Ok ES, et al. Magnesium reduces calcification in bovine vascular smooth muscle cells in a dose-dependent manner. Nephrol Dial Transplant. 2012;27(2):514–521. doi:10.1093/ndt/gfr321
- Louvet L, Buchel J, Steppan S, et al. Magnesium prevents phosphate-induced calcification in human aortic vascular smooth muscle cells. Nephrol Dial Transplant. 2013;28(4):869–878. doi:10.1093/ndt/gfs520
- Montezano AC, Zimmerman D, Yusuf H, et al. Vascular smooth muscle cell differentiation to an osteogenic phenotype involves TRPM7 modulation by magnesium. Hypertension. 2010;56(3):453–462. doi:10.1161/HYPERTENSIONAHA.110.152058
- Ma J, Zhao N, Zhu D. Biphasic responses of human vascular smooth muscle cells to magnesium ion. J Biomed Mater Res A. 2016;104(2):347–356. doi:10.1002/jbm.a.35570
- Weglicki WB, Phillips TM. Pathobiology of magnesium deficiency: a cytokine/neurogenic inflammation hypothesis. Am J Physiol-Regulatory. Integr Comp Physiol. 1992;263(3):R734–R737. doi:10.1152/ajpregu.1992.263.3.R734
- Jin L, Wu J, Yuan G, et al. In vitro study of the inflammatory cells response to biodegradable Mg-based alloy extract. Plos One. 2018;13(3):e0193276.
- Su NY, Peng TC, Tsai PS, et al. Phosphoinositide 3-kinase/Akt pathway is involved in mediating the anti-inflammation effects of magnesium sulfate. J Surg Res. 2013;185(2):726–732. doi:10.1016/j.jss.2013.06.030
- Li XW, Huang Q, Liu L, et al. Reduced inflammatory response by incorporating magnesium into porous TiO2 coating on titanium substrate. Colloids Surf B. 2018;171:276–284. doi:10.1016/j.colsurfb.2018.07.032
- Tong A, Jockusch S, Li Z, et al. Triple fluorescence energy transfer in covalently trichromophore-labeled DNA. J Am Chem Soc. 2001;123(51):12923–12924. doi:10.1021/ja016904h
- Jin L, Chen C, Li Y, et al. A biodegradable Mg-based alloy inhibited the inflammatory response of THP-1 cell-derived macrophages through the TRPM7-PI3K-AKT1 signaling axis. Front Immunol. 2019;10:2798–2798. doi:10.3389/fimmu.2019.02798
- Luthringer BJC, Willumeit-Römer R. Effects of magnesium degradation products on mesenchymal stem cell fate and osteoblastogenesis. Gene. 2016;575(1):9–20. doi:10.1016/j.gene.2015.08.028
- Qiao W, Wong KHM, Shen J, et al. TRPM7 kinase-mediated immunomodulation in macrophage plays a central role in magnesium ion-induced bone regeneration. Nat Commun. 2021;12(1):2885. doi:10.1038/s41467-021-23005-2
- Yang W, Hu P. Skeletal muscle regeneration is modulated by inflammation. J Orthop Translat. 2018;13:25–32. doi:10.1016/j.jot.2018.01.002
- Tidball JG, Villalta SA. Regulatory interactions between muscle and the immune system during muscle regeneration. Am J Physiol-Reg Integr Comparative Physiol. 2010;298(5):R1173–R1187. doi:10.1152/ajpregu.00735.2009
- Saclier M, Yacoubyoussef H, Mackey AL, et al. Differentially activated macrophages orchestrate myogenic precursor cell fate during human skeletal muscle regeneration. Stem Cells. 2013;31(2):384–396. doi:10.1002/stem.1288
- Greising SM, Rivera JC, Goldman SM, et al. Unwavering pathobiology of volumetric muscle loss injury. Sci Rep. 2017;7(1):13179–13179. doi:10.1038/s41598-017-13306-2
- Grasman JM, Zayas MJ, Page RL, et al. Biomimetic scaffolds for regeneration of volumetric muscle loss in skeletal muscle injuries. Acta Biomater. 2015;25:2–15. doi:10.1016/j.actbio.2015.07.038
- Wang H, Morales RTT, Cui X, et al. A photoresponsive hyaluronan hydrogel nanocomposite for dynamic macrophage immunomodulation. Adv Healthcare Mater. 2018;8(4):1801234. doi:10.1002/adhm.201801234
- Lin X, Yang S, Lai K, et al. Orthopedic implant biomaterials with both osteogenic and anti-infection capacities and associated in vivo evaluation methods. Nanomed: Nanotechnol. Biol Med. 2017;13(1):123–142.
- Cao H, Tang K, Liu X. Bifunctional galvanics mediated selective toxicity on titanium. Mater Horiz. 2018;5(2):264–267. doi:10.1039/C7MH00884H
- Feng H, Wang G, Jin W, et al. Systematic study of inherent antibacterial properties of magnesium-based biomaterials. ACS Appl Mater Interfaces. 2016;8(15):9662–9673. doi:10.1021/acsami.6b02241
- Leung YH, Ng AMC, Xu X, et al. Mechanisms of antibacterial Activity of MgO: Non-ROS mediated toxicity of MgO nanoparticles towards Escherichia coli. Small. 2014;10(6):1171–1183. doi:10.1002/smll.201302434
- Pan X, Wang Y, Chen Z, et al. Investigation of antibacterial activity and related mechanism of a series of nano-Mg(OH)2. ACS Appl Mater Interfaces. 2013;5(3):1137–1142. doi:10.1021/am302910q
- Aničic N, Vukomanovic M, Koklič T, et al. Fewer defects in the surface slows the hydrolysis rate, decreases the ROS generation potential, and improves the Non-ROS Antimicrobial activity of MgO. Small. 2018;14(26):1800205. doi:10.1002/smll.201800205
- Meng Y, Zhang D, Jia X, et al. Antimicrobial Activity of nano-magnesium hydroxide against oral bacteria and application in root canal sealer. Med Sci Monit. 2020;26:e922920.
- Robinson DA, Griffith RW, Shechtman D, et al. In vitro antibacterial properties of magnesium metal against Escherichia coli, Pseudomonas aeruginosa and Staphylococcus aureus. Acta Biomater. 2010;6(5):1869–1877. doi:10.1016/j.actbio.2009.10.007
- Zeng J, Ren L, Yuan Y, et al. Short-term effect of magnesium implantation on the osteomyelitis modeled animals induced by Staphylococcus aureus. J Mater Sci: Mater Med. 2013;24(10):2405–2416. doi:10.1007/s10856-013-4982-6
- Zhao W, Wang J, Weiyang J, et al. A novel biodegradable Mg-1Zn-0.5Sn alloy: mechanical properties, corrosion behavior, biocompatibility, and antibacterial activity. J Magnesium Alloys. 2020;8(2):374–386. doi:10.1016/j.jma.2020.02.008
- He G, Wu Y, Zhang Y, et al. Addition of Zn to the ternary Mg-Ca-Sr alloys significantly improves their antibacterial properties. J Mater Chem B. 2015;3(32):6676–6689. doi:10.1039/C5TB01319D
- Tie D, Feyerabend F, Muller WD, et al. Antibacterial biodegradable Mg-Ag alloys. Eur Cell Mater. 2013;25:284–298. doi:10.22203/eCM.v025a20
- Liu C, Fu X, Pan H, et al. Biodegradable Mg-Cu alloys with enhanced osteogenesis, angiogenesis, and long-lasting antibacterial effects. Sci Rep. 2016;6(1):27374. doi:10.1038/srep27374
- Qin H, Zhao Y, An Z, et al. Enhanced antibacterial properties, biocompatibility, and corrosion resistance of degradable Mg-Nd-Zn-Zr alloy. Biomaterials. 2015;53:211–220. doi:10.1016/j.biomaterials.2015.02.096
- Li Y, Liu L, Wan P, et al. Biodegradable Mg-Cu alloy implants with antibacterial activity for the treatment of osteomyelitis: In vitro and in vivo evaluations. Biomaterials. 2016;106:250–263. doi:10.1016/j.biomaterials.2016.08.031
- Brooks EK, Ahn R, Tobias ME, et al. Magnesium alloy AZ91 exhibits antimicrobial properties in vitro but not in vivo. J Biomed Mater Res Part B: Appl Biomater. 2018;106(1):221–227. doi:10.1002/jbm.b.33839
- Hou P, Zhao C, Cheng P, et al. Reduced antibacterial property of metallic magnesium in vivo. Biomed Mater. 2016;12(1):015010. doi:10.1088/1748-605X/12/1/015010
- Fei J, Wen X, Lin X, et al. Biocompatibility and neurotoxicity of magnesium alloys potentially used for neural repairs. Mater Sci Eng: C. 2017;78:1155–1163. doi:10.1016/j.msec.2017.04.106
- Monfared A, Ghaee A, Ebrahimi-Barough S. Fabrication of tannic acid/poly(N-vinylpyrrolidone) layer-by-layer coating on Mg-based metallic glass for nerve tissue regeneration application. Colloids Surf B. 2018;170:617–626. doi:10.1016/j.colsurfb.2018.06.060
- Vennemeyer JJ, Hopkins T, Hershcovitch M, et al. Initial observations on using magnesium metal in peripheral nerve repair. J Biomater Appl. 2014;29(8):1145–1154. doi:10.1177/0885328214553135
- Hopkins TM, Little KJ, Vennemeyer JJ, et al. Short and long gap peripheral nerve repair with magnesium metal filaments. J Biomed Mater Res A. 2017;105(11):3148–3158. doi:10.1002/jbm.a.36176
- Li B.-h, Yang K, Wang X. Biodegradable magnesium wire promotes regeneration of compressed sciatic nerves. Neural Regen Res. 2016;11(12):2012–2017. doi:10.4103/1673-5374.197146
- Li M, Jiang M, Gao Y, et al. Current status and outlook of biodegradable metals in neuroscience and their potential applications as cerebral vascular stent materials. Bioactive Mater. 2022;11:140–153. doi:10.1016/j.bioactmat.2021.09.025
- Wang L, Lu C, Yang S, et al. A fully biodegradable and self-electrified device for neuroregenerative medicine. Sci Adv. 2020;6(50):eabc6686. doi:10.1126/sciadv.abc6686
- Yue J, Jin S, Gu S, et al. High concentration magnesium inhibits extracellular matrix calcification and protects articular cartilage via Erk/autophagy pathway. J Cell Physiol. 2019;234(12):23190–23201. doi:10.1002/jcp.28885
- Shimaya M, Muneta T, Ichinose S, et al. Magnesium enhances adherence and cartilage formation of synovial mesenchymal stem cells through integrins. Osteoar Cartilage. 2010;18(10):1300–1309. doi:10.1016/j.joca.2010.06.005
- Wan W-L, Lin Y-J, Shih P-C, et al. An In situ depot for continuous evolution of gaseous H2 mediated by a magnesium passivation/activation cycle for treating osteoarthritis. Angew Chem Int Ed. 2018;57(31):9875–9879. doi:10.1002/anie.201806159
- Mussoni L, Sironi L, Tedeschi L, et al. Magnesium inhibits arterial thrombi after vascular injury in Rat: in vivo impairment of coagulation. Thromb Haemostasis. 2001;86(5):1292–1295.
- Bussiere FOI, Gueux E, Rock E, et al. Protective effect of calcium deficiency on the inflammatory response in magnesium-deficient rats. Eur J Nutr. 2002;41(5):197–202. doi:10.1007/s00394-002-0376-0
- Malpuechbrugere C, Rock E, Astier C, et al. Exacerbated immune stress response during experimental magnesium deficiency results from abnormal cell calcium homeostasis. Life Sci. 1998;63(20):1815–1822. doi:10.1016/S0024-3205(98)00455-X
- Bussiere FI, Mazur A, Fauquert JL, et al. High magnesium concentration in vitro decreases human leukocyte activation. Magnes Res. 2002;15:43–48.
- Heming TA, Bulayeva NN, Bidani A. Cell alkalosis elevates cytosolic Ca2 + in rabbit resident alveolar macrophages. Clin Sci. 2003;105(1):21–28. doi:10.1042/CS20030004
- Lowry CL, Mcgeehan GM, Vine HL. Metal ion stabilization of the conformation of a recombinant 19-kDa catalytic fragment of human fibroblast collagenase. Proteins. 1992;12(1):42–48. doi:10.1002/prot.340120106
- Aikawa JK. Magnesium: its biologic significance. New York: CRC Press; 2019.
- Wacker WEC, Parisi AF. Magnesium metabolism. N Engl J Med. 1968;278(12):658. doi:10.1056/NEJM196803212781205
- Pei J, Yu X, Bian J, et al. Acidosis antagonizes intracellular calcium response to κ-opioid receptor stimulation in the rat heart. Am J Physiol. 1999;277(3 Pt 1):C492. doi:10.1152/ajpcell.1999.277.3.C492
- Križaj D, Mercer AJ, Thoreson WB, et al. Intracellular pH modulates inner segment calcium homeostasis in vertebrate photoreceptors. Am J Physiol-Cell Physiol. 2011;300(1):C187–C197. doi:10.1152/ajpcell.00264.2010
- Yan Jf, Qin Wp, Xiao Bc, et al. Pathological calcification in osteoarthritis: an outcome or a disease initiator? Biol Rev. 2020;95(4):960–985. doi:10.1111/brv.12595
- Fuerst M, Niggemeyer O, Lammers L, et al. Articular cartilage mineralization in osteoarthritis of the hip. BMC Musculoskel Disord. 2009;10(1):166. doi:10.1186/1471-2474-10-166
- Macmullan P, Mcmahon G, Mccarthy GM. Detection of basic calcium phosphate crystals in osteoarthritis. Joint Bone Spine. 2011;78(4):358–363. doi:10.1016/j.jbspin.2010.10.008
- Blanco F, Rego I, Ruizromero C. The role of mitochondria in osteoarthritis. Nat Rev Rheumatol. 2011;7(3):161–169. doi:10.1038/nrrheum.2010.213
- Szanda G, Rajki A, Gallegosandin S, et al. Effect of cytosolic Mg2 + on mitochondrial Ca2 + signaling. Pflügers Archiv: Eur J Physiol. 2009;457(4):941–954. doi:10.1007/s00424-008-0551-0
- Orrenius S, Zhivotovsky B, Nicotera P. Regulation of cell death: the calcium-apoptosis link. Nat Rev Mol Cell Biol. 2003;4(7):552–565. doi:10.1038/nrm1150
- Huard J, Li Y, Fu FH. Muscle injuries and repair: current trends in research. J Bone Joint Surg. 2002;84(5):822–832.
- Kimura N, Hirata S, Miyasaka N, et al. Injury and subsequent regeneration of muscles for activation of local innate immunity to facilitate the development and relapse of autoimmune myositis in C57BL/6 mice. Arthritis Rheum. 2015;67(4):1107–1116. doi:10.1002/art.39017
- Astier C, Rock E, Lab C, et al. Functional alterations in sarcoplasmic reticulum membranes of magnesium-deficient rat skeletal muscle as consequences of free radical-mediated process. Free Radical Biol Med. 1996;20(5):667–674. doi:10.1016/0891-5849(95)02180-9
- Jiang SX, Zheng RY, Zeng JQ, et al. Reversible inhibition of intracellular calcium influx through NMDA receptors by imidazoline I(2) receptor antagonists. Eur J Pharmacol. 2010;629(1-3):12–19. doi:10.1016/j.ejphar.2009.11.063
- Peng T, Jou M. Oxidative stress caused by mitochondrial calcium overload. Ann N Y Acad Sci. 2010;1201(1):183–188. doi:10.1111/j.1749-6632.2010.05634.x
- Castiglioni S, Maier JA. Magnesium and cancer: a dangerous liason. Magnes Res. 2011;24(3):S92. doi:10.1684/mrh.2011.0285
- Zhang Y, Ren L, Li M, et al. Preliminary study on cytotoxic effect of biodegradation of magnesium on cancer cells. J Mater Sci Technol. 2012;28(9):769–772. doi:10.1016/S1005-0302(12)60128-5
- Li M, Ren L, Li L, et al. Cytotoxic Effect on osteosarcoma MG-63 cells by degradation of magnesium. J Mater Sci Technol. 2014;30(9):888–893. doi:10.1016/j.jmst.2014.04.010
- Zan R, Wang H, Cai W, et al. Controlled release of hydrogen by implantation of magnesium induces P53-mediated tumor cells apoptosis. Bioactive Mater. 2022;9:385–396. doi:10.1016/j.bioactmat.2021.07.026
- Trachootham D, Alexandre J, Huang P, et al. Targeting cancer cells by ROS-mediated mechanisms: a radical therapeutic approach? Nat Rev Drug Discov. 2009;8:579–591. Nature Reviews Drug Discovery 8(7) (2009) 579-591. doi:10.1038/nrd2803
- Kim J, Kim J, Bae J. ROS homeostasis and metabolism: a critical liaison for cancer therapy. Exp Mol Med. 2016;48(11):e269. doi:10.1038/emm.2016.119
- Chen Y, Xiao M, Zhao H, et al. On the antitumor properties of biomedical magnesium metal. J Mater Chem B. 2015;3(5):849–858. doi:10.1039/C4TB01421A
- Anisimova N, Kiselevskiy M, Martynenko N, et al. Cytotoxicity of biodegradable magnesium alloy WE43 to tumor cells in vitro: bioresorbable implants with antitumor activity? J Biomed Mater Res Part B: Appl Biomater. 2020;108(1):167–173. doi:10.1002/jbm.b.34375
- Qiao S, Wang Y, Zan R, et al. Biodegradable Mg implants suppress the growth of ovarian tumor. ACS Biomater Sci Eng. 2020;6(3):1755–1763. doi:10.1021/acsbiomaterials.9b01703
- Li T, Xu W, Liu C, et al. Anticancer effect of biodegradable magnesium on hepatobiliary carcinoma: An In vitro and In vivo study. ACS Biomater Sci Eng. 2021;7(6):2774–2782. doi:10.1021/acsbiomaterials.1c00288
- Dai Y, Tang Y, Xu X, et al. Evaluation of the mechanisms and effects of Mg–Ag–Y alloy on the tumor growth and metastasis of the MG63 osteosarcoma cell line. J Biomed Mater Res Part B. 2019;107(8):2537–2548. doi:10.1002/jbm.b.34344
- Wu Y, He G, Zhang Y, et al. Unique antitumor property of the Mg-Ca-Sr alloys with addition of Zn. Sci Rep. 2016;6(1):21736–21736. doi:10.1038/srep21736
- Shuai C, Liu L, Yang Y, et al. Lanthanum-containing magnesium alloy with antitumor function based on increased reactive oxygen species. Appl Sci. 2018;8(11):2109. doi:10.3390/app8112109
- Li M, Wang WH, Zhu Y, et al. Molecular and cellular mechanisms for zoledronic acid-loaded magnesium-strontium alloys to inhibit giant cell tumors of bone. Acta Biomater. 2018;77:365–379. doi:10.1016/j.actbio.2018.07.028
- Khalid M, Hassani S, Abdollahi M. Metals-induced oxidative stress: An evidence-based update of advantages and disadvantages. Curr Opin Tox. 2020;20–21:55–68. doi:10.1016/j.cotox.2020.05.006
- Wagner FC, Polossek L, Yilmaz T, et al. Biodegradable magnesium versus polylactide pins for radial head fracture stabilization: a biomechanical study. J Shoulder Elbow Surg. 2020;30:365–372.
- Wagner FC, Post A, Yilmaz T, et al. Biomechanical comparison of biodegradable magnesium screws and titanium screws for operative stabilization of displaced capitellar fractures. J Shoulder Elbow Surg. 2020;29(9):1912–1919. doi:10.1016/j.jse.2020.02.009
- Chaya A, Yoshizawa S, Verdelis K, et al. Fracture healing using degradable magnesium fixation plates and screws. J Oral Maxillofac Surg. 2015;73(2):295–305. doi:10.1016/j.joms.2014.09.007
- Chaya A, Yoshizawa S, Verdelis K, et al. In vivo study of magnesium plate and screw degradation and bone fracture healing. Acta Biomater. 2015;18:262–269. doi:10.1016/j.actbio.2015.02.010
- Schaller B, Matthias Burkhard JP, Chagnon M, et al. Fracture healing and bone remodeling With human standard-sized magnesium versus polylactide–Co-glycolide plate and screw systems using a mini-swine craniomaxillofacial osteotomy fixation model. J Oral Maxillofac Surg. 2018;76(10):2138–2150. doi:10.1016/j.joms.2018.03.039
- Chow DHK, Wang J, Wan P, et al. Biodegradable magnesium pins enhanced the healing of transverse patellar fracture in rabbits. Bioactive Mater. 2021;6(11):4176–4185. doi:10.1016/j.bioactmat.2021.03.044
- Hamushan M, Cai W, Zhang Y, et al. High-purity magnesium pin enhances bone consolidation in distraction osteogenesis via regulating ptch protein activating hedgehog-alternative Wnt signaling. Bioactive Mater. 2021;6(6):1563–1574. doi:10.1016/j.bioactmat.2020.11.008
- Huang S, Wang B, Zhang X, et al. High-purity weight-bearing magnesium screw: translational application in the healing of femoral neck fracture. Biomaterials. 2020;238:119829. doi:10.1016/j.biomaterials.2020.119829
- Kim BJ, Piao Y, Wufuer M, et al. Biocompatibility and efficiency of biodegradable magnesium-based Plates and Screws in the facial fracture model of beagles. J Oral Maxillofac Surg. 2018;76(5):1055.e1–1055.e9. doi:10.1016/j.joms.2018.01.015
- Byun S-H, Lim H-K, Cheon K-H, et al. Biodegradable magnesium alloy (WE43) in bone-fixation plate and screw. J Biomed Mater Res Part B: Appl Biomater. 2020;108(6):2505–2512. doi:10.1002/jbm.b.34582
- Zheng N, Xu J, Ruan YC, et al. Magnesium facilitates the healing of atypical femoral fractures: a single-cell transcriptomic study. Mater Today. 2022;52:43–62. doi:10.1016/j.mattod.2021.11.028
- Dhandapani R, Krishnan PD, Zennifer A, et al. Additive manufacturing of biodegradable porous orthopaedic screw. Bioact Mater. 2020;5(3):458–467. doi:10.1016/j.bioactmat.2020.03.009
- Cheng P, Han P, Zhao C, et al. High-purity magnesium interference screws promote fibrocartilaginous entheses regeneration in the anterior cruciate ligament reconstruction rabbit model via accumulation of BMP-2 and VEGF. Biomaterials. 2016;81:14–26. doi:10.1016/j.biomaterials.2015.12.005
- Diekmann J, Bauer S, Weizbauer A, et al. Examination of a biodegradable magnesium screw for the reconstruction of the anterior cruciate ligament: A pilot in vivo study in rabbits. Mater Sci Eng: C. 2016;59:1100–1109. doi:10.1016/j.msec.2015.11.037
- Cheng P, Han P, Zhao C, et al. Magnesium inference screw supports early graft incorporation with inhibition of graft degradation in anterior cruciate ligament reconstruction. Sci Rep. 2016;6:26434. doi:10.1038/srep26434
- Luo Y, Zhang C, Wang J, et al. Clinical translation and challenges of biodegradable magnesium-based interference screws in ACL reconstruction. Bioact Mater. 2021;6(10):3231–3243. doi:10.1016/j.bioactmat.2021.02.032
- Debnath S, Yallowitz AR, McCormick J, et al. Discovery of a periosteal stem cell mediating intramembranous bone formation. Nature. 2018;562(7725):133–139. doi:10.1038/s41586-018-0554-8
- Wang L, Tower RJ, Chandra A, et al. Periosteal mesenchymal progenitor dysfunction and extraskeletally-derived fibrosis contribute to atrophic fracture nonunion. J Bone Miner Res. 2019;34(3):520–532. doi:10.1002/jbmr.3626
- Daentzer D, Willbold E, Kalla K, et al. Bioabsorbable interbody magnesium-polymer cage: degradation kinetics, biomechanical stiffness, and histological findings from an ovine cervical spine fusion model. Spine. 2014;39(20):E1220–E1227. doi:10.1097/BRS.0000000000000507
- Xu H, Zhang F, Wang H, et al. Evaluation of a porous bioabsorbable interbody Mg-Zn alloy cage in a goat cervical spine model. BioMed Res Int. 2018;2018:7961509.
- Zhang F, Xu H, Wang H, et al. Quantitative analysis of near-implant magnesium accumulation for a Si-containing coated AZ31 cage from a goat cervical spine fusion model. BMC Musculoskelet Disord. 2018;19(1):105. doi:10.1186/s12891-018-2027-5
- Lin X, Ge J, Wei D, et al. Surface degradation-enabled osseointegrative, angiogenic and antiinfective properties of magnesium-modified acrylic bone cement. J Orthop Translat. 2019;17:121–132. doi:10.1016/j.jot.2019.04.007
- Lin X, Chan A, Tan X, et al. Fabrication and characterizations of metallic Mg containing PMMA-based partially degradable composite bone cements. Acta Metall Sin (Engl Lett). 2019;32(7):808–816. doi:10.1007/s40195-018-0841-2
- Windhagen H, Radtke K, Weizbauer A, et al. Biodegradable magnesium-based screw clinically equivalent to titanium screw in hallux valgus surgery: short term results of the first prospective, randomized, controlled clinical pilot study. Biomed Eng Online. 2013;12(1):62. doi:10.1186/1475-925X-12-62
- Plaass C, Ettinger S, Sonnow L, et al. Early results using a biodegradable magnesium screw for modified chevron osteotomies. J Orthop Res. 2016;34(12):2207–2214. doi:10.1002/jor.23241
- Plaass C, von Falck C, Ettinger S, et al. Bioabsorbable magnesium versus standard titanium compression screws for fixation of distal metatarsal osteotomies – 3 year results of a randomized clinical trial. J Orthop Sci. 2018;23(2):321–327. doi:10.1016/j.jos.2017.11.005
- Biber R, Pauser J, Brem M, et al. Bioabsorbable metal screws in traumatology: A promising innovation. Trauma Case Reports. 2017;8:11–15. doi:10.1016/j.tcr.2017.01.012
- Kose O, Turan A, Unal M, et al. Fixation of medial malleolar fractures with magnesium bioabsorbable headless compression screws: short-term clinical and radiological outcomes in eleven patients. Arch Orthop Trauma Surg. 2018;138(8):1069–1075. doi:10.1007/s00402-018-2941-x
- Gigante A, Setaro N, Rotini M, et al. Intercondylar eminence fracture treated by resorbable magnesium screws osteosynthesis: A case series. Injury. 2018;49:S48–S53. doi:10.1016/j.injury.2018.09.055
- Acar B, Kose O, Unal M, et al. Comparison of magnesium versus titanium screw fixation for biplane chevron medial malleolar osteotomy in the treatment of osteochondral lesions of the talus. Eur J Orthop Surg Traumatol. 2020;30(1):163–173. doi:10.1007/s00590-019-02524-1
- Leonhardt H, Ziegler A, Lauer G, et al. Osteosynthesis of the mandibular condyle with magnesium-based biodegradable headless compression screws show good clinical results during a 1-year follow-up period. J Oral Maxillofac Surg. 2020;79:637–643.
- Chen L, Lin Z, Wang M, et al. Treatment of trauma-induced femoral head necrosis with biodegradable pure Mg screw-fixed pedicle iliac bone flap. J Orthop Translat. 2019;17:133–137. doi:10.1016/j.jot.2019.01.004
- Erne P, Schier M, Resink TJ. The road to bioabsorbable stents: reaching clinical reality? Cardiovasc Intervent Radiol. 2006;29(1):11–16. doi:10.1007/s00270-004-0341-9
- Mario CD, Griffiths H, Goktekin O, et al. Drug-Eluting bioabsorbable magnesium stent. J Interv Cardiol. 2004;17(6):391–395. doi:10.1111/j.1540-8183.2004.04081.x
- Zhang J, Li H, Wang W, et al. The degradation and transport mechanism of a Mg-Nd-Zn-Zr stent in rabbit common carotid artery: a 20-month study. Acta Biomater. 2018;69:372–384. doi:10.1016/j.actbio.2018.01.018
- Chen C, Chen J, Wu W, et al. In vivo and in vitro evaluation of a biodegradable magnesium vascular stent designed by shape optimization strategy. Biomaterials. 2019;221:119414. doi:10.1016/j.biomaterials.2019.119414
- Zartner P, Cesnjevar R, Singer H, et al. First successful implantation of a biodegradable metal stent into the left pulmonary artery of a preterm baby. Catheter Cardiovasc Interv. 2005;66(4):590–594. doi:10.1002/ccd.20520
- Erbel R, Mario CD, Bartunek J, et al. Temporary scaffolding of coronary arteries with bioabsorbable magnesium stents: a prospective, non-randomised multicentre trial. Lancet. 2007;369(9576):1869–1875. doi:10.1016/S0140-6736(07)60853-8
- Waksman R, Erbel R, Mario CD, et al. Early- and long-term intravascular ultrasound and angiographic findings after bioabsorbable magnesium stent implantation in human coronary arteries. JACC Cardiovasc Interv. 2009;2(4):312–320. doi:10.1016/j.jcin.2008.09.015
- Haude M, Erbel R, Erne P, et al. Safety and performance of the drug-eluting absorbable metal scaffold (DREAMS) in patients with de-novo coronary lesions: 12 month results of the prospective, multicentre, first-in-man BIOSOLVE-I trial. Lancet (Lond Engl). 2013;381(9869):836–844. doi:10.1016/S0140-6736(12)61765-6
- Haude M, Ince H, Abizaid A, et al. Safety and performance of the second-generation drug-eluting absorbable metal scaffold in patients with de-novo coronary artery lesions (BIOSOLVE-II): 6 month results of a prospective, multicentre, non-randomised, first-in-man trial. The Lancet. 2016;387(10013):31–39. doi:10.1016/S0140-6736(15)00447-X
- Laubrie JD, Mousavi JS, Avril S. A new finite-element shell model for arterial growth and remodeling after stent implantation. Int J Numer Method Biomed Eng. 2020;36(1):e3282. doi:10.1002/cnm.3282
- Cheng J, Zhang LT. Simulation of vessel tissue remodeling with residual stress: an application to in-stent restenosis. Int J Smart Nano Mater. 2019;10(1):11–27. doi:10.1080/19475411.2018.1529002
- Boland EL, Grogan JA, McHugh PE. Computational modelling of magnesium stent mechanical performance in a remodelling artery: effects of multiple remodelling stimuli. Int J Numer Method Biomed Eng. 2019;35(10):e3247. doi:10.1002/cnm.3247
- Yoshida T, Fukumoto T, Urade T, et al. Development of a new biodegradable operative clip made of a magnesium alloy: evaluation of its safety and tolerability for canine cholecystectomy. Surgery. 2017;161(6):1553–1560. doi:10.1016/j.surg.2016.12.023
- Bai H, He X, Ding P, et al. Fabrication, microstructure, and properties of a biodegradable Mg-Zn-Ca clip. J Biomed Mater Res Part B. 2019;107(5):1741–1749. doi:10.1002/jbm.b.34267
- Chang YH, Tseng CC, Chao CY, et al. Mg-Zn-Ca alloys for hemostasis clips for vessel ligation: in vitro and in vivo studies of their degradation and response. Materials. 2020;13(13):3039. doi:10.3390/ma13133039
- Yu X, Li D, Liu Y, et al. In vitro and in vivo studies on the degradation and biosafety of Mg-Zn-Ca-Y alloy hemostatic clip with the carotid artery of SD rat model. Mater Sci Eng: C. 2020;115:111093. doi:10.1016/j.msec.2020.111093
- Qu S, Xia J, Yan J, et al. In vivo and in vitro assessment of the biocompatibility and degradation of high-purity Mg anastomotic staples. J Biomater Appl. 2017;31(8):1203–1214. doi:10.1177/0885328217692948
- Huang Q, Liu L, Wu H, et al. The design, development, and in vivo performance of intestinal anastomosis ring fabricated by magnesium-zinc-strontium alloy. Mater Sci Eng: C. 2020;106:110158. doi:10.1016/j.msec.2019.110158
- Wu H, Zhao C, Ni J, et al. Research of a novel biodegradable surgical staple made of high purity magnesium. Bioactive Mater. 2016;1(2):122–126. doi:10.1016/j.bioactmat.2016.09.005
- Amano H, Hanada K, Hinoki A, et al. Biodegradable surgical staple composed of magnesium alloy. Sci Rep. 2019;9(1):14671. doi:10.1038/s41598-019-51123-x
- Wang X, Ni J, Cao N, et al. In vivo evaluation of Mg–6Zn and titanium alloys on collagen metabolism in the healing of intestinal anastomosis. Sci Rep. 2017;7(1):44919. doi:10.1038/srep44919
- Xia J, Chen H, Yan J, et al. High-Purity magnesium staples suppress inflammatory response in rectal anastomoses. ACS Appl Mater Interfaces. 2017;9(11):9506–9515. doi:10.1021/acsami.7b00813
- Liu L, Li N, Lei T, et al. The in vitro biological properties of Mg-Zn-Sr alloy and superiority for preparation of biodegradable intestinal anastomosis rings. Med Sci Monit. 2014;20:1056–1066. doi:10.12659/MSM.890638
- Lu Q, Lin X, Yang L. Animal models for bone tissue Engineering and osteoinductive Biomaterial research. In: B Li, TF Moriarty, T Webster, M Xing, editor. Racing for the surface: Antimicrobial and interface tissue Engineering. Cham: Springer International Publishing; 2020. p. 245–288.
- Li Y, Jahr H, Zhou J, et al. Additively manufactured biodegradable porous metals. Acta Biomater. 2020;115:29–50. doi:10.1016/j.actbio.2020.08.018
- Wang Y, Fu P, Wang N, et al. Challenges and solutions for the additive manufacturing of biodegradable magnesium implants. Engineering. 2020;6:1267–1275. doi:10.1016/j.eng.2020.02.015
- Wang W, Jia G, Wang Q, et al. The in vitro and in vivo biological effects and osteogenic activity of novel biodegradable porous Mg alloy scaffolds. Mater Des. 2020;189:108514. doi:10.1016/j.matdes.2020.108514
- Grau M, Seiler C, Roland L, et al. Osteointegration of porous poly-epsilon-caprolactone-coated and previtalised magnesium implants in critically sized calvarial bone defects in the mouse model. Materials. 2017;11(1):6. doi:10.3390/ma11010006
- Wu Y, Wang YM, Zhao DW, et al. In vivo study of microarc oxidation coated Mg alloy as a substitute for bone defect repairing: degradation behavior, mechanical properties, and bone response. Colloids Surf B Biointerfaces. 2019;181:349–359. doi:10.1016/j.colsurfb.2019.05.052
- Wang W, Nune KC, Tan L, et al. Bone regeneration of hollow tubular magnesium-strontium scaffolds in critical-size segmental defects: effect of surface coatings. Mater Sci Eng: C. 2019;100:297–307. doi:10.1016/j.msec.2019.02.067
- Kang M-H, Lee H, Jang T-S, et al. Biomimetic porous Mg with tunable mechanical properties and biodegradation rates for bone regeneration. Acta Biomater. 2019;84:453–467. doi:10.1016/j.actbio.2018.11.045
- Lu XZ, Lai CP, Chan LC. Novel design of a coral-like open-cell porous degradable magnesium implant for orthopaedic application. Mater Des. 2020;188:108474. doi:10.1016/j.matdes.2020.108474
- Tang Y, Lin S, Yin S, et al. In situ gas foaming based on magnesium particle degradation: A novel approach to fabricate injectable macroporous hydrogels. Biomaterials. 2020;232:119727. doi:10.1016/j.biomaterials.2019.119727
- Yuan Z, Wei P, Huang Y, et al. Injectable PLGA microspheres with tunable magnesium ion release for promoting bone regeneration. Acta Biomater. 2019;85:294–309. doi:10.1016/j.actbio.2018.12.017
- Yu W, Li R, Long J, et al. Use of a three-dimensional printed polylactide-coglycolide/tricalcium phosphate composite scaffold incorporating magnesium powder to enhance bone defect repair in rabbits. J Orthop Translat. 2019;16:62–70. doi:10.1016/j.jot.2018.07.007
- Zheng L-Z, Wang J-L, Xu J-K, et al. Magnesium and vitamin C supplementation attenuates steroid-associated osteonecrosis in a rat model. Biomaterials. 2020;238:119828. doi:10.1016/j.biomaterials.2020.119828
- Noviana D, Paramitha D, Ulum MF, et al. The effect of hydrogen gas evolution of magnesium implant on the postimplantation mortality of rats. J Orthop Translat. 2016;5:9–15. doi:10.1016/j.jot.2015.08.003
- Tie D, Guan R, Liu H, et al. An in vivo study on the metabolism and osteogenic activity of bioabsorbable Mg–1Sr alloy. Acta Biomater. 2016;29:455–467. doi:10.1016/j.actbio.2015.11.014
- Seitz JM, Eifler R, Bach FW, et al. Magnesium degradation products: effects on tissue and human metabolism. J Biomed Mater Res A. 2014;102(10):3744–3753. doi:10.1002/jbm.a.35023
- Zhao D, Wang T, Kuhlmann J, et al. In vivo monitoring the biodegradation of magnesium alloys with an electrochemical H2 sensor. Acta Biomater. 2016;36:361–368. doi:10.1016/j.actbio.2016.03.039
- Kuhlmann J, Bartsch I, Willbold E, et al. Fast escape of hydrogen from gas cavities around corroding magnesium implants. Acta Biomater. 2013;9(10):8714–8721. doi:10.1016/j.actbio.2012.10.008
- Draxler J, Martinelli E, Weinberg AM, et al. The potential of isotopically enriched magnesium to study bone implant degradation in vivo. Acta Biomater. 2017;51:526–536. doi:10.1016/j.actbio.2017.01.054
- Zhao D, Wang T, Nahan K, et al. In vivo characterization of magnesium alloy biodegradation using electrochemical H2 monitoring, ICP-MS, and XPS. Acta Biomater. 2017;50:556–565. doi:10.1016/j.actbio.2017.01.024
- Waizy H, Diekmann J, Weizbauer A, et al. In vivo study of a biodegradable orthopedic screw (MgYREZr-alloy) in a rabbit model for up to 12 months. J Biomater Appl. 2014;28(5):667–675. doi:10.1177/0885328212472215
- Xi Z, Wu Y, Xiang S, et al. Corrosion resistance and biocompatibility assessment of a biodegradable hydrothermal-coated Mg–Zn–Ca alloy: an in vitro and in vivo study. ACS Omega. 2020;5(9):4548–4557. doi:10.1021/acsomega.9b03889
- Liu C, Wang J, Gao C, et al. Enhanced osteoinductivity and corrosion resistance of dopamine/gelatin/rhBMP-2–coated β-TCP/Mg-Zn orthopedic implants: An in vitro and in vivo study. PLoS One. 2020;15(1):e0228247.
- Wang J, Xu J, Liu W, et al. Biodegradable magnesium (Mg) implantation does Not impose related metabolic disorders in rats with chronic renal failure. Sci Rep. 2016;6(1):26341. doi:10.1038/srep26341
- Bodelon OG, Iglesias C, Garrido J, et al. Analysis of metallic traces from the biodegradation of endomedullary AZ31 alloy temporary implants in rat organs after long implantation times. Biomed Mater. 2015;10(4):045015. doi:10.1088/1748-6041/10/4/045015
- Kim Y-K, Kim S-Y, Lee SH, et al. Stabilized loading of hyaluronic acid-containing Hydrogels into magnesium-based cannulated screws. ACS Biomater Sci Eng. 2020;6(1):715–726. doi:10.1021/acsbiomaterials.9b01057