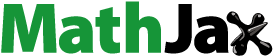
ABSTRACT
Bioceramics are in high demand due to their biocompatibility and bone-regenerative properties, representing a multibillion-dollar industry with orthopaedic and dental implant applications. However, traditional manufacturing methods have limitations in producing complex geometries tailored to match patient-specific bone defects. Vat-photopolymerization 3D printing has emerged as a precise and high-resolution technique to fabricate complex bioceramic parts, generating strong, ultralight, energy-absorbing, and tough materials. Despite their promise, the clinical translation of 3D-printed bioceramic implants is hampered by regulatory and reimbursement hurdles. This review analyses recent advances in vat-photopolymerization printing of bioceramics, highlighting the technical challenges and the potential of nanoscale printing to enhance the mechanical and biological functions of implants. The review also provides recommendations for regulatory frameworks, envisioning a future with the successful clinical translation of advanced 3D architectures.
A brief history of additive manufacturing of bioceramics
The increasing prevalence of bone loss due to trauma, osteoporosis, resection of infected or malignant bone, as well as issues associated with aseptic loosening of orthopaedic implants, has put a massive burden on public healthcare systems around the world [Citation1]. In this context, demand for synthetic substitutes for bone is growing rapidly. Ideal materials for synthetic bone are bioceramics, due to their remarkable bone-forming ability and biocompatibility [Citation2]. However, they are also inherently rigid and brittle, which has made it challenging to shape and process them in intricate and complex geometries, in both porous and nonporous forms [Citation3–5]. Conventional shaping methods such as dry pressing, injection moulding, salt leaching, gas foaming, and foam replication are incapable of producing highly complex ceramic structures with detailed features, as well as the required moulds and templates for the shaping of ceramics, and are often prohibitively expensive for use in iterative design optimizations [Citation6–8].
A few decades ago, additive manufacturing began to offer ways to overcome the disadvantages of conventional methods of fabricating highly complex bioceramic components [Citation5,Citation6]. Additive manufacturing, also known as three-dimensional (3D) printing, essentially involves the successive addition of two-dimensional (2D) layers of a starting material, with each new layer printed on top of the previous layer until the entire 3D construct is built [Citation9]. Historically, the additive manufacturing of bioceramic materials has come to fruition much later than that of polymeric and metallic materials. Whereas polymer and metal components can be formed in a single processing step using one machine, ceramic components are difficult to process and require multiple post-processing steps. In fact, most bioceramic additive manufacturing techniques are indirect: they initially produce a green body – a composite of ceramic powders and organic binders – and only after repeated cycles of heat treatment to remove the binder and solidify the powder is the final component produced.
According to ASTM/ISO standardization [Citation10], there are three main categories of additive manufacturing technologies for bioceramic materials: selective laser sintering (SLS), extrusion-based (EB) techniques, and vat-photopolymerization. Of these, SLS and EB are the most frequently cited (search for ‘3D printing AND bioceramics’ in Scopus).
SLS has been used to fabricate a range of bioceramic compositions, including calcium phosphates and calcium silicates [Citation11,Citation12]. In this process, a bed of powder is exposed to a laser beam, and the high temperature resulting from the interaction between the laser beam and the particles sinters a layer of the material [Citation13]. However, SLS has limitations: only bioceramics that do not decompose at high temperatures are suitable for use; and components typically end up with rough surfaces, and with insufficient sintering densities because particles may only fuse partially.
EB techniques such as robocasting [Citation14] involve the extrusion, from a nozzle, of a paste containing ceramic particles and organic binders. Dimensional resolution is restricted, however, by the nozzle’s diameter, making it impossible to print components with features less than 200 μm in size or with geometries that incorporate intricate overhangs.
Another EB technique is 3DP (binder jetting), which is popular for printing calcium phosphates. In this process a roller applies ceramic powder, at a predefined thickness, to a build platform, and a printhead then sprays a binder solution onto a specific region of the powder bed [Citation15]. This stimulates a reaction that binds the particles of powder together, forming a green body.
The accuracy of 3DP is impacted by three key factors: the flowability of the powder, the duration of the curing reaction and the wettability of the binder [Citation15]. High fluidity allows for the formation of a thinner and more homogeneous powder layer, resulting in more precisely printed constructs. Powders with small and homogeneous particle sizes can be used to create a 3D-printed construct with high-resolution; however, often the strong interparticle cohesion and the uneven morphology of small particles may lead to particle agglomeration, resulting in defective prints [Citation5]. The curing reaction between binders and powders needs to be quick in order to prevent the binder from flowing into the non-printing area, while also maintaining a viscosity that allows the next layer of powder to adhere. If the binder is too wet, its dispersion can be unpredictable, lowering printing accuracy; if it is not wet enough, it can result in insufficient adhesion between printed layers, with the result that the component has low mechanical strength [Citation5,Citation15].
Building on the described drawbacks of SLS and EB techniques, vat-photopolymerization has emerged as the most potent 3D-printing technology for producing bioceramic constructs with high dimensional accuracy and resolution. Vat-photopolymerization, often known as stereolithography, is a technique in which a photocurable resin is polymerized by a light source (laser) in a layer-by-layer manner. This process enables freeform design and production of complex shapes and geometries, including porous, semi-porous, and graded structures [Citation16]. The high degree of control it enables over internal architecture, shape and geometry makes it possible to finely tune the mechanical and biological properties of a bioceramic implant, which can be used to improve its osseointegration (bonding of bioceramic to bone), osteoconduction (underpinning support for new bone tissue) and osteoinduction (promotion of new bone tissue formation) [Citation17–23].
Although bioceramics have some obvious advantages (e.g. unlimited supply, exceptional bone bioactivity and biocompatibility in vivo), as yet they are unable to regenerate bone as successfully as bone grafts can, and often cannot be used in load-bearing applications. Consequently, the autologous bone graft remains the gold standard in clinical settings. However, once certain challenges are overcome (discussed in the next section), vat-photopolymerization could shift this paradigm. Vat-photopolymerization, via complex topological optimizations, could be used to manufacture bioceramic implants that are strong, tough and bioactive and that satisfy clinical requirements.
Current challenges in vat-photopolymerization of bioceramics
Currently, vat-photopolymerization of bioceramics faces multiple challenges [Citation24,Citation25]: (1) the speed of fabrication is slow, compared to industrial manufacturing techniques such as injection moulding; (2) the printing resolution is limited to micron-scale because the polymerizing laser light is scattered by solid particles; (3) the surface finish of components is inferior, compared to those manufactured by injection moulding and (4) there are difficulties in formulating an appropriate resin-ceramic composite with optimum properties for mutually exclusive variables such as curing depth, solid-loading and viscosity. For example, high solid-loading (i.e. increasing the particle content) of the composite resin is desirable because it reduces the volumetric shrinkage of the component during sintering, where excessive shrinkage can lead to structural warping and deformation; yet increasing the particle content also increases the viscosity of the resin and reduces the curing depth, which compromises the ability to print intricate features.
These challenges have fuelled research to advance vat-photopolymerization techniques for bioceramics, leading to an exponential rise in the number of peer-reviewed articles on the topic since 2019. This review paper discusses recent advances in vat-photopolymerization to increase the speed and resolution of printing, and elaborates on key variables in the preparation of the resin-ceramic composite and in the post-processing steps to obtain a sound print.
Vat-photopolymerization of bioceramics
Types of techniques
Scanning stereolithography (SSLA)
There are three types of vat-photopolymerization: laser scanning stereolithography (SSLA), mask image projection stereolithography, and two-photon polymerization (TPP) [Citation26–28]. In SSLA, a galvanometer is used to steer a laser over the surface of the resin to cure it. The laser can be directed to the resin surface from the bottom up ((a)) or the top down ((b)).
Figure 1. Schematic of commonly used vat-photopolymerization techniques for 3D printing of bioceramics. (a) bottom-up and (b) top-down laser scanning stereolithography and (c) mask image projection stereolithography [Citation25].
![Figure 1. Schematic of commonly used vat-photopolymerization techniques for 3D printing of bioceramics. (a) bottom-up and (b) top-down laser scanning stereolithography and (c) mask image projection stereolithography [Citation25].](/cms/asset/ea77d752-914e-45b4-9bba-fcbbcbe3d530/yimr_a_2194744_f0001_oc.jpg)
Bottom-up SSLA is the oldest and most common technique of vat-photopolymerization for printing bioceramics ((a)). In this technique, the ceramic resin is contained in a vat that is transparent to UV light; the vat is also coated with a non-stick material so that the printed layers will adhere to the building platform instead of the vat [Citation25]. The first layer is built by moving the building plate down into the resin until an appropriate resin layer thickness is reached between the plate and the base of the vat. The resin is then irradiated with a UV laser that scans the entire layer, voxel by voxel, tracing the required geometry. Once the layer is cured, the building plate moves upward, moving the cured first layer with it away from the base of the vat. These steps are repeated layer by layer until the component is completed.
Bottom-up SSLA offers several advantages over top-down SSLA: (1) the printed layer is lifted out of the resin rather than submerged in it, therefore only a minimal amount of low viscosity resin is required; (2) the irradiated layer is not exposed to the air, thus oxygen inhibition is minimal and (3) the height of the printed object is not restricted by an overhead scanner, therefore less post-processing is required to remove uncured resins.
illustrates one of the first examples in which SSLA (using a top-down approach) was used to manufacture a patient-specific hydroxyapatite implant for the reconstruction of a large and complex craniofacial bone defect [Citation29]. The implant’s shape was derived directly from a scanned image of the patient’s skull, and implants were produced in 2–3 weeks without machining or moulding, with a dimensional accuracy of 400 μm [Citation29]. However, in this study, the size and shape of the implant was not perfectly adapted to the bone defect. This was particularly the case with the edges of the implant, which were not thin enough to overlap the surrounding living bone. The authors concluded that this method of manufacture does not produce the requisite three-dimensional shape ((c)).
Figure 2. An example of scanning stereolithography used to print a patient-specific bioceramic implant. (a) Implant designed with the aid of CAD software using scan of patient skull. (b) 3D-printed hydroxyapatite implant with macroporous regions to promote osseointegration. (c) Intraoperative view of the implant in the defect region, also depicting how the edges of the implant are not thin enough to completely overlap the surrounding bone [Citation29].
![Figure 2. An example of scanning stereolithography used to print a patient-specific bioceramic implant. (a) Implant designed with the aid of CAD software using scan of patient skull. (b) 3D-printed hydroxyapatite implant with macroporous regions to promote osseointegration. (c) Intraoperative view of the implant in the defect region, also depicting how the edges of the implant are not thin enough to completely overlap the surrounding bone [Citation29].](/cms/asset/04e3068c-4858-43ec-ba41-68aac75ab583/yimr_a_2194744_f0002_oc.jpg)
Mask image projection stereolithography: digital light processing and liquid crystal display
In mask image projection stereolithography, instead of scanning the resin surface layer by layer with a laser spot, each layer is cured in one exposure by projecting onto the surface a picture that depicts the component’s 2D cross-section ((c)). The two main variants of mask image projection stereolithography used for printing bioceramics are digital light processing (DLP) and liquid crystal display (LCD) techniques.
DLP is the latest evolution of SSLA and relies on a UV projector with a dynamic mask system to polymerize a complete 2D section of ceramic resin per exposure cycle. In this approach, a digital micromirror device acts as a dynamic mask by selectively enabling or disabling single mirrors in an array of several million mirrors ((c)). Production times with DLP are dramatically shortened compared to SSLA since a complete layer of resin can be cured at the same time by projecting a 2D pixel pattern onto it, rather than using a voxel-by-voxel approach [Citation6]. This means the build time is determined solely by the component’s height, the layer’s thickness, and the exposure time, and is independent of the shape, the size (of the x–y plane) and the complexity of the printed component. This is especially critical when using partially stable ceramic resins with short processing times. It also needs less complicated technology, because accurate control is only required for the z-plane [Citation6]. These features of the DLP technique make it highly advantageous for mass production, with ensuing economic benefits.
Due to its fast layer-setting and high precision, many researchers are shifting toward DLP technology for printing bioceramics for bone tissue engineering. shows the level of accuracy and architectural complexity that can be achieved using DLP: from a wollastonite diopside glass bioceramic, Schmidt et al [Citation30] managed to print kelvin structures with tetrakaidecahedron unit cells; and they observed that all the features of the printed sample, including the presence of discrete layers in the z-direction, were completely transferred to the sintered sample. The images confirm that the uniform and isotropic shrinkage led to no deformation of the structures after heat treatment.
Figure 3. Kelvin-structured constructs made from wollastonite diopside glass bioceramic and printed using the DLP technique, depicting its ability to produce intricate structures. as printed (a, b) and sintered (c, d) [Citation30].
![Figure 3. Kelvin-structured constructs made from wollastonite diopside glass bioceramic and printed using the DLP technique, depicting its ability to produce intricate structures. as printed (a, b) and sintered (c, d) [Citation30].](/cms/asset/5e4a807f-c103-40c1-805f-d58d1bff1fb6/yimr_a_2194744_f0003_ob.jpg)
In a recent report, Baino et al [Citation31] demonstrated the capacity of DLP to print hydroxyapatite constructs with an open-cell foam structure similar to the trabecular architecture of cancellous bone; they used a tomographic reconstruction of an open-cell polymeric foam as an input file to the DLP-based stereolithographic system (). Such porous structures were produced previously using the traditional technique of foam replication, but this lacks control over the size and shape of the struts, and generates mechanically weak scaffolds. DLP allows a high level of control over pore and strut characteristics, and has good reproducibility, generating porous constructs with higher mechanical strength than the conventional foam replication technique.
Figure 4. The first report of DLP technology being used to generate hydroxyapatite constructs with open-cell foam structure. (a) Cubic sponge, (b) double cubic sponge and (c) cylindrical sponge structures generated using a computer-aided design file created from micro-computed tomography of a commercial polyurethane sponge (45 pores per inch). (d and e) Printed structures after removing the uncured resin, and (f) sintered scaffolds with sponge architecture [Citation31].
![Figure 4. The first report of DLP technology being used to generate hydroxyapatite constructs with open-cell foam structure. (a) Cubic sponge, (b) double cubic sponge and (c) cylindrical sponge structures generated using a computer-aided design file created from micro-computed tomography of a commercial polyurethane sponge (45 pores per inch). (d and e) Printed structures after removing the uncured resin, and (f) sintered scaffolds with sponge architecture [Citation31].](/cms/asset/ee7196dd-af8b-438e-8be8-1e2b1f5c1274/yimr_a_2194744_f0004_oc.jpg)
Liquid crystal display, the latest and more affordable technique, is similar to SSLA and DLP except that it uses a different imaging system. This technique uses a liquid crystal display to irradiate the resin layer with UV, which results in monomer polymerization. Since the resolution (pixel density) of existing LCDs is low compared to that of the digital micromirror device used in DLP, the LCD approach has yet to be adopted for printing bioceramics. But the development of next-generation high-resolution LCDs could evolve this technology into a game-changer for bioceramic printing.
Two-photon polymerization (TPP): the future of high-resolution, defect-tolerant bioceramics
In TPP, photopolymerization of a photosensitive resin occurs through the absorption of two photons of light with two different wavelengths. This means curing takes place within a highly localized focal point, enabling structures to be printed at a high resolution of <200 nm, which is impossible to achieve with single-photon photopolymerization techniques (SSLA, DLP and LCD). Although the TPP technique is predominantly used for printing polymeric materials and has yet to be realized for bioceramics, there have been some advances in adapting it to ceramic materials.
One strategy is to use preceramic precursor resins (also called preceramic polymers), which consist of polymeric chains containing non-metallic elements such as silicon [Citation32]. These resins are transparent to light, unlike manually made mixtures of inorganic particles and photosensitive resins that substantially scatter the laser. The cured preceramic polymers can be transformed into ceramic compounds by pyrolysis under the correct conditions, usually without oxygen. However, this method has significant hurdles to overcome, such as excessive shrinkage during heat treatment, and partial ceramization due to pyrolysis temperatures below the polymer-to-ceramic transformation completion temperature.
Another strategy is templating. First, a polymer structure is printed by TPP. Then its surface is coated with a thin layer of ceramic, using deposition technologies such as chemical vapour deposition and atomic layer deposition (ALD). Finally, the polymer core is removed, using a chemical etching process, leaving a hollow ceramic nanolattice. This technique has been used by Bauer et al [Citation33] to fabricate delicate alumina (Al2O3) cellular microarchitectures with exceptional mechanical and physical properties (). TPP-printed micro-lattice polymeric templates were coated with Al2O3 layers of different thicknesses (ranging from 5 to 60 nm) using atomic layer deposition, leading to different relative densities and yield strengths [Citation33]. Then the polymer was etched away in O2 plasma, yielding a micro-ceramic lattice composed of hollow ceramic tubes ((a)). The microstructures were mechanically tested in compression ((b)) [Citation33]. As shown, the printed bar of the truss measures only ∼1 µm. The trusses reached a compressive strength of 55 MPa for a density of ∼0.42 g cm−3, while the optimized honeycomb exhibits 280 MPa for a density of ∼0.81 g cm−3. This impressive compressive strength exceeds all natural and engineered materials with a density below 1 g cm−3 (density of water) ((c,d)) [Citation33].
Figure 5. (a) High-resolution cellular alumina constructs printed using the TPP technique and atomic layer deposition of the ceramic coating (scale bars. 10 μm). All ceramic constructs were tested by uniaxial compressive loading. (b) An in-situ test of a polymeric truss structure (side view). (c) Stress–strain curves of fully triangular trusses. With increasing layer thickness, the compressive strength and stiffness (Young’s modulus) increase, compared with uncoated polymeric structures. (d) Specific compressive strength as a function of the coating thickness [Citation33].
![Figure 5. (a) High-resolution cellular alumina constructs printed using the TPP technique and atomic layer deposition of the ceramic coating (scale bars. 10 μm). All ceramic constructs were tested by uniaxial compressive loading. (b) An in-situ test of a polymeric truss structure (side view). (c) Stress–strain curves of fully triangular trusses. With increasing layer thickness, the compressive strength and stiffness (Young’s modulus) increase, compared with uncoated polymeric structures. (d) Specific compressive strength as a function of the coating thickness [Citation33].](/cms/asset/de655111-6ea9-46fc-a720-d67befa36b78/yimr_a_2194744_f0005_oc.jpg)
The mechanical properties of ceramic materials are defect-dominated because they are brittle and have low damage tolerance. Addressing brittleness is one of the premises of transforming the nanoscale printing of ceramics. Meza et al [Citation34] created ultralight, hollow ceramic nanolattices that absorb energy, recover after significant compression, and reach an untapped strength and stiffness material property space ((a–c)). This was achieved using high-strength ALD alumina engineered into a thin-walled nanolattice that is capable of deforming elastically via shell buckling. These ultralight ceramic nanolattices represent the concept of materials by design, whereby a strong, dense, brittle ceramic is transformed into a strong, ultralight, energy-absorbing and recoverable metamaterial ((d–h)). This contrasted with the brittle behaviour of nanolattices with thick walls, demonstrating a linear elastic region followed by catastrophic brittle failure with no post-compression recovery ((i–m)).
Figure 6. Architecture, design, and microstructure of ductile alumina nanolattices. (a) Alumina octet-truss nanolattice. (b) Zoomed-in section of the alumina octet-truss nanolattice; the inset shows an isolated hollow tube. (c) TEM dark-field image with diffraction grating of the alumina nanolattice tube wall. (d to h) Mechanical data and still frames from the compression test on a thin-walled (10 nm) nanolattice demonstrating the slow, ductile-like deformation, local shell buckling, and recovery of the structure after compression. (i to m) Mechanical data and still frames from the compression test on a thick-walled (50 nm) nanolattice showing catastrophic brittle failure and no post-compression recovery [Citation34].
![Figure 6. Architecture, design, and microstructure of ductile alumina nanolattices. (a) Alumina octet-truss nanolattice. (b) Zoomed-in section of the alumina octet-truss nanolattice; the inset shows an isolated hollow tube. (c) TEM dark-field image with diffraction grating of the alumina nanolattice tube wall. (d to h) Mechanical data and still frames from the compression test on a thin-walled (10 nm) nanolattice demonstrating the slow, ductile-like deformation, local shell buckling, and recovery of the structure after compression. (i to m) Mechanical data and still frames from the compression test on a thick-walled (50 nm) nanolattice showing catastrophic brittle failure and no post-compression recovery [Citation34].](/cms/asset/9999d23e-dddc-4ea4-adb3-12620708220e/yimr_a_2194744_f0006_oc.jpg)
Until recently, it was widely assumed that incorporating ceramic particles into photopolymers would not work for TPP. However, Wen et al [Citation35] successfully formulated a high loading of PEG-functionalized colloidal silica nanoparticles mixed in a photosensitive resin, then used the TPP technique and then sintering to generate high-quality 3D silica structures with arbitrary shapes in amorphous glass or polycrystalline cristobalite at sub-200 nm resolution (). They found that in order to be suitable for TPP, the ceramic resin should meet several conditions: (1) the ceramic nanoparticles should be small, close to 10 nm; (2) the refractive index of the photopolymer resin should match that of the nanoparticles, to produce a transparent suspension that eliminates photo extinction and scattering; (3) the heat conductivity of the ceramic resin should be high enough to avoid instant vaporization by the femtosecond laser at megawatts of peak power; (4) the resin mixture should be very homogeneous and well dispersed, to maintain nanoscale resolution and to avoid localized vaporization and (5) the mass loading of the particles should be high enough to maintain the printed geometry and to minimize deformation. Wen et al [Citation35] also suggested that, while using smaller nanoparticles is necessary to achieve high resolution, it can result in a resin-ceramic mixture in which the viscosity is too high, which in turn makes mechanical mixing difficult. Furthermore, as viscosity is generally inversely related to heat conductivity, excessive viscosity can also result in low heat conductivity. Consequently, optimization of particle size is vital.
Figure 7. (a) Schematic of set-up and process for TPP-enabled 3D printing of silica nanoparticles. Upper right inset. photo of the as-synthesized polymer precursors, the colloidal silica nanoparticles and the as-synthesized nanocomposite ink. Bottom right inset. atomic structures of silica under different sintering temperatures. (b–e) SEM images of 3D-printed structures. face-centred cubics (fcc) lattice truss structure (b; scale bar 5 μm), diamond lattice truss structure (c; scale bar 10 μm), disc-on-truss structure (d; scale bar 10 μm), and needle array (e; scale bar 10 μm). (f) Zoomed-in top view of fcc lattice truss structure (scale bar 400 nm). (g) Zoomed-in top view of diamond lattice truss structure (scale bar 1 μm). (h) Zoomed-in top front view of disc-on-truss structure (scale bar 2.5 μm). (i) Zoomed-in top front view of needle array; tip radius 230 nm (scale bar 2.5 μm).

Types of resin: function and role of constituents
The main component in vat-photopolymerization techniques is a photosensitive resin, which typically contains one or more types of monomers (or oligomers). These polymerize to form highly cross-linked structures, photoinitiators that generate reactive initiating species upon exposure to UV, and dispersant molecules that improve the stability and homogeneity of ceramic particles in the resin. In SSLA and mask-based stereolithography techniques, resins mixed with ceramic particles turn into a solid ceramic–polymer composite mass within a few seconds of exposure to UV. This is due to the crosslinking of long-chain macromolecules through continuous reaction of small molecules (monomers) with reactive species generated by photoinitiators.
The rate and efficacy of crosslinking and curation are governed by several factors: the intensity and wavelength of the light (wavelength in the range of 200–700 nm), exposure time, type of monomer and photoinitiator, and ceramic content. Ideally, resins should contain 40–50 vol.-% of ceramic powder to avoid excessive shrinkage and structural deformation during the sintering cycle, which gives rise to a high-density component. Monomers, which are responsible for binding the ceramic particles during the photopolymerization process, form a large portion of the printed ceramic structures and should be removed thermally during sintering. Most monomers decompose at high temperatures (>300°C) and generate a large volume of gas when they burn. This suggests that resins should be tested by thermogravimetric analysis to understand their decomposition behaviour before mixing with ceramics.
A key factor in choosing a monomer for ceramic stereolithography is viscosity. The monomer should have low viscosity in order to achieve a ceramic-resin mixture with adequate flowability. Monomers can be categorized into aqueous (acrylamide-based) and non-aqueous (organic-based). Aqueous monomers have lower viscosity than non-aqueous, but they also possess a low refractive index. Since the difference in refractive index between ceramic particles and aqueous monomers is therefore high, these monomers are not favourable for printing bioceramics. Non-aqueous monomers with rheological properties suitable for photopolymerization-based additive manufacturing typically include acrylates, methacrylate epoxides, vinyl ethers, propenyl ethers, siloxanes, cyclic acetals, and furfural. Of these, acrylates (e.g. methylacrylate) with RH (R = aryl group or CH3) are the most widely used for 3D printing of ceramic components. These monomers have low viscosity and are highly reactive in the presence of a suitable photoinitiator under UV light. An alternative to monomers is oligomers, which consist of more structural and functional units. Despite this versatility, however, they have a higher viscosity than monomers and are therefore less applicable for use with ceramic particles.
The type of photoinitiator is also crucial because it directly influences the curing depth of the resin. Two types are used extensively in the preparation of ceramic resins: Type I and Type II, categorized according to what causes the monomers to polymerize. In Type I, the formation of benzoyl radicals is responsible for the polymerization of monomers, whereas in Type II it is the formation of aromatic ketones coupled with radicals of hydrogen donor (amines, ethers and alcohols). Common Type II photoinitiators are aromatic diketones, benzophenones, phenylglyoxalates, thioxanthones, methylphenylglyoxylates and camphorquinones.
The concentration of the photoinitiator, as well as the type, directly influences the curing depth of the resin. Typically, at low concentrations, the curing depth approaches zero and at excessively high concentrations, the penetration depth of photons decreases. In order to obtain an optimum curing depth, therefore, an intermediate concentration is required. In most cases, this is reported to be in the range of 0.3–1 wt-% with respect to the resin weight.
The other component in ceramic-resin mixtures is a dispersant. Dispersants are added to prevent the ceramic particles from agglomerating, and to help reducing the viscosity of resin. A specific dispersant formulation must be developed for each ceramic resin, catering to the type of monomer and the composition of the ceramic particles.
Fundamental parameters in vat-photopolymerization of bioceramics
The fundamental parameters in vat-photopolymerization can be classified into three groups: (1) parameters that can be adjusted by the device and controlled by the slicing software (e.g. layer thickness, laser power, and exposure time); (2) parameters that influence viscosity, light-scattering properties, and curing depth (e.g. particle shape, size, distribution, volume fraction, and composition; and dispersant type and concentration) and (3) postprocessing parameters such as debinding and sintering (e.g. temperature, holding time and ramp rate). Most of these variables are interrelated, and their impacts on ceramic resin characteristics are either synergistic or reductive.
Viscosity
Ideally, the viscosity of the ceramic resin used in ceramic vat-photopolymerization should be similar to that of the resin without ceramic particles (between 80 and 200 mPa.s). However, introducing ceramic particles significantly increases the viscosity of resin, therefore, this is practically impossible to be achieved. A resin containing 40–50 vol.-% ceramic particles should have a viscosity value of less than 3000 mPa.s. The ceramic resin should also possess shear-thinning properties for improved flow when shear forces are applied; this is an essential condition for the ceramic resin to be able to re-coat and self-level and, ultimately, to print complex geometries. On the other hand, there should be a high proportion of ceramic particles in the resin (in the range of 40–50 vol.-%), but this often substantially increases the viscosity. A high solid loading is particularly important for printing large and complex objects. While a low solid loading (25–35 vol.-%) may result in successful prints, despite high shrinkage, for smaller, symmetrical and less complex objects, a high solid loading mitigates excessive shrinkage during the sintering stage, as well as the risk of cracking and warping. However, it can cause inhomogeneity, and hence reduce the efficiency of the resin for polymerization. It is therefore necessary to apply a dispersant to regulate particle-particle interactions and maintain a low viscosity. The concentration of dispersants in relation to the mass of ceramic powder is usually between 1 and 3 wt-%. For each resin and ceramic powder, the type and concentration of dispersant should be thoroughly investigated before use.
Light scattering: curing depth and particle physico-chemical properties
Understanding the physics of the interaction between UV light and solid particles, which governs light scattering, is essential for a successful print. In a heterogeneous ceramic-resin composite, ceramic particles scatter the photon; increasing the number of particles per unit volume therefore results in more scattering in the resin (). On the other hand, photosensitive resins without inorganic particles are quite transparent to UV lasers, allowing the light to pass through and initiate the polymerization cascade.
Figure 8. A schematic depicting the interaction of light with ceramic particles suspended in a liquid resin [Citation25].
![Figure 8. A schematic depicting the interaction of light with ceramic particles suspended in a liquid resin [Citation25].](/cms/asset/c424f91c-156f-4f03-a6ee-d16339f0fb76/yimr_a_2194744_f0008_oc.jpg)
The energy of a laser beam has a Gaussian distribution, meaning that laser energy is maximum at its apex (Emax) and decays incrementally toward the edge, where it reaches a critical energy (Ec). Therefore, when the laser beam irradiates the resin, what is cured is a layer in the shape of a parabolic cylinder ((a)). In this cured layer, the maximum cured depth and width are known as curing depth (Cd) and curing width (Cw); the longitudinal and lateral resolutions of prints are significantly influenced by these two characteristics. The irradiated light has its Emax at the resin surface (at peak intensity of I0 at the beam centre), and its Ec at the bottom of Cd must be the least amount of energy required to initiate photopolymerization. Particles with a high refractive index scatter the laser into large angles of deflection. Due to this light-scattering effect, the inclusion of ceramic particles in resin reduces Cd and thus affects resolution ((b) and ) [Citation25,Citation36]. The incident light is also absorbed by the ceramic particles, further lowering the Cd. Since the typical thickness of the resin layer in single photon vat-photopolymerization of ceramics is between 10 and 100 μm, the Cd of the ceramic resin must be higher than this to facilitate printing.
Figure 9. (a) A model of laser line exposure to photosensitive resin, depicting an energy Gaussian distribution for the irradiated laser where Vs. scanning speed in x-direction, I0. peak intensity at the centre of beam where the laser’s energy is at its maximum value (Emax), W0. radius of laser, Cd. curing depth, Lw. line width, which equals curing width (Cw), Ec. critical energy, minimum laser energy required for curing the resin. (b) A schematic of the curing profile for two ceramic photocurable resins, one with low and the other with high refractive index (RI) contrast (Δn), subject to the same exposure [Citation25].
![Figure 9. (a) A model of laser line exposure to photosensitive resin, depicting an energy Gaussian distribution for the irradiated laser where Vs. scanning speed in x-direction, I0. peak intensity at the centre of beam where the laser’s energy is at its maximum value (Emax), W0. radius of laser, Cd. curing depth, Lw. line width, which equals curing width (Cw), Ec. critical energy, minimum laser energy required for curing the resin. (b) A schematic of the curing profile for two ceramic photocurable resins, one with low and the other with high refractive index (RI) contrast (Δn), subject to the same exposure [Citation25].](/cms/asset/fd0294ae-f69b-4a3d-ac14-b7f040345f14/yimr_a_2194744_f0009_oc.jpg)
Figure 10. The shape of the cured line in silica suspensions with varying refractive index values for monomer solution (E0 = energy dosage of laser). The schematic illustrates how a line of resin is cured, at different RI contrast values, by a square-wave beam from a slit propagated into the suspension through a glass slide. At low RI contrast, the line’s shape is similar to the square wave; as the RI contrast increases, the line broadens [Citation36].
![Figure 10. The shape of the cured line in silica suspensions with varying refractive index values for monomer solution (E0 = energy dosage of laser). The schematic illustrates how a line of resin is cured, at different RI contrast values, by a square-wave beam from a slit propagated into the suspension through a glass slide. At low RI contrast, the line’s shape is similar to the square wave; as the RI contrast increases, the line broadens [Citation36].](/cms/asset/b3ffe771-2fb0-45ca-acf2-b63fd4eea92b/yimr_a_2194744_f0010_oc.jpg)
The Cd is inversely proportional to the difference between the refractive indexes of the ceramic particles and the resin (Δn); a large difference results in severe scattering events and hence a shallow Cd. Due to the light-scattering effect of the particles, the cone-shaped profile of the cured area is broadened, while the penetration depth is decreased. Typically, ceramic resins with low Δn have greater Cd, and ones with high Δn have smaller Cd. For example, if a resin has a refractive index of 1.56, and if there are three ceramic materials with particle refractive indexes of A: 1.5, B: 1.7 and C: 2.1, then photopolymerization of ceramic-resin A will be facile, B possible, but C challenging, under the same printing conditions. A large mismatch between refractive indexes also results in particle coarsening, as well as structures with severe cracks, low mechanical properties and poor surface quality. Achieving a proper Cd is also important in promoting binding between layers via over-curing. Over-curing by approximately ∼20% of the layer thickness helps individual layers adhere to each other, preventing delamination. In this context, Cd should be equal to the over-cured thickness plus the layer thickness. In other words, over-curing must be ∼20% more than the layer thickness.
Another key factor in the vat-photopolymerization of ceramic resins is curing width (Cw), which affects the lateral resolution. Cw can be calculated using the following equation:
(1)
(1) In this equation, W0 is the laser radius and Dp is the penetration depth at which the laser’s intensity is reduced to ∼37% of its value at the resin’s surface. This equation demonstrates that curing width is directly proportional to the laser radius and the square root of the ratio of curing depth and penetration depth. This equation is significant because if these relationships are not met for a ceramic resin, the resin should be replaced.
A more accurate description of the curing process when ceramic particles are introduced into the resin can be obtained using the Kubelka–Munk model. This model takes into consideration the reflectance, transmittance and absorption caused by ceramic particles:
(2)
(2) In this equation, the value of radiation energy release (E) at a certain depth (z) inside the ceramic-resin mixture is correlated to Emax, scattering coefficient (S) which is proportional to 1/Dp and specific adsorption coefficient (K), which are dependent on the thickness and density of a cured layer. Thus, the reflectance term can be substituted by the reflective indexes of the photocuring liquid (n0) and the ceramic particles (np) where Δn = np–n0. The penetration depth of the cured layer can be calculated by the median size of the ceramic particles (D50), the wavelength of incoming light (λ), and the interparticle spacing (solid loading) (Φ):
(3)
(3) The equation shows that the penetration depth of the laser beam in a ceramic resin is inversely proportional to Δn2. In other words, a small change in resin and ceramic refractive indexes can result in a large effect on curing depth, potentially greater than that due to particle size and interparticle spacing, which are other factors that determine the penetration depth. Although particle size has a lesser effect on curing depth, it significantly influences slurry rheology and debinding steps, so must be carefully controlled. According to this equation, ceramic powders with a smaller median size (D50) result in higher scattering and lower penetration depth. Larger particles, on the other hand, transmit more light. In practice, both median particle size and the size distribution of ceramic powders affect laser scattering. Small particles substantially reduce the Cd, particularly when particle size approaches the wavelength of curing light. The same has been observed for powders with a broad particle size distribution. Some studies suggest that lowering the average particle size leads to a greater Cd, although this contradicts Equation (3) above [Citation37,Citation38]. However, interparticle distancing should be considered in such investigations. It is worth noting that the majority of those results were for a diluted suspension.
Overall, four main criteria should be satisfied when combining ceramic materials and photosensitive resins: (1) the difference between the refractive indexes of the ceramic particles and the resin should be small; (2) the ceramic particles should not absorb large quantities of UV; (3) the ceramic particles should be smaller than the thickness of one layer of resin and (4) the size of the ceramic particles should have a narrow distribution range.
Debinding
After the vat-photopolymerization process, the print is a green body of ceramic powders and organic binders. To transform this into the final construct requires two postprocessing steps: (1) removing residual resins from the print using compressed air and/or solvents (‘cleaning’) and (2) burning the print at a high temperature to remove organic components (‘debinding’) and densify the ceramic component (‘sintering’). Debinding is crucial since it directly affects the quality of the final construct, so is worth some discussion.
The main factors affecting the quality of the debinding process are: (1) resin chemistry (including monomer or oligomer, photoinitiator, dispersant and diluent); (2) composition of the ceramic; (3) ceramic particle size and size distribution; (4) ceramic particle loading and packing; (5) size of the print and thickness of its walls, and the extent of its structural intricacy and complexity and (6) heating rate and holding temperature. During the debinding process, several typical phenomena occur, as depicted in . These phenomena can be divided into four stages (temperatures are nominal; precise temperatures could be determined through a thermogravimetry study of ceramic-resin mixtures): (1) low temperature (∼room temperature to 200°C); (2) medium temperature (∼200°C to 300°C); (3) high temperature or pyrolysis (300°C to 600°C) and (4) sintering. In stage 1, some of the resin in the structure softens, and some of the resin on the surface starts decomposing. In stage 2, with the removal of resin on the surface, the gases generated from decomposing organic components travel from inside the green body to its surface, with the softening of the binder under the action of pressure after thermal expansion and capillary force between powders. As the volume of gases increases sharply, cascades of softening and produced gases push and rearrange the ceramic particles, creating channels in the green body. At this stage, if the heating rate or holding temperature is too high, not enough connecting channels are created. This generates excessive pressure, leading to the formation of cracks that are too large to be fused (repaired) during the sintering stage, which can result in the collapse of the entire construct. On the other hand, a proper debinding schedule in stage 2 ensures that remaining organic components are removed during stage 3. In this high-temperature debinding stage, organic residues are subjected to vigorous exothermic pyrolysis through reaction with oxygen-producing carbon dioxide. This rapidly expanding carbon dioxide not only exacerbates any cracks formed in stage 3, but may also generates new defects.
Figure 11. Schematic of the debinding process from the inner part of the green body to the surface [Citation39].
![Figure 11. Schematic of the debinding process from the inner part of the green body to the surface [Citation39].](/cms/asset/4f6d3733-68b5-4389-a110-ab66d73bc20c/yimr_a_2194744_f0011_oc.jpg)
The current state, challenges and future developments in vat-photopolymerization of bioceramics
The current state and challenges
Vat-photopolymerization of inert bioceramics
Utilizing vat-photopolymerization for the fabrication of inert and bioactive bioceramics is continuously increasing due to the growing demand to create implants with accurate and complex structures. Within the past few years, the fabrication of inert bioceramics using vat-photopolymerization has focused on dental applications, with the aim to replace the use of metallic implants [Citation40–44]. The main advantages of vat-photopolymerization, compared to other 3D printing techniques such as SLS, are the production of implants with higher dimensional accuracy and smoother surface finish, as required for dental implants [Citation44]. Various studies have attempted to formulate photosensitive slurries using inert bioceramics to obtain high-density and mechanically strong solid implants. However, these were constrained to alumina (Al2O3), and yttria-stabilized zirconia (ZrO2), due to their high chemical stability (corrosion resistance) and mechanical properties; both key requirements for dental implants [Citation40–44]. The challenge in applying vat-photopolymerization technology to inert bioceramics (Al2O3 and ZrO2) remains in the need to develop photosensitive slurry formulations that deliver high density implant structures; necessary to fabricate strong inert bioceramics.
Vat-photopolymerization of bioactive bioceramics
Advanced design strategies: Bioactive implants for bone regeneration should be highly porous and mechanically stable to facilitate implant integration with bone structures. Vat-photopolymerization techniques have opened an unprecedented opportunity in fabricating porous structures with advanced designs and high-level of complexity to enhance mechanical properties and bioactivity. Recently, vat-photopolymerization techniques, particularly DLP, have been used to fabricate porous bioactive bioceramics implants with significantly enhanced function, compared to traditionally fabricated bioceramics [Citation19,Citation31,Citation45–61]. For example, using stereolithography technique, silicon nitride (Si3N4), a bioactive bioceramics, has been printed with biomimetic structure, like that of cancellous bone, for use as spinal fusion implant and as femoral heads in hip replacement procedures [Citation54,Citation55]. The printed Si3N4 possessed unique combination of biocompatibility, high fracture toughness, flexural strength, hardness and wear resistance [Citation54,Citation55]. Vat-photopolymerization has also been applied to fabricate calcium phosphate-based bioactive bioceramics implants with unique designs to improve the mechanical and biological functions of the implants. For example, using Voronoi design, β-tricalcium phosphate porous scaffolds with design mimicking cancellous bone was developed [Citation56]. Triply periodic minimal surface and graded porosity structures were applied to fabricate hydroxyapatite and biphasic calcium phosphates with improved mechanical properties and suitable templated materials with enhanced ability to promote bone formation [Citation31,Citation45,Citation46,Citation57–61].
New bioactive bioceramic-resin formulations: Vat-photopolymerization has commonly been used to fabricate bioactive calcium phosphate ceramics. However, only few reports exists in the literature on the application of vat-photopolymerization techniques in other types of bioceramics, such as bioglass (45S5) [Citation49], Mg-substituted wollastonite [Citation50–52], and calcium silicate doped with magnesium and strontium [Citation19,Citation53]. One explanation for this trend, is that calcium phosphate particles have a closer refractive index to common photosensitive resins and lower UV adsorption, compared to the most bioactive inorganic materials (e.g. titanium oxide). Therefore, the printing process and formulation of photosensitive calcium phosphates slurries with high cure depth and resolution are less challenging, compared to other types of bioceramics. To expand on the use of vat-photopolymerization technique in wider bioactive bioceramic compositions, new resins and slurry formulations are required to achieve a better cure depth and to improve dimensional accuracy of the final prints.
It is important to note that even with an optimum slurry, certain limitations exist in the ability of vat-photopolymerization to print bioceramics with complex geometries. This is mainly due to the requirement of the detachment of cured layers which are initially bound to the build platform [Citation20]. During the detachment, mechanical stress is induced in the structure of the cured layer. Depending on the layer geometry, this can lead to defects formation during printing or delamination in the debinding and sintering steps due to the release of stored residual stress. These phenomena, typically occur in layers with large surface area; or layers that have a combination of very thin and very thick structures; and in layers containing connection points with large distances between them or overhangs with high slopes.
One of the current challenges in the vat-photopolymerization of bioceramics in general, is removing of the un-cured slurry from prints. Typically, slurry is removed by a combination of using solvents and compressed air. However, too much exposure to solvents leads to swelling and the introduction of defects. In addition, compressed air, used during cleaning is not suitable for super lightweight structures with intricate geometries. Debinding and sintering of structures can take days, particularly for large solid structures, where gasses generated by burning of the resin need to travel a large diffusion path. Moreover, there is a high risk of formation of cracks and failure of parts due to excessive pressure exerted during accumulation of gasses and fast-heating rates. The last challenge to overcome, is the high cost of vat-photopolymerization in printing bioceramic parts, compared to conventional techniques. For large-scale manufacturing, the production cost of parts produced by the vat-photopolymerization will be always higher than for conventional shaping techniques. For example, additional costs related to cleaning, debinding and sintering steps, the extended time required for printing large constructs, cleaning of equipment, formulation of a proper slurry, as well as handling and processing of prints.
Vat-photopolymerization of ceramics offers several advantages compared to other additive manufacturing techniques. (1) High printing speed: a full cross-section of the object can be cured with one image exposure (mask projection approach), which is unattainable using conventional 3D printing processes. (2) Scalability: multiple components can be manufactured on one platform using a large area resin vat. (3) Low waste: uncured ceramic resin can be collected or left in the vat to be continuously used. (4) Economic: the cost of apparatus for SLA and DLP with reasonable resolution is justifiable and moderate. For large-scale manufacturing, the production costs of parts produced by the vat-photopolymerization will be always higher than for conventional shaping techniques. For example, additional costs related to cleaning, debinding and sintering steps, the extended time required for printing large constructs, cleaning of equipment, formulation of a proper slurry, as well as handling and processing of prints. However, for traditional manufacturing methods there is a significant initial outlay (i.e. for injection moulding tooling and moulds are required to create the parts) before even knowing if the design is suitable, whereas vat-photopolymerization allows fabrication of one-off designs, to trial and compare parts to determine design suitability, which can help to offset the initial cost of the printer/resins, etc. (5) Close to the model surface finish: in comparison to other 3D printing processes, prints have a superior surface finish. The premise for printing bioceramics is the capability to fabricate constructs with customisable shape, pore geometry, pore size, strut shape and mechanical properties. Combined with a precise 3D image of the bone defect (obtained from computed tomography or magnetic resonance imaging) that defines the design specifications, this would enable the manufacture of implants for patient-specific bone regeneration. But to achieve this, certain issues in vat-photopolymerization technologies need to be rectified.
As we discussed, one of the challenges in the vat-photopolymerization of bioceramics is that high-resolution printing of large objects is slow and speed is one of the most important determinants of whether a disruptive manufacturing technology can supplement existing manufacturing processes. For example, it takes a few seconds to generate a large-sized ceramic green body using injection moulding; once the permanent mould is designed, the size and volume of the final product has minimal impact on the manufacturing time. In contrast, high-resolution 3D printing of a large-sized ceramic object might take several hours, depending on the intricacy of the internal structure. Thus, the development of high-speed vat-photopolymerization technologies is a specific aim for many researchers, mainly to reduce separation time, the time required for a layer to cure and cleave, or eliminate the build platform with repeated up and down movements or the need for the vat to hold a large amount of viscous resin. Recent progress has been made by enabling continuous printing from one single droplet of resin, eliminating the need for a vat and for low viscosity resin, as indicated in . Zhang et al [Citation62] developed a technique to fabricate controllable 3D structures from a single droplet ascribing to the receding property of the three-phase contact line (TCL) of the resin droplet. As shown in (Step I), a single droplet of liquid resin is placed on the curing substrate [Citation62]. Then, the supporting plate contacts the curing substrate, with the resin droplet pressed between the plate and interface (Step II). By continuously projecting a UV illumination pattern onto the curing interface and elevating the supporting plate at a constant speed, the liquid resin is cured (solidifies) in that pattern (Step III); in the process, the TCL of the original droplet recedes. After repeating Step III multiple times, the liquid droplet is finally cured into the desired solid 3D structure (Step IV). The well-controlled de-wetting force of liquid resin on the cured structure leaves minimal liquid residue on the substrate, and results in the high wet and net material utilization efficiency in forming a droplet into a 3D structure. This process can also prevent the formation of protruding or stepped sidewalls, induced by extra curing at high printing speed, which require high UV intensity. The critical parameter here, with the introduction of the receding TCL, is the free contact surface property of the droplet system which increases the inner droplet liquid circulation and reduces the adhesion properties of the liquid resin, cured resin and resin vat.
Figure 12. (a) A schematic showing how a single resin droplet is cured into the desired 3D structure. (b–e) Optical images of the curing sequence. With the continuous projection of the UV illumination pattern and with the slow, constant elevation of the supporting plate, the liquid resin is successively cured into the solid structure at the curing interface. Simultaneously, the droplet recedes as the liquid resin is consumed [Citation62].
![Figure 12. (a) A schematic showing how a single resin droplet is cured into the desired 3D structure. (b–e) Optical images of the curing sequence. With the continuous projection of the UV illumination pattern and with the slow, constant elevation of the supporting plate, the liquid resin is successively cured into the solid structure at the curing interface. Simultaneously, the droplet recedes as the liquid resin is consumed [Citation62].](/cms/asset/757158af-7775-448c-b697-8633decd1c4f/yimr_a_2194744_f0012_oc.jpg)
The pursuit of high-resolution, high-speed and high-throughput vat-photopolymerization of bioceramics will require extensive, ongoing research. Nonetheless, this technology has outstanding prospects, including opportunities for commercializing the ready-to-go bioceramic-resin mixtures, the fabrication of load-bearing and zero-defect constructs via successful utilization of the TTP technique, and the design and fabrication of topologically optimized scaffolds for accelerated bone formation. To fulfil these premises, new resins and slurry formulations are required to accelerate the use of novel composition bioceramics not only for DLP, LCD and SSLA but also for the TPP technique. Although current advances promise to tackle processability and brittleness issues, the dimensional accuracy and surface finish are still not ideal due to the layer-by-layer formation of constructs, and a new generation of bioceramic-resin formulations enables nanoscale printing of bioceramics using the TPP technique which potentially leads to a paradigm shift in generating defect-free and ultralightweight structures.
New perspectives and future opportunities in bioceramic implant manufacturing
In this review, we summarized recent advances made in the field of vat-photopolymerization of bioceramic materials, on the two distinct scales of printing, macro/micro and nanoscale, and the associated technical limitations and processing factors.
SLA and DLP printing techniques have significant resolution limitations, with the minimum achievable feature size being 100 microns [Citation31,Citation40,Citation59,Citation60,Citation42,Citation45,Citation47,Citation51,Citation55–58]. This has been identified as a major drawback of these techniques, which limits their ability to create intricate structures with the high precision and accuracy required for implant manufacture. Due to these limitations associated with printing at higher scales, nanoscale printing represents the future of manufacturing bioceramic implants. However, the current state of research on nanoscale printing of bioceramics is quite limited, with only two inert compositions, alumina and zirconia, having been explored so far, and those limited to small constructs. The primary constraint in broadening the scope of this approach to a wider variety of bioceramics is the light-scattering effect caused by the bioceramic particles that need to be blended with photosensitive resin at high concentrations. The concept of printing bioceramics at the nanoscale represents a significant shift in improving the mechanical and biological performance of implants. This is achieved by enabling the accurate design of the architecture of constructs across multiple length scales, including the nano, micro and macro scales, while also creating custom surface topography that is critical in steering the interaction of materials with the biological environment. Given the growing demand for bioceramic implants with better functionality and performance, there is a need for further research in this area, particularly in developing novel techniques that enable the printing of intricate structures at the nanoscale level. Such advances have the potential to revolutionize the field of bioceramic printing, allowing for the creation of more precise and functional implants that offer better patient outcomes. Therefore, continued exploration and development in this field are of utmost importance for advancing bioceramic printing technology.
The key requirement for successful printing at the nanoscale is precise control over the assembly and integration of bioceramic building blocks, such as nanoparticles, with nanoscale accuracy and temporary stabilization to form the green body before sintering. The methodology governing the assembly of these building blocks is, therefore, critical. The recent techniques have focused on using either two-photon laser irradiation or atomic deposition to achieve this assembly. The former technique appears to be the most promising for printing bioceramic implants with nanoscale resolution, but its applicability is hampered by low printing speed and light scattering effects caused by solid particles. A range of bioceramics with nanoscale resolution could be printed using two-photon radiation, provided their building blocks have uniform shapes with particle sizes of less than 30 nm, are effectively mixed with the resin at high loading concentrations, and have a low refractive index mismatch with the resin. However, the process is currently too slow to be practical for manufacturing large bioceramic implants. The use of a continuous droplet system developed by Zhang et al. [Citation62], can substantially improve the printing speed, but the method only applies to small prints. Recently, Han et al. [Citation63] developed a novel solution by utilizing hydrogels patterned by femtosecond light sheets that can act as templates for the direct assembly of nano blocks from a range of materials to form designed nanostructures. This method, combined with the shrinking of the hydrogel, enables high-speed (300 mm3/hour) printing of ceramic materials without concern for light scattering, as the technique does not require using a high concentration of nanoparticles. However, and in order to improve the density of green constructs, the hydrogels need to undergo significant shrinkage (∼13 times). We are clearly in the early stage of developing a universal technique for nanoscale resolution printing of bioceramic implants. At present, bioceramic implant fabrication is restricted to additive manufacturing techniques that can only produce structures within micrometre range. However, the achievement of successful fabrication of bioceramic structures at the nanoscale would represent a significant advancement in the field. The production of nanoscale bioceramic implants offers several advantages over traditional manufacturing techniques, including the ability to control the porosity, surface roughness and topography of the implant. This level of control allows for the precise manipulation of the biological response to the implant, ultimately resulting in improved tissue integration and enhanced mechanical properties. As research in this area continues to progress, we can expect to see significant advances in the field of bioceramic implant fabrication.
Lost in clinical translation: regulatory hurdles and commercialization challenges facing innovative technologies
Further to the technical challenges of manufacturing patient-specific bioceramic implants with sophisticated and complex designs, there is a major challenge in navigating the pathway to clinical translation, specifically regulatory approval. While bioceramic implants manufactured using traditional technologies are already authorized for clinical use, when bioceramic implants are made using vat-photopolymerization technologies, even with similar geometry and physical characteristics to traditionally manufactured implants, the path to translation will be more burdensome. This section discusses the regulatory and commercial landscape, and presents recommendations to ease the translation of emerging innovative technologies, such as bioceramic implant materials, into commercially marketed, clinically approved medical devices.
Despite the demand for new medical devices, the translation of emerging innovative materials and technologies into commercially marketed, clinically approved medical devices, is a well-known challenge; characterized by opposing drives, but the necessary intersection of academia, industry and regulatory bodies [Citation64]. Most commonly, it is the role of academic researchers to advance scientific knowledge and clinical breakthroughs, developing revolutionary materials and technologies that disrupt the status-quo and present a new solution to current medical challenges [Citation65]. Regulatory bodies review device submissions, often There is a tendency to compare proposed new materials and technologies against existing medical devices, questioning whether a new device brings about any additional risks to patients [Citation66], inherently favouring devices that are similar to older technologies, rather than a revolutionary approach [Citation67].
The role of industry tends to lie between that of academic researchers and regulators, with expertise in commercial-scale manufacturing and implementation of quality systems that ensure the development process of new materials and technologies will be acceptable to the regulatory bodies [Citation68]. The downfall of many commercialization projects is the challenge of repeating promising research results, in an independent industry facility, with validated methods for production and analysis, at a scale and cost that is commercially viable [Citation69]. The roles of academia, industry and regulatory bodies are symbiotic – each playing a vital part in the progression of innovative materials and technologies from the research bench to the clinic. However, the often-conflicting drives and approaches of each group create obstacles in ensuring that the best technologies are available to patients.
Different requirements of jurisdictions globally affect device availability and patient safety
The process for assessing and approving a new medical device is not globally centralized; instead, there is a separate process for each country/jurisdiction in which the device is intended to be sold. Most countries have established a single, centralized regulatory body to review and approve medical device submissions, based on its federal regulations, for example, the Food and Drug Administration (FDA) in the U.S.A., the Therapeutic Goods Administration (TGA) in Australia, the Pharmaceuticals and Medical Devices Agency (PMDA) in Japan, and Health Canada in Canada [Citation70]. In the European Union (EU), however, regulatory approval of medical devices is conducted through a network of agencies. Each country in the EU uses a competent authority to transpose the requirements of the EU 2017/745 Medical Devices Regulation (MDR) into national law, and to designate one of more notified body to act as independent third party assessors in granting regulatory approval [Citation71].
Here we examine the differences between the centralized regulatory approach of the U.S.A. and the decentralized approach of the EU, as these are the major medical device markets with strong regulatory frameworks and practices [Citation72].
By virtue of being a single authority system, the FDA can provide better coordination and enforcement, but this comes at the expense of the approval process being rigid, lengthy and expensive [Citation73]. Conversely, while the EU system of multiple notified bodies creates flexibility and faster approval of devices, this can come at the expense of patient outcomes. Different applications and interpretations of the regulations by different notified bodies can allow manufacturers to exploit loopholes and lodge a submission with the notified body most likely to approve their device [Citation74]. This is further complicated by the independent nature of European notified bodies, where rejecting a manufacturer’s device submission equates to loss of the customer and associated income [Citation75].
A further difference is in the time it takes for a medical device to be made clinically available. This is influenced by the timing for reimbursement approval, which is how manufacturers receive financial reimbursement from government agencies and private insurance companies, based on the nature of the device. While it is generally agreed that the regulatory approval process takes longer in the U.S.A. than in the EU, the reimbursement approval process takes longer in the EU, making the overall timelines similar, or perhaps shorter in the U.S.A. [Citation76].
Recommendation: Harmonization between global medical device regulatory bodies should be sought, to ensure that a patient’s access to health treatments is determined by the capabilities of medical technologies, not the country in which they reside, an effort championed by the World Health Organization (WHO) [Citation77]. This may take the form of 1) forming a single global medical device regulatory body, which establishes a global legislative-like framework for device approval, reviews all new device submissions, and approves them for use globally; 2) forming a single global medical device regulatory body, which establishes a global legislative-like framework, but continued use of local regulatory bodies to review new device submissions based on the global framework or 3) continued use of local legislation and regulatory bodies to review new device submissions, but approval in one jurisdiction can be translated to global jurisdictions with equivalently established regulatory frameworks. Such an approach should reduce the burden on regulatory bodies, and ensure that the resources of manufacturers and researchers are dedicated to patients and solving unmet clinical needs, rather than bureaucratic processes.
Disconnect between rigid regulatory frameworks and the emergence of innovative and personalized technologies
As discussed earlier, the emergence of 3D printing enables the fabrication of implants for which the geometry and material properties can be adjusted to satisfy the requirements of specific patients. While attractive in principle, this is challenging for regulators in practice because personalized devices (patient-matched and/or custom-made) with varying sizes and shapes fall outside traditional device classifications. Both categories of devices stray from the standard product definitions and thus require additional resources for manufacturers to pursue a non-standard approval pathway [Citation78].
The FDA, for example, classifies medical devices as Class I (low risk: no contact with internal systems, e.g. elastic bandages and tongue depressors), Class II (higher risk: sustained contact with patient including internal systems, and/or use as diagnostic tools, e.g. catheters and absorbable sutures), or Class III (highest risk: sustain or support life, implants, e.g. pacemakers, total joint replacements, defibrillators) [Citation79] based on the risks they present to patients, and the regulatory controls necessary to provide a reasonable assurance of safety and effectiveness. Similar classification systems are used in other jurisdictions [Citation80].
As well as these device classifications, the FDA uses product codes, which are assigned to generic categories of device, such as ‘MQV’ for Calcium Compound Bone Void Fillers [Citation81]. The use of device classifications and product codes provides a standardized approach to assessing novel medical devices. However, device classifications and product codes fall short when emerging technologies lie outside their definitions. For instance, product code ‘PHM’ defines a thoracic intervertebral fusion device with a supplementary bone graft, a definition based on the historical inability of materials both to support the loading of the spinal column (requiring strength) and to facilitate bone fusion (requiring bioactivity). However, if development of novel materials and advanced 3D printing technologies enables a bioceramic device optimized for strength and bioactivity such that no supplementary bone graft is needed, it falls outside the ‘PHM’ definition, so cannot be assessed in a straightforward manner, increasing the time and cost for approval. This may incline a manufacturer to redundantly include a lumen for bone graft, an arguably inferior outcome that involves less advanced technology and increases the likelihood of harm to patients via bone graft harvesting.
There may also be reimbursement hurdles for 3D printed personalized devices. Certain jurisdictions, including Australia, offer no reimbursement for patient-specific or custom-made medical devices. The use of such devices is reliant on manufacturers and clinicians being granted ex-gratia pre-approval by medical insurance companies on a case-by-case basis [Citation82]. While personalized devices such as 3D-printed bioceramic implants hold great promise, implementing them is a major challenge due to the regulatory burden on demonstrating safety and effectiveness of non-standard device types.
Recommendation: Regulatory frameworks should assist reviewers to ensure not only that devices available to patients are safe and effective, but also that the best materials and devices are made available, including those with recent technological advances. As such, these frameworks should be used as tools to guide regulatory bodies, but not as rigid definitions. Using a definition to broadly describe the intended use of a device helps to ensure a review panel contains suitably qualified subject-matter experts, but that expertise should be trusted to review technical device features; rather than the submission being diverted to a more burdensome process based on classification definition before reaching those experts. Softening the definition boundaries while still performing a comprehensive review of new technology types in a straightforward process, may encourage manufacturers to prioritize the development of truly innovative material and device developments to revolutionize health treatments.
Applicability of established regulatory pathways to personalized devices
There are two main pathways to obtaining FDA approval for medical devices: the pre-market notification (PMN) process, and the more rigorous pre-market approval (PMA) process [Citation83].
A PMA is the strictest application process and is required for any new device for which there is no existing equivalent. To obtain PMA approval, it must be demonstrated that a device is safe and effective in its intended use. In general, this means clinical evidence is required for most new Class III devices, so the process involves considerable cost and time. In the period 2006-2016, only about 2% of medical devices that underwent the PMA process were approved [Citation84].
A PMN, also known as a 510(k) application, is a process where a new device is claimed to be safe and efficacious based on being substantially equivalent to another legally marketed device, a predicate device. This process fast-tracks approval, often without the need for clinical data, thereby lowering the time and cost of getting devices to patients. However, the framework for deeming devices ‘equivalent’ is often criticized as being too loose. So, too, is the use of ‘serial predicates’, where a new device is approved based on a predicate device that was itself approved based on another, different predicate; meaning that approval of the newest device may come decades after the original PMA and the clinical evidence supporting it [Citation84]. In 2017, the FDA cleared 3,173 devices through the 510(k) system (82% of all devices approved in the U.S.A.), and nearly 20% of these were based on a predicate device more than 10 years old [Citation85]. The PMN process incentivizes manufacturers to invest in materials and devices that represent incremental improvements rather than true innovations, since the pathway to market is faster and cheaper [Citation83].
Reproduced below () is the FDA’s decision-making flowchart for the 510(k) process [Citation86]. Assuming a predicate device is available with the same intended use, the FDA will evaluate whether the new and predicate devices have the same technological characteristics, which include materials, design, energy source, and other device features, and whether any differences in technological characteristics lead to different questions of safety and effectiveness. In addition, the FDA has produced a guidance document, Technical Considerations for Additive Manufactured Medical Devices [Citation87], which highlights the unique aspects of the additive manufacturing process and additive manufacturing materials. The material controls and material characterization intersects with Decision 4 of the 510(k) process, and this is where 3D-printed devices are most at risk of being designated NSE and forced into the burdensome PMA process. The failure of the 510(k) process to capture the nuances of 3D printing materials and to accommodate devices fabricated by additive manufacturing therefore discourages the development of these innovative medical devices by manufacturers.
Figure 13. FDA 510(k) Decision-Making Flowchart [Citation86].
![Figure 13. FDA 510(k) Decision-Making Flowchart [Citation86].](/cms/asset/bf9f38d3-b9ea-4877-b50c-792b0c71cd7c/yimr_a_2194744_f0013_ob.jpg)
Recommendation: There should be open multi-directional communication between researchers, industry, and regulatory bodies to guide increased understanding of 3D printing materials and additively manufactured products. This will enable a more balanced review process that takes into consideration patient safety and provides greater scope to make novel medical devices available to patients. While there may be a relative lack of clinical history of additively manufactured devices, the research sector has immense experience with these technologies, and a state of clinical history of additively manufactured devices can only be reached if manufacturers pursue their development, which requires a more balanced regulatory environment. Leveraging the expertise of the research sector may allow developments based on state-of-the-art knowledge.
Reliance on consensus standards for testing guidelines
Standards are the cornerstone of testing for regulatory submissions; they provide guidelines, specifications and procedures on how medical devices should be designed, developed, manufactured, and evaluated [Citation88]. The FDA reviews available standards, and marks those it considers appropriate as consensus standards, to streamline review, provide clear regulatory expectations, and facilitate market entry for safe and effective medical products [Citation89].
Although standards are a valuable resource, they are not always applicable to new and emerging materials and technologies. For example, standard ASTM F2077 [Citation90], first released in 2000, governs the Test Methods For Intervertebral Body Fusion Devices. In line with the available technology at the time of release, the standard assumes that these devices are manufactured from polyetheretherketone (PEEK), and therefore recommends a test set-up suitable for evaluating this rigid thermoplastic material. More recently, such devices are increasingly being manufactured from cellular metals and custom ceramics with material properties that differ significantly from PEEK, so cannot be adequately assessed under the recommended test set-up for PEEK. Standard test methods inherently take time to develop and cannot keep up with the development of new and emerging technologies. However, the FDA recommendation that intervertebral body devices be assessed according to this standard, despite a device being manufactured from a novel material prevents fair assessment of these devices [Citation91].
Recommendation: Reduced reliance on unmodified consensus standard methods for evaluating novel devices, and increased involvement of research-sector subject matter experts to validate a testing method. Just as the research sector relies on multidisciplinary collaboration, the medical device sector would also benefit from increased collaboration between regulators, industry and researchers. Standards development involves rigorous review by subject matter experts, but naturally, these cannot stay ahead of technological advancements. When a new material falls outside of the recommended boundaries of a standard, the systems should allow regulators to review that technology, with increased involvement of research sector subject matter experts to certify the suitability of a testing method.
Continuing challenges to medical device regulation and approval
As the capabilities and knowledge of the medical devices research field have grown, so too has the sophistication of medical devices, including use of novel materials and manufacturing techniques leading to regulatory challenges in evaluating these emerging and innovative technologies. While some government and medical bodies view the FDA 510(k) process as lacking rigor in screening for the safety and effectiveness of new devices, and call for it to be scrapped, industry views the process as too slow, risk adverse and expensive [Citation92]. On the other hand, the EU regulatory process has been criticized for providing insufficient safeguards for technologies that affect morbidity, mortality and health-related quality of life [Citation73]. Multiple devices approved by the EU have been denied approval by the FDA, and have later been recalled in Europe due to patient safety concerns [Citation93].
Regulatory bodies are emerging in more countries, for example, the National Medical Products Administration (NMPA) in China (formerly China Food and Drug Administration, CFDA) and the Ministry of Food and Drug Safety (MFDS) in South Korea. The emergence of new regulatory markets – including both approval and reimbursement – will allow multi-national companies to enter these regions, thus ensuring increased availability of technologies to citizens, and driving business innovation by manufacturers. However, this also creates challenges due to the rapidly changing regulatory environments and lack of available information and documents published in English to communicate local requirements to global manufacturers [Citation94].
While there are many opinions and controversies about the medical device regulatory landscape, there is also consensus on certain matters. First, it is widely agreed that the regulatory approval pathways should better address patient safety via improved post-market surveillance [Citation95], while still encouraging innovation with emerging technologies. Second, the symbiotic relationships between industry, academia, and regulatory authorities need to be well leveraged. In a review of devices developed in academia, collaboration with industry was associated with greater regulatory approval, and in 218 clinical studies that led to the regulatory approval of 99 new devices, industry was found to have a role in the development and approval of most [Citation96], highlighting the vital role that industry plays, sitting between that of researchers and the regulatory approval process. Therefore, collaboration between academia and industry to foster technology transfer is vital for the ongoing development of innovative technologies from the bench to commercial outcomes.
Conclusion
Despite the great progress made in high-resolution vat-photopolymerization of bioceramics, the obstacles to widen its application to a wider range of bioceramics, remains a challenge. The existing obstacles are increasing resolution of printing to submicron scales, large-scale production, increasing speed of the process, and optimization of processing and postprocessing steps. While technical advances in vat-polymerization will open the possibility for nanoscale printing and manufacturing personalized bioceramic implants, the reality remains that translation into a commercialized product relies on successful regulatory approval. However, the type and timelines of device availability to patients is often influenced by rigid frameworks that were developed for historical materials and technologies, which have not kept up with modern personalization capabilities. This paper has presented recommendations to ensure that emerging and innovative materials and technologies are helped rather than hampered through the regulatory pathway.
Acknowledgements
The authors acknowledge the Australia National Health and Medical Research Council and the Australian Research Council. Authors would like to thank Prof. Colin Dunstan for his contribution and insight in preparation of this manuscript.
Disclosure statement
No potential conflict of interest was reported by the author(s).
References
- Marongiu G, Verona M, Cardoni G, et al. Synthetic bone substitutes and mechanical devices for the augmentation of osteoporotic proximal humeral fractures: a systematic review of clinical studies. J Funct Biomater. 2020;11.
- Vallet-Regí M, Ruiz-Hernández E. Bioceramics: from bone regeneration to cancer nanomedicine. Adv Mater. 2011;23:5177–5218.
- Janssen R, Scheppokat S, Claussen N. Tailor-made ceramic-based components-advantages by reactive processing and advanced shaping techniques. J Eur Ceram Soc. 2008;28:1369–1379.
- Bouville F, Maire E, Meille S, et al. Strong, tough and stiff bioinspired ceramics from brittle constituents. Nat. Mater. 2014;13:508–514.
- Lin K, Sheikh R, Romanazzo S, et al. 3D printing of bioceramic scaffolds-barriers to the clinical translation: from promise to reality, and future perspectives. Materials (Basel). 2019;12:2660.
- Zhang F, Li Z, Xu M, et al. A review of 3D printed porous ceramics. J Eur Ceram Soc. 2022;42:3351–3373.
- Colombo P, Degischer HP. Highly porous metals and ceramics. Mater Sci Technol. 2010;26:1145–1158.
- He R, Liu W, Wu Z, et al. Fabrication of complex-shaped zirconia ceramic parts via a DLP- stereolithography-based 3D printing method. Ceram Int 2018;44:3412–3416.
- Pelz JS, Ku N, Meyers MA, et al. Additive manufacturing of structural ceramics: a historical perspective. J Mater Res Technol. 2021;15:670–695.
- Standard terminology for additive manufacturing – general principles – terminology. ASTM Int 2015, 52900, 1–9.
- Gmeiner R, Deisinger U, Schönherr J, et al. Additive manufacturing of bioactive glasses and silicate bioceramics. J Ceram Sci Technol. 2015;6:75–86.
- Bose S, Vahabzadeh S, Bandyopadhyay A. Bone tissue engineering using 3D printing. Mater Today. 2013;16:496–504.
- Shahzad K, Deckers J, Zhang Z, et al. Additive manufacturing of zirconia parts by indirect selective laser sintering. J Eur Ceram Soc. 2014;34:81–89.
- Roohani-Esfahani S-I, Newman P, Zreiqat H. Design and fabrication of 3D printed scaffolds with a mechanical strength comparable to cortical bone to repair large bone defects. Sci Rep. 2016;6:1–8.
- Kumar A, Mandal S, Barui S, et al. Low temperature additive manufacturing of three dimensional scaffolds for bone-tissue engineering applications: processing related challenges and property assessment. Mater Sci Eng R Reports. 2016;103:1–39.
- Diptanshu; Young, E.; Ma, C.; Obeidat, S.; Pang, B.; Kang, N. Ceramic additive manufacturing using vat photopolymerization 2018, 1.
- Barba A, Maazouz Y, Diez-Escudero A, et al. Osteogenesis by foamed and 3D-printed nanostructured calcium phosphate scaffolds: effect of pore architecture. Acta Biomater. 2018;79:135–147.
- Entezari A, Roohani I, Li G, et al. Architectural design of 3D printed scaffolds controls the volume and functionality of newly formed bone. Adv Healthc Mater. 2019;8:1–12.
- Mirkhalaf M, Goldsmith J, Ren J, et al. Highly substituted calcium silicates 3D printed with complex architectures to produce stiff, strong and bioactive scaffolds for bone regeneration. Appl Mater Today. 2021;25:1–12.
- Li X, Chen Y. Vat-Photopolymerization-Based ceramic manufacturing. J Mater Eng Perform. 2021;30:4819–4836.
- Ma H, Feng C, Chang J, et al. 3D-printed bioceramic scaffolds: from bone tissue engineering to tumor therapy. Acta Biomater. 2018;79:37–59.
- Vorndran E, Moseke C, Gbureck U. 3D printing of ceramic implants. MRS Bull. 2015;40:127–136.
- Johnson ZM, Yuan Y, Li X, et al. Mesenchymal stem cells and three-dimensional-osteoconductive scaffold regenerate calvarial bone in critical size defects in swine. Stem Cells Transl Med. 2021;10:1170–1183.
- Badev A, Abouliatim Y, Chartier T, et al. Photopolymerization kinetics of a polyether acrylate in the presence of ceramic fillers used in stereolithography. J Photochem Photobiol A Chem. 2011;222:117–122.
- Zakeri S, Vippola M, Levänen E. A comprehensive review of the photopolymerization of ceramic resins used in stereolithography. Addit Manuf. 2020;35:1–14.
- Sun H-B, Kawata S. Two-photon photopolymerization and 3D lithographic microfabrication. Adv Polym Sci. 2004;170:169–273.
- Song X, Chen Y, Lee TW, et al. Ceramic fabrication using Mask-Image-Projection-based Stereolithography integrated with tape-casting. J Manuf Process. 2015;20:456–464.
- Mitteramskogler G, Gmeiner R, Felzmann R, et al. Light curing strategies for lithography-based additive manufacturing of customized ceramics. Addit Manuf. 2014;1:110–118.
- Brie J, Chartier T, Chaput C, et al. A new custom made bioceramic implant for the repair of large and complex craniofacial bone defects. J Cranio-Maxillofacial Surg. 2013;41:403–407.
- Schmidt J, Elsayed H, Bernardo E, et al. Digital light processing of wollastonite-diopside glass-ceramic complex structures. J Eur Ceram Soc. 2018;38:4580–4584.
- Baino F, Magnaterra G, Fiume E, et al. Digital light processing stereolithography of hydroxyapatite scaffolds with bone-like architecture, permeability, and mechanical properties. J Am Ceram Soc. 2022;105:1648–1657.
- Brigo L, Schmidt JEM, Gandin A, et al. 3D nanofabrication of SiOC ceramic structures. Adv Sci. 2018;5:1–8.
- Bauer J, Hengsbach S, Tesari I, et al. High-strength cellular ceramic composites with 3D microarchitecture. Proc Natl Acad Sci U.S.A. 2014;111:2453–2458.
- Meza LR, Das S, Greer JR. Strong, lightweight, and recoverable three-dimensional ceramic nanolattices. Science (80-.). 2014;345:1322–1326.
- Wen X, Zhang B, Wang W, et al. 3D-printed silica with nanoscale resolution. Nat Mater. 2021;20:1506–1511.
- Hafkamp T, van Baars G, de Jager B, et al. A feasibility study on process monitoring and control in vat photopolymerization of ceramics. Mechatronics (Oxf). 2018;56:220–241.
- Halloran JW, Tomeckova V, Gentry S, et al. Photopolymerization of powder suspensions for shaping ceramics. J Eur Ceram. Soc. 2011;31:2613–2619.
- Gentry SP, Halloran JW. Depth and width of cured lines in photopolymerizable ceramic suspensions. J Eur Ceram Soc. 2013;33:1981–1988.
- Wang K, Qiu M, Jiao C, et al. Study on defect-free debinding green body of ceramic formed by DLP technology. Ceram Int. 2020;46:2438–2446.
- Chen F, Wu Y, Zhu H, et al. Mechanical and biological properties of ZrO2 bioceramics by stereolithography technique. Kuei Suan Jen Hsueh Pao/Journal Chinese Ceram. Soc. 2021;49:1837–1845.
- Goldberg M, Obolkina T, Smirnov S, et al. The influence of co additive on the sintering, mechanical properties, cytocompatibility, and digital light processing based stereolithography of 3Y-TZP-5Al2O3 ceramics. Materials (Basel). 2020;13:1–23.
- Zhang C, Jiang Z, Zhao L, et al. Stability, rheological behaviors, and curing properties of 3Y–ZrO2 and 3Y–ZrO2/GO ceramic suspensions in stereolithography applied for dental implants. Ceram Int. 2021;47:13344–13350.
- Li Y, Wang M, Wu H, et al. Cure behavior of colorful ZrO2 suspensions during digital light processing (DLP) based stereolithography process. J Eur Ceram Soc. 2019;39:4921–4927.
- Chen F, Wu Y-R, Wu J-M, et al. Preparation and characterization of ZrO2-Al2O3 bioceramics by stereolithography technology for dental restorations. Addit Manuf. 2021;44:1–14.
- Wang Z, Huang C, Wang J, et al. Design and characterization of hydroxyapatite scaffolds fabricated by stereolithography for Bone Tissue Engineering Application 2020. Procedia CIRP. 2020;89:170–175.
- Liu K, Wu X, Liu J, et al. Design and manufacture of a customized, large-size and high-strength bioactive HA osteoid composite ceramic by stereolithography. Ceram Int. 2022;49:11630–11640.
- Schmidleithner C, Malferarri S, Palgrave R, et al. Application of high resolution DLP stereolithography for fabrication of tricalcium phosphate scaffolds for bone regeneration. Biomed Mater. 2019;14:45018.
- Huang X, Dai H, Hu Y, et al. Development of a high solid loading β-TCP suspension with a low refractive index contrast for DLP -based ceramic stereolithography. J Eur Ceram Soc. 2021;41:3743–3754.
- Thavornyutikarn B, Tesavibul P, Sitthiseripratip K, et al. Porous 45S5 Bioglass®-based scaffolds using stereolithography: effect of partial pre-sintering on structural and mechanical properties of scaffolds. Mater Sci Eng C. 2017;75:1281–1288.
- Wu R, Li Y, Shen M, et al. Bone tissue regeneration: the role of finely tuned pore architecture of bioactive scaffolds before clinical translation. Bioact Mater. 2021;6:1242–1254.
- Wang J, Dai X, Peng Y, et al. Digital light processing strength-strong ultra-thin bioceramic scaffolds for challengeable orbital bone regeneration and repair in situ. Appl Mater Today. 2021;22:1–13.
- Lu F, Wu R, Shen M, et al. Rational design of bioceramic scaffolds with tuning pore geometry by stereolithography: microstructure evaluation and mechanical evolution. J Eur Ceram Soc. 2021;41:1672–1682.
- Li Y, Wu R, Yu L, et al. Rational design of nonstoichiometric bioceramic scaffolds via digital light processing: tuning chemical composition and pore geometry evaluation. J Biol Eng. 2021;15:1–12.
- Bal BS, Rahaman MN. Orthopedic applications of silicon nitride ceramics. Acta Biomater. 2012;8:2889–2898.
- Schwarzer-Fischer E, Zschippang E, Kunz W, et al. CerAMfacturing of silicon nitride by using lithography-based ceramic vat photopolymerization (CerAM VPP). J Eur Ceram Soc. 2023;43:321–331.
- Li J, Guo D, Li J, et al. Irregular pore size of degradable bioceramic Voronoi scaffolds prepared by stereolithography: osteogenesis and computational fluid dynamics analysis. Mater Des. 2022;224:1–16.
- Zhang Y, Zhang Q, He F, et al. Fabrication of cancellous-bone-mimicking β-tricalcium phosphate bioceramic scaffolds with tunable architecture and mechanical strength by stereolithography 3D printing. J Eur Ceram Soc. 2022;42:6713–6720.
- Navarrete-Segado P, Tourbin M, Frances C, et al. Masked stereolithography of hydroxyapatite bioceramic scaffolds: from powder tailoring to evaluation of 3D printed parts properties. Open Ceram. 2022;9:100235.
- Van hede D, Liang B, Anania S, et al. 3D-Printed synthetic hydroxyapatite scaffold with in silico optimized macrostructure enhances bone formation in vivo. Adv Funct Mater. 2022;32:1–10.
- Dong D, Su H, Li X, et al. Microstructures and mechanical properties of biphasic calcium phosphate bioceramics fabricated by SLA 3D printing. J Manuf Process. 2022;81:433–443.
- Han X, Sun M, Chen B, et al. Lotus seedpod-inspired internal vascularized 3D printed scaffold for bone tissue repair. Bioact Mater. 2021;6:1639–1652.
- Zhang Y, Dong Z, Li C, et al. Continuous 3D printing from one single droplet. Nat Commun. 2020;11:4685.
- Han F, Gu S, Klimas A, et al. Three-dimensional nanofabrication via ultrafast laser patterning and kinetically regulated material assembly. Science (80-.). 2022;378:1325–1331.
- Páez-Avilés C, Juanola-Feliu E, Bogachan-Tahirbegi I, et al. Innovation and technology transfer of medical devices fostered by cross-disciplinary communities of practitioners. Int J Innov Manag. 2015;19:1540012.
- Gambardella A. Competitive advantages from in-house scientific research: The US pharmaceutical industry in the 1980s. Res Policy. 1992;21:391–407.
- Jefferys DB. The regulation of medical devices and the role of the Medical Devices Agency. Br. J. Clin. Pharmacol. 2001;52:229–235.
- Wizemann T. Public health effectiveness of the FDA 510 (k) clearance process: balancing patient safety and innovation: workshop report; 2010;.
- Lee SS. Medical technology development and globalization: the role of the medical device industry. J Korean Med Assoc. 2014;57:919–926.
- Moilanen J, Montonen T, Eriksson P. The conflictual sense of commercialisation and academic entrepreneurship. Int J Hum Resour Dev Manag. 2021;21:165–177.
- Therapeutic Goods Administration. List of countries and jurisdictions determined to be comparable overseas regulators (CORs). Available online: https://www.tga.gov.au/list-countries-and-jurisdictions-determined-be-comparable-overseas-regulators-cors. October, 2019
- European Medicines Agency. Medical Devices. Available online: https://www.ema.europa.eu/en/human-regulatory/overview/medical-devices. January 2021
- Bergsland J, Elle OJ, Fosse E. Barriers to medical device innovation. Med Devices. 2014;7:205.
- Sorenson C, Drummond M. Improving medical device regulation: the United States and Europe in perspective. Milbank Q. 2014;92:114–150.
- Cohen D BM. Europeans are left to their own devices. Br Med J. 2011;342:d2952.
- McCulloch P. The EU’s system for regulating medical devices: now is the time for radical change. Br Med J. 2012;345:e7126.
- Basu S, Hassenplug J. Patient access to medical devices – a comparison of U.S. and European review processes. N Engl J Med. 2012;367:485–488.
- Lamph S. Regulation of medical devices outside the European union. J. R. Soc. Med. 2012;105:12–21.
- Therapeutic Goods Administration (November 2021). Personalised medical devices (including 3D-printed devices) Regulatory changes for custom-made medical devices.
- Baura GD. Corporate considerations on biomaterials and medical devices: case studies in regulation and reimbursement. Claremont (CA): Academic Press; 2013.
- Food and Drug Administration. Overview of Medical Device Classification and Reclassification Available online: https://www.fda.gov/about-fda/cdrh-transparency/overview-medical-device-classification-and-reclassification. December 2017.
- Food and Drug Administration. Product Code Classification Database Available online: https://www.accessdata.fda.gov/scripts/cdrh/cfdocs/cfPCD/classification.cfm. March 2018.
- D’Urso. P. Searching for an even playing field: a call to government to assist Australian medical innovation; 2016.
- Van Norman GA. Drugs, devices, and the FDA: part 1: an overview of approval processes for drugs. JACC Basic to Transl Sci. 2016;1:170–179.
- Federal Register Notice 80(148). Department of Health and Human Services, Federal Food and Drug Administration. Augest 2015.
- Food and Drug Administration. Statement from FDA Commissioner Scott Gottlieb, M.D. and Jeff Shuren, M.D., on transformative new steps to modernize FDA’s 510(k) program to advance the Review of the Safety and Effectiveness Of Medical Devices. Available online: https://www.fda.gov/news-events/press-announcements/statement-fda-commissioner-scott-gottlieb-md-and-jeff-shuren-md-director-center-devices-and. 2018.
- Food and Drug Administration. The 510(k) Program: Evaluating Substantial Equivalence in Premarket Notification [510(k)]. Guidance for Industry and Food and Drug administration Staff Available online: https://www.fda.gov/regulatory-information/search-fda-guidance-documents/510k-program-evaluating-substantial-equivalence-premarket-notifications-510k. May 2019.
- Food and Drug Administration. Technical Considerations for Additive Manufacured Medical Devices. Guidance for Industry and Food and Drug Administration Staff Available online: https://www.fda.gov/regulatory-information/search-fda-guidance-documents/technical-considerations-additive-manufactured-medical-devices. 2014
- Ho C, Jensen D, Lacy F, et al. Use of standards in the review of medical devices. J Electrocardiol. 2005;38:171–174.
- Food and Drug Administration. Standards and Conformity Assessment Program Available online: https://www.fda.gov/medical-devices/premarket-submissions-selecting-and-preparing-correct-submission/standards-and-conformity-assessment-program. 2019
- Test Methods For Intervertebral Body Fusion Devices. Am. Soc. Test. Mater. 2018, ASTM F2077-18.
- Recognized Consensus Standards. ASTM F2077-18. In Food and Drug Administration; 2019.
- The FDA 510(k) Clearance Process at 35 Years. In Institute of Medicine. Medical Devices and the Public’s Health.Washington, DC: National Academies Press; 2011.
- Heneghan C. The saga of poly implant prothèse breast implants. Br Med J. 2011;344:e306.
- Lueddemann T, Chang D, Sahin S, et al. Medical device approval process in China since the introduction of the China Food and Drug Administration. Proceedings of the IEEE Symposium on Product Compliance Engineering (ISPCE). 2016; p. 1–6.
- Normand S, Hatfield L, Drozda J. Postmarket surveillance for medical devices: America’s new strategy. Br Med J. 2012;345:1–3.
- Marcus HJ, Payne CJ, Hughes-Hallett A, et al. Regulatory approval of new medical devices: cross sectional study. Br Med J. 2016;353:1–5.