Abstract
Purpose: The threat of a radiological/nuclear event is a critical concern for all government agencies involved in national security and public health preparedness. Countermeasures that are safe, easily administered, and effective at diminishing or eliminating adverse health effects to individuals and the overall public health impact of radiation exposure are urgently needed. Radiation countermeasures included in this three-part series have been classified under various subheadings based specifically on their developmental stages for United States Food and Drug Administration (FDA) approval. We have included FDA-approved agents for acute radiation syndrome (ARS) in part I. This is part II in which we have reviewed FDA-approved agents for limited indications, internalized radionuclides, emesis, late effects, radiomitigators available in the strategic national stockpile (SNS), agents with FDA investigational new drug (IND) status, and those with NHP efficacy data without FDA IND. Agents discussed in part III are those agents that have been peer reviewed, published, and have demonstrated significant survival benefits in animal models of ARS. Agents investigated in in vitro models only or studied in animal models without peer-reviewed publications have not been included.
Conclusions: The dearth of FDA-approved radiation countermeasures has prompted intensified research for a new generation of radiation countermeasures. A number of promising radiation countermeasures are currently moving forward with continued support and effort by both governmental agencies and by publicly and privately held pharmaceutical companies. There is a limited number of countermeasures which are progressing well following the Animal Rule and may get approved in the near future, thus serving to close the gap of this critically important, unmet radiobiomedical need.
Introduction
The development and availability of suitable radiation countermeasures needed to protect individuals and the public at large from serious, potentially lethal injuries following unwanted radiation exposures remains a substantial unmet medical need and has been recognized as a high priority area by the U.S. government. We have focused on medical countermeasures that are currently under development and that have been evaluated for efficacy largely in animal models for a survival benefit in this article. Such agents are expected to be assessed fully for their safety and efficacy, with the expectation of the latter approved by the Food and Drug Administration (FDA) for use in humans (). It is beyond the scope of this article, however, to include all medicinals that are presently being researched and developed as potential countering agents against either low doses of radiation or as anti-cancer agents, for those agents which are used as dietary supplements, or other plant products which may not be regulated by FDA for approval, use, and marketing. We have discussed mostly medical countermeasures (MCM) that are being developed for hematopoietic acute radiation syndrome (H-ARS) or gastrointestinal ARS (GI-ARS), although we briefly discuss a number of additional agents that are currently under development for both pulmonary and cutaneous syndromes. This is part II of a three-part series in which we have reviewed countermeasures approved by the FDA for limited indications, internalized radionuclides, emesis, late effects as well as a number of radiomitigators available in the strategic national stockpile (SNS) for H-ARS. Several newer agents with FDA investigational new drug (IND) status as well as those with NHP efficacy data without IND are also included (). Radiation countermeasures included in this three-part series have been classified under various subheadings based on their developmental stages for FDA approval. Agents discussed in part III included in ‘Countermeasures under early stages of development’ are those agents that have appeared in peer-reviewed publications, demonstrating significant survival benefits in animal models of ARS. Agents which have been investigated only in in vitro models or studied in animal models without peer-reviewed publication have not been included in that section. We fully recognize that the listing of new drugs may not be exhaustive due to the lack of accessibility to reported data regarding the nature and medicinal actions of select agents. This inaccessibility is created largely by pharmaceutical companies with vested financial interests in protecting potentially marketable agents. Issues of confidentiality and the ‘Intellectual Property’ rights of corporations and government agencies, particularly the U.S. FDA, also limit accessibility. Despite the significant progress that has been made, additional effort is still needed to have multiple countermeasures approved for various radiological indications (Singh et al. Citation2012b, Citation2015a, Citation2016c).
Figure 1. Current status of radiation countermeasures for radiological and nuclear threats. Three countermeasures for ARS (Neupogen, Neulasta, and Leukine) and four agents for internalized radionuclides (Prussian Blue, Potassium iodide, Ca-DTPA, and Zn-DTPA) have been procured for SNS, and nine radiation countermeasures have U.S. FDA IND. Color coding: red, acute and late-arising injuries; light pink, MCM with FDA IND status; baby blue, new countermeasures under development without IND status; burnt orange, FDA fully-approved medicals for internalized radionuclides; light green, FDA fully-approved medicals for ARS within the SNS.
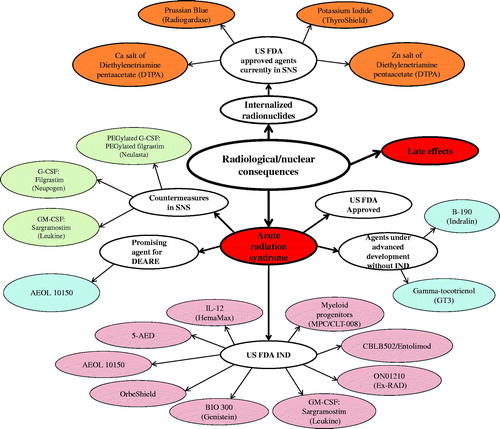
Countermeasures approved for limited indications
Amifostine
Amifostine has limited FDA approval for use in humans, but only for narrow clinical oncologic indications. These ‘approved’ indications do not include protection against acute radiation syndrome (ARS) arising from unwanted radiation exposures. The drug’s major limitations are its toxic side-effects at doses required for this indication (i.e. protection against ARS) (Culy & Spencer Citation2001; Wasserman & Brizel Citation2001).
The FDA-approved drug, amifostine (also known as WR2721), is a thiol compound that acts primarily as a free radical scavenger to reduce the levels of oxidative radicals that would otherwise attack important cellular targets, such as deoxyribonucleic acid (DNA) and other cellular macromolecules (van der Vijgh & Peters Citation1994). The drug has well-documented, broad-spectrum radioprotective attributes (Santini Citation2001; Cassatt et al. Citation2002; Huang et al. Citation2002; Seed Citation2005; Seed et al. Citation2014). Radioprotectors other than amifostine, such as PrC-210, acting through similar mechanisms, are discussed in part III of this article series (Peebles et al. Citation2012; Soref et al. Citation2012; Copp et al. Citation2013). Of these protectors, amifostine is the only agent which has been approved by the FDA for limited indications and is currently gaining attention for development in combination with other agents to reduce its toxicity (Johnke et al. Citation2014; Seed et al. Citation2014; Singh et al. Citation2016a). Amifostine has been used successfully to prevent xerostomia (dry mouth) in head and neck cancer radiotherapy patients, which can otherwise cause permanent dry mouth due to the inclusion of the salivary glands (particularly the parotid glands) within the radiation field (Bourhis & Rosine Citation2002; Karacetin et al. Citation2004). This agent is also used to protect against renal toxicity due to cisplatinum, a DNA cross-linking agent that is also known to cause oxidative stress in patients with advanced ovarian cancer (Schuchter Citation1996; Ali & Al Moundhri Citation2006; Chirino & Pedraza-Chaverri Citation2009).
Unfortunately, amifostine has several clinically relevant limitations including: (1) a narrow time frame for administration (15–30 min) before each radiation dose; (2) its approval only for intravenous administration, although alternate routes (e.g. subcutaneous [sc]) have been explored previously, but still remain under investigation (Praetorius & Mandal Citation2008; Koukourakis et al. Citation2013); and (3) toxicity, including nausea, vomiting, somnolence, and hypotension. Recently, it has been demonstrated in a rodent model that radioprotective doses for amifostine to protect vital hematopoietic tissues of mice appear to lie between 25 and 50 mg/kilogram (kg); mature, lineage-restricted progenitors appear to be more responsive to the protective effects of low doses of amifostine than the more primitive, multipotential progenitors (Seed et al. Citation2014). Further, low doses of amifostine and γ-tocotrienol (GT3) exhibit an additive effect for radioprotection against cobalt-60 (60Co) γ-irradiation in CD2F1 mice (Singh et al. Citation2016a).
Palifermin (keratinocyte growth factor [KGF])
Palifermin is a recombinant N-terminal truncated form of KGF (also known as fibroblast growth factor-7) and acts in a paracrine manner to stimulate the reparative proliferation of epithelial cells from a variety of cell and tissue types including liver (hepatocytes), GI, lung (type II pneumocytes), transitional urothelial cells, hair follicle cells, and keratinocytes in all stratified squamous epithelia (Danilenko Citation1999; Farrell et al. Citation1999). KGF functions in the protection and repair of epithelial tissues through its cognate receptor fibroblast growth factor receptor-2B. Its protective action appears to be attributed to a combination of stimulating cell proliferation and protection against apoptosis (Finch et al. Citation2013). KGF treatment (5 mg/kg/day (d), intraperitoneal, once daily for 5 consecutive days before irradiation) has been shown to prevent radiation-induced (6 Gy [Gray] 60Co γ-radiation) intestinal damage in a C57Bl/6 mouse model (Cai et al. Citation2013). Under comparable treatment regimens, KGF has proven to effectively limit bacterial translocation across previously radiation-damaged villus epithelium of the GI tract of mice, therefore largely sparing these animals from potentially fatal, bacterial sepsis (Seed et al. unpublished observation).
A variety of preclinical studies suggest palifermin can ameliorate mucosal toxicity due to radiotherapy and chemotherapy (Finch et al. Citation2013). Palifermin was first approved by the FDA to prevent severe oral mucositis in patients receiving hematopoietic stem cell transplantation to treat hematological cancers (Vadhan-Raj et al. Citation2013; Johnke et al. Citation2014; Lauritano et al. Citation2014).
Erythropoietin (epoetin/epogen/procrit/darbepoetin/aransep)
Epoetin, or related drugs, Epogen, Procrit, Darbepoetin, and Aransep are all bioengineered analogs of erythropoietin (EPO) produced by DNA technology with FDA approval for use in humans. Their primary indication is the stimulation of erythropoiesis for the treatment of severe anemia (commonly associated with chronic renal dysfunction) arising from intense chemo- and/or radiation therapies (Covic & Abraham Citation2015; Gianoncelli et al. Citation2015; Agarwal & McBride Citation2016). However, these recombinants have not been specifically approved by the FDA for use in radiation casualty scenarios. Nevertheless, EPO was used for the victims of radiation exposure in the Tokai-mura, Japan and Henan Province, China (Nagayama et al. Citation2002; Liu et al. Citation2008).
Interleukin-3 (IL-3)
Studies in animal models have demonstrated that recombinant IL-3 stimulates myelopoiesis in both acute suppressive and normal steady state conditions of hematopoiesis; however, despite positive therapeutic attributes, the recombinant is not being actively pursued as an MCM for ARS or for chemotherapy-induced myelosuppression (Hammond et al. Citation1990; Lord et al. Citation1991). Similarly, the chimeric IL-3 receptor agonist, leridistim (myelopoietin), is not being actively pursued, despite the very promising results in ameliorating neutropenia (MacVittie et al. Citation2000). A phase III trial comparing leridistim and granulocyte-colony stimulating factor (G-CSF) showed the latter to be superior over the former in preventing chemotherapy-induced febrile neutropenia (Nabholtz et al. Citation2002). However, these agents have not been fully approved by the FDA for specific use in treating ARS. Nevertheless, similar to EPO, IL-3 has indeed been used with promising results in treating radiation accident victims of Soreq (Israel) and Nyasvizh (Belarus) (International Atomic Energy Agency [IAEA] Citation1993, Citation1996).
Interleukin-11 (IL-11, oprelvekin)
IL-11 is a multifunctional cytokine of the interleukin-6 (IL-6) family. It displays strong stimulation of megakaryocyte maturation, anti-inflammatory properties, and cytoprotective effects on GI crypt cells. Although IL-11 is indicated for preventing chemotherapy-induced thrombocytopenia, it is an important agent for the treatment of inflammatory bowel disease due to its anti-inflammatory and GI-protective properties. Recombinant Human IL-11 (rHuIL-11)’s therapeutic advantage following acute or chronic radiation injuries has been investigated previously as part of a combined recombinant cytokine treatment modality and has been promoted as such. Administration of IL-11 improves crypt survival and reduces GI mucosal injury after total-body irradiation (TBI) in mice (Potten Citation1995, Citation1996). Though IL-11 is well-tolerated in animals, significant adverse effects including fluid retention, multisystem organ failure, and pleural effusion have limited its clinical utility in humans. In brief, IL-11 has demonstrated efficacy as a radioprotector as well as a radiomitigator (Potten Citation1995; Burnett et al. Citation2013), but its structure and route of administration needs modification in order to avoid significant toxicity (Hauer-Jensen Citation2014).
Countermeasures approved by the FDA for the medical management of patients with internalized radionuclides
Four agents have been approved by the FDA for either the prevention or treatment of individuals exposed to internalized radionuclides: potassium iodide (KI; ThyroShield), Trisodium zinc diethylenetriaminepentaacetate (Zn-DTPA), Trisodium calcium diethylenetriaminepentaacetate (Ca-DTPA), and Prussian Blue (ferric hexacyanoferrate) (Centers for Disease Control and Prevention Citation2016). These agents block uptake, chelate, bind, or simultaneously chelate and reduce ‘body-burdens’ of internalized radionuclides. Despite their full FDA approval status, direct evidence that these drugs provide a significant clinical benefit to contaminated humans remains limited with the exception of KI.
Potassium iodide (KI, blocker)
Radioactive iodine (131I) enters the body through the lungs and GI tract, and is then avidly absorbed by the thyroid glands, especially during iodine-deficient periods. Ideally KI is administered prior to or shortly following the threat of exposure to 131I. The thyroid glands absorb this source of iodine, saturating the gland and preventing the incorporation of 131I and, in turn, significantly reducing carcinogenic risk. KI is readily available, relatively non-toxic, effective, and easily self-administered by oral route.
Following the Chernobyl reactor incident and the release of massive amounts of radioactive nuclides, KI was administered to the Polish population; this treatment was thought presumptively to be directly responsible for the greatly reduced incidence of thyroid cancers within the country, as opposed to other countries where such broad-scale KI treatments were not dispensed to the general population.
However, the major disadvantage of KI is that the protection it affords is restricted to the thyroid and associated late-arising pathologies, and the time-window for the effective administration of the protective agent is quite narrow (up to ∼3–4 hours (h)). Furthermore, KI is effective only in combating internal contamination with 131I. It does not protect against any other isotope, nor does it prevent or limit the uptake of 131I into any other organ. With time following exposure, the efficacy of KI diminishes, and several days following exposure, KI provides little to no benefit (American Thyroid Association Citation2013).
131I is released most frequently after incidents involving nuclear reactors (such as Chernobyl and Fukushima Daiichi). Although nuclear bomb detonations do indeed produce 131I fallout, the problem tends to be limited due to atmospheric dilution. Furthermore, of that 131I which is produced, approximately 10% will reach the earth’s surface as most will decay to stable xenon-131, thus limiting potential and sizable exposures to radioactive iodine following a nuclear detonation. Accordingly, and due to KI being readily available to the general public, the stockpiling of KI in the SNS was discontinued briefly, but only to be restarted as a result of the Fukushima Daiichi incident and associated pediatric concerns (Whitcomb et al. Citation2015).
Prussian blue (PB, binder)
PB is insoluble ferric hexacyanoferrate (Fe4[Fe(CN)6]3) and goes by the trade name of Radiogardase®. PB is an FDA-approved binding agent, stockpiled within the SNS for the treatment of internal contamination with radioactive cesium-137 (137Cs) and radioactive (and non-radioactive) thallium (Hussar Citation2005). It is used to reduce body burdens by blocking the absorption and uptake of the nuclides within the GI tract followed by the chelation and excretion of the contaminating isotopes. PB is well-tolerated when administered 1–3 g, 3 times per d orally (po). If PB treatments are initiated within hours following radionuclide contamination, the biological half-life of 137Cs can be reduced from ∼115 days to ∼40 days (67% reduction). PB efficacy can be improved by the simultaneous administration of other blocking agents and/or alginates.
A major disadvantage of PB rests in its limited ability to reduce body burdens to low levels when treatment initiation is greatly delayed after exposure. This disadvantage (associated with delayed PB treatment) is exacerbated by the limited availability of PB, both in terms of the amount and the number of locations in which it is stockpiled. PB is not intended for prophylactic use, and therefore the extent of 137Cs contamination needs to be quantified prior to treatment initiation. Patients receiving PB treatments need to be closely monitored (Yamamoto Citation2013).
Zn/Ca DTPA (chelators)
This nucleate chelating agent comes in two salts (Zn and Ca); both can be used to treat individuals contaminated with transuranic radionuclides (plutonium, americium, californium, curium, and neptunium) and/or rare earth radionuclides (cerium, lanthanum, promethium, scandium, and yttrium) (The Radiation Emergency Assistance Center/Training Site Citation2013). The FDA approved these agents in 2003 for radiation contingency events and made them available in SNS. These agents function by specific ionic binding (chelation) and enhanced excretion of nuclides in vivo.
The major disadvantage of this treatment is that it is only effective against a limited number of contaminating radionuclides. It is commonly accepted that DTPA should not be used to counter uranium contamination because it is not effective in removing uranium from the body, and it can enhance the nephrotoxic effects of uranium contamination. Following a mixed internal contamination with plutonium and uranium, DTPA can be used if there is less than 3 mg of uranium in systemic circulation; if the level is greater, pursuing DTPA treatments would have to be reassessed by the attending physicians in terms of relative health benefits versus health risks. However, if the quantity of contaminating plutonium is high enough to cause tissue reactions, then DTPA should be administered regardless of the level of uranium present (Kazzi et al. Citation2012). Additionally, DTPA availability is limited in terms of the quantity stockpiled at each site as well as the limited number of sites.
Countermeasures for radiation-induced emesis
In addition to countermeasures that protect from radiation injury or mitigate its effects, there are agents which can provide support if used appropriately. One of these agents is Kytril, which is used to treat emesis.
Granisetron (Kytril)
Granisetron (endo-N-(9-methyl-9-azabicylo-[3.3.1] non-3-yl)-1-methyl-1 H-indazole-3caroxamide hydrochloride) is a highly effective, anti-emetic agent commonly used clinically to reduce the toxic side-effects of radiation and/or chemotherapeutic treatments via the agent’s antagonistic effects on 5-hydroxytryptamine. Developed and marketed by Hoffman-LaRoche Inc., it received full FDA approval for use in humans in 2001 for this indication. The drug is not considered radioprotective, per se; however, it significantly minimizes initial performance-decrementing effects associated with the prodromal responses of acute radiation exposures. It is non-toxic and well-tolerated (Kehlet et al. Citation1996). Granisetron is effective when administered prophylactically or therapeutically when given in doses of 2 mg/d (oral), up to 14 d. Major disadvantages of the drug are that it will mask prodromal responses that serve as useful bioindicators of acute radiation exposure and it is limited in its protective actions; it does not protect normal tissue from radiation injury.
Countermeasures approved for late-arising injuries following either acute or chronic radiation exposures of various intensities
There is currently a scarcity of MCM that are fully approved by the FDA for use in mitigating the late-arising health consequences resulting from unwanted (non-clinical) radiation exposure. The reasons for the latter situation are many and complex, but largely related to the FDA’s prioritization of evaluating medicinals for acute radiation injury (as opposed to delayed-type injuries), especially those that may provide substantial and immediate survival benefits (Ainsworth et al. Citation1989; Gudkov et al. Citation2015). Such delayed health effects can be grouped into syndromes that arise either stochastically (e.g. cancer) or non-stochastically (e.g. tissue fibrosis). The most challenging medicinals to develop are those that would counter the expression of stochastic-type diseases long after an exposure event. Nevertheless, there are several agents that have proven to be extremely useful in providing effective anti-cancer protection. The previously described countering agent, KI, falls into this category as it serves to effectively counter the carcinogenic actions of internalized deposited radioactive iodides in the thyroid gland. KI’s efficacy is well-documented both experimentally and clinically. In addition, there are a number of potentially useful medicinals that could be designed or repurposed specifically for the prevention of ‘late-arising’ pathologies. Agents such as amifostine and angiotensin-converting enzyme inhibitors could serve this purpose as they have been either previously approved by the FDA for various clinical purposes or are currently being developed and experimentally tested in various stages of the FDA approval process. Agents currently under development are discussed under part III of this three-part article.
Countermeasures for acute injury in strategic national stockpile (SNS)
To date, there are three radiation countermeasures for ARS which have been procured for the SNS: granulocyte colony-stimulating factor (G-CSF), Polyethylene glycol (PEGylated) G-CSF, and granulocyte-macrophage-colony stimulating factor (GM-CSF) (U.S. Department of Health & Human Services Citation2013; Health and Human Services Citation2016). Descriptions of first two agents can be found in part I of this three-part article. GM-CSF is discussed below.
ARS countermeasures with U.S. FDA IND status
There are nine radiation countermeasures for ARS which have received FDA IND. Apart from 5-Androstenediol (5-AED), all the other agents are currently moving forward in the regulatory approval process (Singh et al. Citation2015b).
Genistein/BIO 300
Genistein (4′,5,7-trihydroxyisoflavone) is a soy-derived isoflavone with antioxidant, selective estrogen receptor activation, protein tyrosine kinase inhibition, and free radical scavenging activities (Akiyama et al. Citation1987; Valachovicova et al. Citation2004; Kruk et al. Citation2005; Wu & Chan Citation2007). Genistein can act as both a radioprotector and radiomitigator. BIO 300 is an aqueous nanosuspension consisting of synthetic genistein that is manufactured using a proprietary nanomilling process that significantly reduces the genistein particle size (Ha et al. Citation2013). This formulation has several key advantages, including improved bioavailability and ease of administration. Supporting experiments were executed with either genistein or BIO 300 as described below. Initial preclinical research carried out at the Armed Forces Radiobiology Research Institute using murine models established genistein’s radioprotective efficacy (Landauer et al. Citation2003). When administered via sc route of administration 24–12 h prior to TBI, a single dose of BIO 300 significantly improved 30-d survival in mice exposed to 9.25 Gy 60Co γ-radiation (Ha et al. Citation2013). Another study demonstrated that twice daily oral administration of BIO 300, initiating 6 d prior to exposure, significantly improved 30-d survival in mice exposed to 8.75 Gy 60Co γ-radiation (personal communication – Michael Kaytor, Humanetics Corporation). BIO 300 underwent further development as a radiomitigator for ARS and delayed effects of acute radiation exposure (DEARE) at Humanetics Pharmaceuticals (Humanetics Pharmaceuticals Citation2016). Extensive systematic efficacy screening was performed, assessing the ability of BIO 300 to mitigate lethal, radiation-induced pneumonitis/fibrosis in an established murine model of whole-thorax lung irradiation (Jackson et al. Citation2010, Citation2011, Citation2012). One study demonstrated that mice treated orally with BIO 300 (400 mg/kg), initiating 24 h following exposure to a single dose of whole-thorax lung irradiation and continuing once daily for 4–6 weeks, significantly improved 180-d survival compared to those that were untreated (personal communication – Michael Kaytor, Humanetics Corporation). Genistein, used as a mitigator, was reported in clinical trials to reduce adverse effects of chemotherapy and radiotherapy (Ahmad et al. Citation2010; Tacyildiz et al. Citation2010). In a murine model of ARS, genistein protected bone marrow (BM) progenitor cells from radiation-induced injury, leading to prevention of hematopoietic stem cell pool exhaustion, and thereby augmenting subsequent recovery of blood neutrophil and platelet levels (Landauer et al. Citation2003; Davis et al. Citation2007, Citation2008). BIO 300 administration also mitigated radiation-induced lung injury and improved overall lung function (personal communication – Michael Kaytor, Humanetics Corporation), and genistein increased murine survival after thoracic irradiation (Para et al. Citation2009). Genistein, administered after irradiation, reduced micronuclei in Lin− marrow cells and primary lung fibroblasts, suggesting a direct reduction of radiation-induced DNA damage (Day et al. Citation2008, Citation2013; Para et al. Citation2009; Mahmood et al. Citation2011). Several mechanisms have been proposed for the radioprotective efficacy of genistein, including activation of the DNA repair enzyme, growth arrest and DNA damage-inducible 45 (GADD45) (Oki et al. Citation2004; Caetano et al. Citation2006; Grace et al. Citation2007), quiescence of the cell cycle of Lin− cells in the G0/G1 phase in vivo (Tamulevicius et al. Citation2007; Davis et al. Citation2008), and the suppression of inflammation (Kim et al. Citation1998; Calveley et al. Citation2005; Comalada et al. Citation2006; Ha et al. Citation2013). Currently, this agent is under investigation at the Armed Forces Radiobiology Research Institute, Bethesda, MD, USA, to determine its efficacy as a radioprotector when administered by the sc or oral route in nonhuman primates (NHP).
CBLB502/entolimod
Salmonella typhimurium flagellin is a stable protein and natural activator of nuclear factor-κB which has been demonstrated to protect mice from lethal doses of TBI. A truncated version of this protein, CBLB502, retains the radioprotective efficacy and stability of flagellin without its immunogenic properties. CBLB502’s efficacy is due to its ability to bind to toll-like receptor-5, subsequently activating nuclear factor-κB signaling. Activation of this signaling pathway modulates the expression of several genes, including scavengers of reactive oxygen species, inhibitors of apoptosis, and a wide spectrum of cytokines (Burdelya et al. Citation2008). G-CSF and IL-6 have been identified as biomarkers to assess CBLB502 efficacy. Both biomarkers were found to play significant roles in mechanisms pertinent to enhanced survival rates in irradiated animals; administration of antibody to either G-CSF or IL-6 effectively abrogates the radioprotective efficacy of CBLB502 in irradiated rodents (Krivokrysenko et al. Citation2012).
CBLB502 has been found to be effective against both H- and GI-ARS in mice as well as NHP, and acts as both radioprotector and radiomitigator (Burdelya et al. Citation2008; Krivokrysenko et al. Citation2012). CBLB502 treatment reduces apoptosis and accelerates crypt regeneration in the GI tract. These characteristics make CBLB502 a potentially useful radiation countermeasure for both civilian and military personnel alike.
Like other radiation countermeasures, the FDA has granted IND, fast-track, and orphan drug status to CBLB502 as a radiation countermeasure. It is currently in clinical development for assessment of pharmacokinetics (PK), toxicity, and biomarkers; human safety studies indicated that CBLB502 was systemically well-tolerated and had biomarker results corresponding to previous biomarkers data from animal ARS models (Krivokrysenko et al. Citation2012, Citation2015; Cleveland BioLabs Inc. Citation2016). This safety, efficacy, and animal-to-human dose conversion data was sufficient, allowing progression with a pre-EAU application; this application is pending with the FDA.
GM-CSF/leukine/sargramostim
Leukine (Sargramostim/rhuGM-CSF) has FDA approval for five indications, three of which relate to the acceleration of neutrophil recovery in myelosuppressed patients (Gupta Citation2013; Sanofi-aventis U.S. LLC Citation2013). The use of GM-CSF to treat neutropenia in clinical settings supports its use in individuals exposed to non-therapeutic ionizing radiation. GM-CSF has been used to treat 14 radiation-exposed victims in three different accidents (dose range of 2.4–8.1 Gy), of whom nine survived (IAEA Citation1988, Citation1990; Gupta Citation2013). Similar to Neupogen and Neulasta, post-conditioning treatments with Leukine effectively enhances neutrophil recovery and serves to reduce the incidence of life-threatening infections. Although Leukine would certainly be utilized in cases of major radiological exposure contingencies and is well recognized to be effective in treating acute, radiation-induced hematopoietic injuries (as per radiation conditioning regimens for marrow transplantation), it has not been fully approved by the FDA for the specific treatment of ARS.
The efficacious nature of Leukine has been demonstrated in a number of animal models, both large and small, as well as in direct clinical application (Monroy et al. Citation1990; Reeves Citation2014). The radioprotective efficacy of GM-CSF has been evaluated in mice, canines, and NHP (Monroy et al. Citation1988; Atkinson et al. Citation1991; MacVittie et al. Citation1991; Clayton et al. Citation2016). Unlike G-CSF, GM-CSF is species-specific. Recombinant human and canine GM-CSF have been used in NHP and canine studies, respectively.
GM-CSF enhances the recovery of white blood cells in different strains of mice (Tanikawa et al. Citation1989; Atkinson et al. Citation1991; Waddick et al. Citation1991; Patchen et al. Citation1993), canines (MacVittie et al. Citation1991; Selig et al. Citation1991; Nothdurft et al. Citation1992), and NHP (Monroy et al. Citation1988; Farese et al. Citation1993; Neelis et al. Citation1997; Clayton et al. Citation2016) when administered either alone or in combination with other cytokines. Like G-CSF, GM-CSF administration enhanced neutrophil recovery, reduced the severity and duration of neutropenia, along with overall recovery of white blood cells, and enhanced granulocyte-macrophage colony-forming units in the BM. Results consistently support recovery from severe neutropenia as an advantage of using GM-CSF in H-ARS. In murine and canine studies where the effectiveness of G-CSF and GM-CSF have been compared side-by-side, G-CSF was found to be more effective in protecting irradiated animals (Waddick et al. Citation1991; Nash et al. Citation1994). Recently, GM-CSF has been evaluated as a radiomitigator in NHP and found to significantly improve survival when administered 48 h post-irradiation (7 μg/kg/d, sc, number of doses depended on neutrophil counts) with minimal supportive care (i.e. single antibiotic [Baytril] and no blood products) against two different doses of radiation (6.55 and 7.18 Gy) (Clayton et al. Citation2016). It is important to note that G-CSF was not effective when evaluated in the absence of full supportive care (blood products) (Gluzman-Poltorak et al. Citation2014b) but GM-CSF was found to be effective without blood products (Clayton et al. Citation2016). Sanofi is preparing a supplemental biological licensing application submission to the FDA.
AEOL 10150
AEOL 10150 (meso-porphyrin mimetic; C48H56C15MnN12) is a novel agent currently being developed as an MCM against the pulmonary effects of ARS and the DEARE (Gridley et al. Citation2007; Pearlstein et al. Citation2010; Zhang et al. Citation2012; Batinic-Haberle et al. Citation2013). It is a well-tolerated, broad-spectrum catalytic antioxidant capable of extending survival and minimizing acute pathology as demonstrated by studies administering sc daily treatments for 28 d to both mouse and NHP (Orrell Citation2006). Recent investigations determined that AEOL 10150 administration reduced lung injury and increased survival after 11.5 Gy whole thorax lung lethal dose (LD100/180) radiation exposure in the NHP (Garofalo et al. Citation2014). Daily administrations of AEOL 10150 (5 mg/kg, sc) initiated 24 h post-radiation exposure served to spare 26% of the treated animals from radiation-induced lung injury that would have been otherwise fatal (100% death in control cohort). Furthermore, CT scans revealed less pulmonary injury (pneumonitis, fibrosis, effusions), as assessed by quantitative radiography in the AEOL 10150-treated cohort at 60 d post-irradiation; the same AEOL 10150-treated animals required less dexamethasone and had relatively lower levels of transforming growth factor-β1 circulating in their plasma compared with control animals. These studies indicate that AEOL 10150 reduces clinical, radiographic, anatomic, and molecular evidence of radiation-induced lung injury.
Effective dosing for the drug, AEOL 10150, has been recently explored using radiation-induced lung injury murine model (whole-thorax lung exposure with 14.6 Gy) in CBA/J mice (Murigi et al. Citation2015). Drug treatments at a daily dose of 25 mg/kg for a total of 28 d, starting 24 h after whole-thorax lung irradiation, was observed to be optimal, with irradiated animals exhibiting improved lung function as well as improved rates of survival. Beyond using this drug to counter high radiation dose pulmonary injury, this agent is also being investigated as a potential countermeasure for GI-ARS (Aeolus Pharmaceuticals Citation2012).
OrbeShield®/beclomethasone 17,21-dipropionate (BDP)
BDP is a potent, topically-active corticosteroid currently under development as an orally-administered radiation countermeasure for GI-ARS (Soligenix Inc Citation2016). It offers potent anti-inflammatory effects with minimal toxicity compared with other systemic effective corticosteroids. BDP showed significant survival benefit in a canine model of GI sub-syndrome (Soligenix Inc Citation2016). In this model, canines received TBI, followed by autologous BM administration and supportive care, and coupled to experimental treatments with the test agent or placebo. This study had three groups: one control group and two BDP-treated groups (each with a different treatment schedule). Both BDP-treated groups had a higher number of survivors compared to the control, suggesting that BDP can rescue inflamed tissues of the GI mucosa after irradiation and can improve survival when treatment is initiated as late as 24 h post-irradiation (Georges et al. Citation2012). OrbeShield® has received FDA IND, orphan drug, and fast-track statuses. This candidate countermeasure for GI-ARS has been formulated as a single agent with two tablets for oral administration; one tablet is intended to release BDP in the proximal, and the other in the distal portions of the GI tract. No reports exist regarding its toxicity and PK in humans. BDP is available in the U.S. as the active pharmaceutical ingredient in a nasal spray and inhaler used for the treatment of asthma and allergic rhinitis.
Interleukin-12 (IL-12)/HemaMax
Human recombinant IL-12 has been shown to enhance mouse survival when administered as a single dose, either 24 h prior or 1 h or 24 h after TBI. Neumedicines is developing IL-12 as a radiomitigator for the treatment of H-ARS and is preparing a biological licensing application submission to the FDA (Neumedicines Citation2014). Allometrically equivalent doses of mouse and human IL-12 have comparable PK; IL-12 administration significantly increased rodent and NHP survival, when injected 24 h post-irradiation without supportive care (Gluzman-Poltorak et al. Citation2014a).
A clinical phase-Ib safety study evaluating the predicted effective human dose has indicated IL-12 is safe and well-tolerated in humans. Phase II equivalent data (good laboratory practice, randomized, and double-blinded) demonstrated that single administration of human IL-12 to NHP significantly enhanced survival and reduced neutropenia/thrombocytopenia when injected 24 h post-irradiation. Injection of IL-12 promotes immune functions, multi-lineage hematopoietic recovery, and possibly GI functions within acutely irradiated mice and NHP (Gluzman-Poltorak et al. Citation2014a, Citation2014b, Citation2015).
IL-12 has also been evaluated in a combined injury murine model (sublethal TBI with 137Cs γ-rays and β-particle irradiation) in order to test whether the recombinant could protect the cutaneous system from combined injury stemming from acute exposures to two different qualities of ionizing radiation. The added clinical complication associated with β-particle burns resulted in a breakdown of skin integrity and loss of vital barrier-function within immunocompromised mice. When IL-12 was administered intradermally 48 h post-irradiation, it reduced transepidermal water loss and the ultimate size of burn lesions, while also retaining essential cutaneous dendritic cells (Gerber et al. Citation2015). These results suggest that IL-12 is also a potential mitigator of injuries arising from exposures to a combination of radiation qualities and it may provide additional support for further development of this countermeasure.
Myeloid progenitor cells (MPC)
MPC can improve survival of mice against high doses of radiation. MPC have been evaluated for use as a bridging therapy for radiation injuries and to mitigate the effects of lethal doses of 60Co γ-irradiation and X-rays in different strains of mice (Singh et al. Citation2012a). Different cell numbers of pooled allogeneic MPC from C57Bl/6, FVB, and AKR mice were transfused intravenously into haplotype-mismatched recipient BALB/c or CD2F1 mice at various times after irradiation to assess their effect on survival. Results demonstrated that cryopreserved allogeneic MPC significantly improved survival in both strains of mice irradiated with lethal doses of 60Co γ-radiation (CD2F1, 9.2 Gy) and X-ray exposures (BALB/c, 9 Gy) that are known to cause ARS in hematopoietic tissues (Singh et al. Citation2012a). The survival benefit was MPC-dose-dependent and significant even when MPC administration was delayed up to 7 d post-irradiation. Additionally, MPC administration decreased deaths from ARS at radiation doses up to 15 Gy (60Co γ-radiation, CD2F1 mice). Exposure levels of such magnitude can cause mice to succumb to multi-organ failure. Even at doses of up to 14 Gy 60Co γ-radiation, MPC administration could be delayed up to 5 d in CD2F1 mice and still provide significant benefit to 30-d survival. However, as interesting as these studies are, additional studies with extended observational periods are critically needed in order to rule any/all potential late-arising pathologies that stem from graft versus host disease. To study the structural integrity of the GI tract, mice received MPC treatment after doses of radiation capable of causing GI injury. GI tissues were collected at different time-points after irradiation and analyzed for architecture and surviving crypts as well as villus height and number. The histopathology of GI tissue from irradiated and MPC-injected mice demonstrated improved gut architecture compared to untreated controls. The effect of MPC administration on bacterial translocation from the GI to the heart, spleen, and liver in irradiated mice was also investigated by bacterial colony cultures and measuring endotoxin levels in serum samples. MPC decreased bacterial infection and inhibited endotoxin levels in serum. In vivo studies show that allogeneic myeloid progenitors give rise to mostly myeloid and erythroid cells that transiently engraft and contribute to mice protection. In brief, the results of these studies tentatively support administration of MPC as a bridging therapy, not only for the hematopoietic system, but also for GI tissue. MPC improve the structural integrity of GI tissue and inhibit bacterial translocation from the GI tract of mice following acute, potentially lethal radiation. The risk of long-term pathology arising from graft versus host disease remains ill-defined. This caveat associated with the eventual use of this therapeutic approach needs to be fully investigated and reported.
Cellerant Therapeutics, Inc. (San Carlos, CA, USA) is developing a cell-based therapy containing human myeloid progenitors, CLT-008 (Cellerant Therapeutics Citation2013). Derived from adult hematopoietic stem cells, CTL-008 can produce functional granulocytes, red blood cells, and platelets in vivo. It is currently under a phase I clinical trial. This product has received U.S. FDA IND status (Cellerant Therapeutics – personal communication).
ON01210/Ex-RAD
ON01210 (a chlorobenzylsulfone derivative known as Ex-RAD) is a novel, small-molecule kinase inhibitor under development as a radiation countermeasure. Ex-RAD provided significant protection against 60Co γ-irradiation when administered sc (500 mg/kg) to C3H/HeN mice 24 h and 15 min before irradiation, with an estimated dose reduction factor of 1.16 (Ghosh et al. Citation2009). In another study, Ex-RAD showed a significant survival benefit after prophylactic oral administration (Suman et al. Citation2012a). NHP studies have been recently initiated, with interim results obtained, but final analyses and reporting still pending (i.e. awaiting public release by the company, Onconova Therapeutics).
This drug accelerated the recovery of peripheral blood elements in irradiated mice when administered either sc or orally (Ghosh et al. Citation2012; Suman et al. Citation2012a). In addition, Ex-RAD-treated mice (either through the po or sc route) contained higher numbers of granulocyte-macrophage colony-forming unit than vehicle-treated mice. BM obtained from irradiated mice indicated that Ex-RAD protected cells from radiation-induced apoptosis after exposure to 60Co γ-radiation (Ghosh et al. Citation2012). Ex-RAD also assists in the recovery of the GI system. Mice treated with Ex-RAD had a higher number of crypts surviving after acute radiation exposure when compared to untreated irradiated controls (Ghosh et al. Citation2012). These effects may be due in part to signaling pathways that are affected by Ex-RAD. Attenuation of ataxia telangiectasia mutated-tumor protein 53-mediated DNA damage response by Ex-RAD contributes to the mitigation of radiation-induced hematopoietic toxicity (Suman et al. Citation2012b). Recently, Kang et al. demonstrated that Ex-RAD manifests its protective effects through the up-regulation of phosphatidylinositol 3-kinase (PI3K)/Akt (Serine/threonine kinase, protein kinase B) pathways in cells exposed to radiation (Kang et al. Citation2013). Ex-RAD has been granted FDA IND status. Oral efficacy has been demonstrated with Ex-RAD using experimental mice; as such, effective oral administration makes Ex-RAD a potentially interesting and useful countermeasure that might be used to treat first responders as well as at-risk civilian populations in the event of a nuclear accident. However, prior to promoting the future utility of this agent, results of essential studies using large animal models are clearly required.
5-Androstenediol (5-AED)/Neumune
5-AED (androst-5-ene-3β,17β-diol) was the first radiation countermeasure for ARS to receive FDA IND status. It was evaluated as both a radioprotector and radiomitigator in mice and NHP (Whitnall et al. Citation2002; Stickney et al. Citation2006, Citation2007). Single administration of 5-AED, before or after a lethal dose of TBI, enhanced murine survival, though post-irradiation drug administration was less effective than pre-irradiation administration (Whitnall et al. Citation2000, Citation2002, Citation2005). The radiomitigator efficacy of 5-AED was confirmed with both mice and NHP (Loria et al. Citation2000; Stickney et al. Citation2006). 5-AED significantly increased G-CSF and IL-6 in mice; findings that perhaps suggest the agent’s molecular mode of protective action. Neutralization studies demonstrated that G-CSF was partially responsible for its efficacy (Singh et al. Citation2005). 5-AED stimulated B-cell lymphoma-2 (BCL-2) and BCL-2-associated X protein, up-regulated cyclin-dependent kinase inhibitor 1A and DNA damage-binding protein-1 expression, and reduced DNA strand breakage in splenocytes of irradiated mice (Grace et al. Citation2012). Clinical trials suggest that intraperitoneal administration of 5-AED may be a safe and effective method to stimulate innate immunity and alleviate ARS-associated neutropenia and thrombocytopenia (Stickney et al. Citation2010). Even with the above promising results, development of this countermeasure remains uncertain. The Department of Health and Human Services decided not to purchase 5-AED (Neumune) from Hollis-Eden citing that it was ‘technically unacceptable’ and not in competitive range. As a result, Hollis-Eden (now Harbor Biosciences) decided to discontinue development of 5-AED (Beaulieu Citation2007; Davies Citation2007).
ARS countermeasures under advanced development
Advanced development implies the use of large animals for testing and associated tests related to pharmacology scaling (to the human). There are a few radiation countermeasures that have demonstrated efficacy in large animal models in addition to small animals but have not achieved IND status yet.
γ-tocotrienol (GT3)
GT3 is one of eight isomers of vitamin E (a group of compounds also known as tocols); it has received great attention by researchers over the last 10 years and seems to be one of the more promising radioprotective tocols tested to date. The initial preclinical research carried out at the Armed Forces Radiobiology Research Institute using murine models established GT3’s radioprotective efficacy; this work supported a U.S. patent application and eventual patent approval (Henry et al. Citation2004; Kumar et al. Citation2006). When administered 24 h prior to 60Co γ-irradiation, GT3 significantly protected mice against radiation doses as high as 11.5 Gy. The dose reduction factor of GT3, when administered as a radioprotector (24 h before irradiation, 200 mg/kg, sc), is 1.29 in mice. GT3 treatment enhanced hematopoietic recovery in the peripheral blood, accelerated recovery of hematopoietic progenitors in BM of irradiated mice, and induced high levels of G-CSF and IL-6 (Kulkarni et al. Citation2013; Singh & Hauer-Jensen Citation2016). Mouse survival studies with GT3 suggested the most effective time for administration is 24 h before irradiation, possibly due to the induction of vital hematopoietic cytokines during that time frame. Prophylactic GT3 administration demonstrated a downregulation of pro-apoptotic genes and up-regulation of anti-apoptotic genes at 4 and 24 h post-irradiation (Suman et al. Citation2013). Jejuna crypt analysis, using TUNEL staining, demonstrated that GI tissues were protected by GT3 treatment. Similar to several other countermeasures, it has been demonstrated that the administration of G-CSF antibody completely abrogates the radioprotective efficacy of GT3 (Kulkarni et al. Citation2013).
GT3 has antioxidant properties common to other tocols (Singh & Hauer-Jensen Citation2016). GT3 decreases vascular oxidative stress after irradiation, reduces intestinal damage, and enhances the recovery of soluble biomarkers of endothelial function (Berbee et al. Citation2009). Radiation exposure reduces tetrahydrobiopterin in the lungs which can lead to vasoconstriction and neurovascular dysfunction; GT3 administration reverses this effect. Both GT3 and tetrahydrobiopterin supplementation inhibited post-irradiation vascular peroxynitrite production (Berbee et al. Citation2011a). Berbee et al. reported an additive effect when combined pentoxifylline (methylxanthine-derived phosphodiesterase inhibitor) and GT3. This combination significantly enhanced survival of mice against 60Co γ-irradiation compared with either agent administered alone (Berbee et al. Citation2011b). The pentoxifylline and GT3 combination protected all mice against TBI doses as high as 12 Gy. Radiation-modulated micro-ribonucleic acid (microRNA, miRNA), discovered to be involved in hematopoiesis, exhibited dose- and time-dependent levels of expression in GT3-pretreated spleen tissues, whereas vehicle-treated tissues did not. Further, GT3 reduced serum levels of McDonough feline sarcoma viral oncogene homolog (FMS)-related tyrosine kinase 3 ligand (Ghosh et al. Citation2016). GT3 pretreatment reduced DNA double-strand break formation in human umbilical vein endothelial cells as well as mouse BM cells after irradiation; GT3 also reduced radiation-induced cytogenetic damage by most likely affecting double-strand DNA repair protein expression (Pathak et al. Citation2016).
GT3 has been investigated for its radioprotective efficacy against 60Co γ-irradiation using an NHP model with encouraging results (Singh et al. Citation2016b; Singh & Olabisi Citation2017). GT3 helped the recovery of radiation-induced thrombocytopenia and neutropenia compared to the vehicle controls; these results were especially significant after lethal exposures to 5.8 or 6.5 Gy 60Co γ-TBI. The stimulatory effect of GT3 on platelets and neutrophils was positively and directly correlated with radiation dose; the 75 mg/kg dose was more effective compared to a dose of 37.5 mg/kg. GT3 was also effective against 6.5 Gy whole-body γ-irradiation for improving platelets and neutrophils. Moreover, a single dose of GT3, without any supportive care, was comparable to multiple doses of Neupogen and two doses of Neulasta with full supportive care (including blood products) in terms of improving hematopoietic recovery in the NHP. These results demonstrate that GT3 may well serve as an extremely useful radioprotector for use in humans, particularly for first responders and military personnel (Singh & Hauer-Jensen Citation2016). Recently, we demonstrated that three miRNAs correlated with the radioprotective efficacy of GT3; these miRNA in GT3-treated and irradiated NHPs resembled the unirradiated animals: miR-30a, miR-126 and miR-375 (Fendler et al. Citation2017). In brief, GT3 is a promising radioprotector and needs to be further developed for U.S. FDA approval for ARS.
B-190 (indralin)
Indralin is an adrenoreceptor agonist, and its radioprotective efficacy is mediated through activation of α1(B)-adrenoreceptors, which are members of a family of G-protein-coupled receptors. The radioprotective efficacy of indralin has been studied in seven species of experimental animals: mice, rats, hamsters, guinea pigs, rabbits, canine, and NHP, and demonstrated consistent efficacy (Vasin et al. Citation1996, Citation2014). Recently, its efficacy was investigated in NHP (2–3 years old, weighing 2.1–3.5 kg) using whole-body 60Co γ-radiation. Animals were exposed to a dose of 6.8 Gy (100% lethality in control over 30 d) (Vasin et al. Citation2014). Indralin (40–120 mg/kg) was administered intramuscularly 5 min before irradiation. Indralin at a dose of 120 mg/kg protected five out of six NHP compared with the control group, in which all ten animals died. Indralin decreased radiation-induced injuries in NHP, mitigating the effects of ARS. The therapeutic index for indralin, the ratio of the maximum tolerated dose to the average effective dose, was ten. These results suggest that indralin has noteworthy radioprotective efficacy and has a high therapeutic index. However, the protective ‘window’ for drug administration remains uncertain and needs to be clarified.
Conclusions and future directions
To date, only a limited number of radiation countermeasures have been fully approved by the U.S. FDA for the purpose of limiting the health hazards arising from unwanted ionizing radiation exposures. Currently, the FDA has successfully approved a mere six agents that are designed and developed to protect against or to mitigate irradiation-associated bodily injuries. Four of these agents, KI (ThyroShield), Zn-DTPA, Ca-DTPA, and Prussian Blue are agents that bind, chelate, or block internalized radionuclides so that body-burdens of radioisotope can be minimized (Singh et al. Citation2015b). Two growth factors, G-CSF and PEGylated G-CSF, were approved by the FDA in 2015 as radiomitigators for H-ARS (National Institute of Allergic and Infectious Diseases Citation2015; U.S. Food and Drug Administration Citation2015). Two additional agents, amifostine and palifermin, have been approved by the FDA for very narrow clinical indications. Out of the above agents, only amifostine is classified as a radioprotector. This agent, along with several other agents, have rather poor toxicity and safety profiles, a common limitation with many of the small molecular agents that are currently being pursued in the area of radiation countermeasures (Seed Citation2005; Brizel Citation2007). There are a few additional cytokines and growth factors approved by FDA for limited indications arising from radiotherapy and chemotherapy-induced neutropenia in cancer patients: EPO, IL-3, and IL-11.
Nine radiation countermeasures have received FDA IND status and three of those injury-mitigating agents, G-CSF, PEGylated G-CSF, and GM-CSF, have already been procured by the U.S. government for the SNS. Additionally, the FDA is currently reviewing an emergency use authorization application from Cleveland BioLabs, Inc. for CBLB502 as a radioprotective/radiomitigative agent. Further, there are two radiation countermeasures, GT3 and B-190, which are promising and moving well. GT3 significantly enhanced, in a dose-dependent manner, the recovery of radiation-induced thrombocytopenia and neutropenia compared to the vehicle-treated controls and was effective against 5.8 and 6.5 Gy whole body γ-irradiation. Most significantly and in terms of improving hematopoietic recovery following acute irradiation, a single dose of GT3 without any supportive care was equivalent to multiple doses of G-CSF or to two doses of PEGylated G-CSF with full supportive care in the NHP. In a mass casualty scenario, hospital-based medical care facilities definitely would be limited. Therefore, an ideal radiation countermeasure should be effective in the absence of hospital-based medical care, thus adding significance to the recent report of GT3’s efficacy in NHP in the absence of supportive care. GT3 is regarded by the U.S. FDA as ‘generally recognized as safe (GRAS)’ when used orally as a nutritional supplement at lower doses than intended for use as a countermeasure. Currently, GT3 is being evaluated in the NHP using a large sample size to consolidate the findings. However, and much like many other promising radiation injury countering drugs under study, safety is of paramount concern, and additional clinical safety trials are clearly needed for the proposed alternate routes of drug administration.
Countermeasures are typically tested in rodents using 30-d survival as the major endpoint and in a 60-d survival endpoint in canines or in NHP. These time intervals have been selected to reflect the ability of the agent to provide protection against or mitigate ARS following TBI or partial-body irradiation. Delayed or late effects of whole-body or partial-body exposures, including survivors of ARS, is gaining interest in the field of radiation countermeasure investigation. The more prominent of the latter medicinals currently being researched as countermeasures for radiation-associated ‘late effects’ (or DEARE) are listed and discussed in part III of the series (e.g. ACE inhibitors and pentoxifylline for late-arising pulmonary syndromes). These effects may be of critical importance as there would surely be many more long surviving individuals with moderate to low radiation exposure histories in any given radiological or nuclear exposure contingency. Further, there are a number of other radiosensitive organ systems of the body besides the well-studied hematopoietic and GI, including the neurovascular/cardiovascular systems, pulmonary, cutaneous, and urogenital systems. All of the latter organ systems are in need of more thorough study in terms of developing safe and effective, injury-countering medicinals. Also, the efficacy of radioprotectors/mitigators in the setting of exposure to particulate radiation (in particular to high LET irradiation), such as neutrons, protons, and heavier ions, is another largely unexplored area since the majority of studies utilize low LET γ-radiation or X-rays. Finally, the use of combinations of different agents has not been extensively tested. Thus, combinations of countermeasures with different mechanisms of action may be superior to single agents in the same manner that combination cancer chemotherapy is often superior to treatment with individual agents (Singh et al. Citation2016a).
Notes on contributors
Vijay K. Singh is a well-recognized radiation biologist and is involved in the advanced development of radiation countermeasures for acute radiation syndrome. The primary focus of his research is to understand the mechanism of action of radiation countermeasures, identify their efficacy biomarkers, and elucidate the pathways involved in radiation injury.
Melissa Garcia is a researcher who obtained her degree from the University of Maryland. She is currently investigating the mechanisms of radiation injury and evaluating the efficacy of several potential radiation countermeasures. Her research focuses on the development of countermeasures to combat H-ARS and GI-ARS, utilizing large animal models.
Thomas M. Seed currently serves in a consulting capacity for both public and private organizations alike with vested interests in the nature of ionizing radiation injuries and agents and methods designed to counter those injuries.
Acknowledgements
The opinions or assertions contained herein are the private views of the authors and are not necessarily those of the Armed Forces Radiobiology Research Institute, the Uniformed Services University of the Health Sciences, or the Department of Defense. Mention of specific therapeutic agents does not constitute endorsement by the U.S. Department of Defense, and trade names are used only for the purpose of clarification. We apologize to those having contributed substantially to the topics discussed herein that we were unable to cite because of space constraints.
Disclosure statement
The senior author (VKS), along with the co-author (MG) report no conflicts of interest of either a financial nor proprietary nature. The authors (VKS, MG, TMS) alone are responsible for the content and writing of this manuscript. The last co-author (TMS) likewise has no material interest in any of the companies/products listed, but has previously provided consultation services for various private and public organizations with vested interests in various radiological issues. Products of two organizations (Cleveland Bio Labs, Inc. and CuraGen Corporation) are mentioned in these articles. This co-author (TMS) was also part of the U.S. Department of Defense-based patents for two specific classes of radioprotective agents, i.e. isoflavonoids and the tocotrienols; these agents are also listed.
Funding
The authors gratefully acknowledge the research support from the National Institute of Allergy and Infectious Diseases [AAI-12044-0000-05000, Work Plan G] and Congressionally Directed Medical Research Programs [W81XWH-15-C-0117, JW140032] of U.S. Department of Defense to VKS.
References
- Aeolus Pharmaceuticals. 2012. Aeolus Pharmaceuticals announces AEOL 10150 reduces lung damage after Neupogen treatment following radiation exposure. Mission Viejo (CA). Available at: http://www.aolsrx.com/news/press-releases/detail/924/aeolus-pharmaceuticals-announces-aeol-10150-reduces-lung [accessed 3 July 2014].
- Agarwal AB, McBride A. 2016. Understanding the biosimilar approval and extrapolation process – a case study of an epoetin biosimilar. Crit Rev Oncol Hematol. 104:98–107.
- Ahmad IU, Forman JD, Sarkar FH, Hillman GG, Heath E, Vaishampayan U, Cher ML, Andic F, Rossi PJ, Kucuk O. 2010. Soy isoflavones in conjunction with radiation therapy in patients with prostate cancer. Nutr Cancer. 62:996–1000.
- Ainsworth EJ, Afzal SM, Crouse DA, Hanson WR, Fry RJ. 1989. Tissue responses to low protracted doses of high LET radiations or photons: early and late damage relevant to radio-protective countermeasures. Adv Space Res. 9:299–313.
- Akiyama T, Ishida J, Nakagawa S, Ogawara H, Watanabe S, Itoh N, Shibuya M, Fukami Y. 1987. Genistein, a specific inhibitor of tyrosine-specific protein kinases. J Biol Chem. 262:5592–5595.
- Ali BH, Al Moundhri MS. 2006. Agents ameliorating or augmenting the nephrotoxicity of cisplatin and other platinum compounds: a review of some recent research. Food Chem Toxicol. 44:1173–1183.
- American Thyroid Association. 2013. Nuclear radiation and the thyroid. Available at: http://www.thyroid.org/wp-content/uploads/patients/brochures/NuclearRadiation_brochure.pdf [accessed 1 Sept 2016].
- Atkinson K, Matias C, Guiffre A, Seymour R, Cooley M, Biggs J, Munro V, Gillis S. 1991. In vivo administration of granulocyte colony-stimulating factor (G-CSF), granulocyte-macrophage CSF, interleukin-1 (IL-1), and IL-4, alone and in combination, after allogeneic murine hematopoietic stem cell transplantation. Blood. 77:1376–1382.
- Batinic-Haberle I, Tovmasyan A, Roberts E, Vujaskovic Z, Leong KW, Spasojevic I. 2013. SOD therapeutics: latest insights into their structure-activity relationships and impact on the cellular redox-based signaling pathways. Antioxid Redox Signal. 20:2372–2415.
- Beaulieu D. 2007. US government rejects Hollis-Eden’s Neumune proposal [Online]. FirstWorld Pharma. Available at: http://www.firstwordpharma.com/node/112458#axzz4hMkBsGLh [accessed 17 May 2017].
- Berbee M, Fu Q, Boerma M, Pathak R, Zhou D, Kumar KS, Hauer-Jensen M. 2011a. Reduction of radiation-induced vascular nitrosative stress by the vitamin E analog gamma-tocotrienol: evidence of a role for tetrahydrobiopterin. Int J Radiat Oncol Biol Phys. 79:884–891.
- Berbee M, Fu Q, Boerma M, Wang J, Kumar KS, Hauer-Jensen M. 2009. Gamma-Tocotrienol ameliorates intestinal radiation injury and reduces vascular oxidative stress after total-body irradiation by an HMG-CoA reductase-dependent mechanism. Radiat Res. 171:596–605.
- Berbee M, Fu Q, Garg S, Kulkarni S, Kumar KS, Hauer-Jensen M. 2011b. Pentoxifylline enhances the radioprotective properties of gamma-tocotrienol: differential effects on the hematopoietic, gastrointestinal and vascular systems. Radiat Res. 175:297–306.
- Bourhis J, Rosine D. 2002. Radioprotective effect of amifostine in patients with head and neck squamous cell carcinoma. Semin Oncol. 29:61–62.
- Brizel DM. 2007. Pharmacologic approaches to radiation protection. J Clin Oncol. 25:4084–4089.
- Burdelya LG, Krivokrysenko VI, Tallant TC, Strom E, Gleiberman AS, Gupta D, Kurnasov OV, Fort FL, Osterman AL, Didonato JA, et al. 2008. An agonist of Toll-like receptor 5 has radioprotective activity in mouse and primate models. Science. 320:226–230.
- Burnett AF, Biju PG, Lui H, Hauer-Jensen M. 2013. Oral interleukin 11 as a countermeasure to lethal total-body irradiation in a murine model. Radiat Res. 180:595–602.
- Caetano B, Le Corre L, Chalabi N, Delort L, Bignon YJ, Bernard-Gallon DJ. 2006. Soya phytonutrients act on a panel of genes implicated with BRCA1 and BRCA2 oncosuppressors in human breast cell lines. Br J Nutr. 95:406–413.
- Cai Y, Wang W, Liang H, Sun L, Teitelbaum DH, Yang H. 2013. Keratinocyte growth factor pretreatment prevents radiation-induced intestinal damage in a mouse model. Scand J Gastroenterol. 48:419–426.
- Calveley VL, Khan MA, Yeung IW, Vandyk J, Hill RP. 2005. Partial volume rat lung irradiation: temporal fluctuations of in-field and out-of-field DNA damage and inflammatory cytokines following irradiation. Int J Radiat Biol. 81:887–899.
- Cassatt DR, Fazenbaker CA, Bachy CM, Hanson MS. 2002. Preclinical modeling of improved amifostine (Ethyol) use in radiation therapy. Semin Radiat Oncol. 12:97–102.
- Cellerant Therapeutics. 2013. CLT-008 myeloid progenitor cells [Online]. Cellerant therapeutics; Available at: http://www.cellerant.com/ [accessed 4 July 2016].
- Centers for Disease Control and Prevention. 2016. Emergency preparedness and response. Available at: http://emergency.cdc.gov/radiation/countermeasures.asp [accessed 1 Sept 2016].
- Chirino YI, Pedraza-Chaverri J. 2009. Role of oxidative and nitrosative stress in cisplatin-induced nephrotoxicity. Exp Toxicol Pathol. 61:223–242.
- Clayton NP, Charpentier EJJ, LaCasse ER, Khan-Malek RC, Keutzer JM. 2016. Sargramostim significantly improved the mortality rate at Day 60 in a non-human primate model of hematopoietic acute radiation syndrome with minimal supportive care when administered 48 h after total body irradiation. Poster presented at: 42nd Conference of the European Radiation Research Society; Amsterdam, Netherlands.
- Cleveland BioLabs Inc. 2016. Entolimod (CBLB502) biodefense. Cleveland BioLabs, Inc. Available at: http://www.cbiolabs.com/ [accessed 10 June 2016].
- Comalada M, Ballester I, Bailon E, Sierra S, Xaus J, Galvez J, de Medina FS, Zarzuelo A. 2006. Inhibition of pro-inflammatory markers in primary bone marrow-derived mouse macrophages by naturally occurring flavonoids: analysis of the structure-activity relationship. Biochem Pharmacol. 72:1010–1021.
- Copp RR, Peebles DD, Soref CM, Fahl WE. 2013. Radioprotective efficacy and toxicity of a new family of aminothiol analogs. Int J Radiat Biol. 89:485–492.
- Covic A, Abraham I. 2015. State-of-the-art biosimilar erythropoietins in the management of renal anemia: lessons learned from Europe and implications for US nephrologists. Int Urol Nephrol. 47:1529–1539.
- Culy CR, Spencer CM. 2001. Amifostine: an update on its clinical status as a cytoprotectant in patients with cancer receiving chemotherapy or radiotherapy and its potential therapeutic application in myelodysplastic syndrome. Drugs. 61:641–684.
- Danilenko DM. 1999. Preclinical and early clinical development of keratinocyte growth factor, an epithelial-specific tissue growth factor. Toxicol Pathol. 27:64–71.
- Davies J. 2007. U.S. won’t buy Hollis-Eden treatment for radiation. Union Turbine. Available at: http://legacy.sandiegouniontribune.com/news/business/biotech/20070317-9999-1b17hollis.html [accessed 17 May 2017].
- Davis TA, Clarke TK, Mog SR, Landauer MR. 2007. Subcutaneous administration of genistein prior to lethal irradiation supports multilineage, hematopoietic progenitor cell recovery and survival. Int J Radiat Biol. 83:141–151.
- Davis TA, Mungunsukh O, Zins S, Day RM, Landauer MR. 2008. Genistein induces radioprotection by hematopoietic stem cell quiescence. Int J Radiat Biol. 84:713–726.
- Day R, Barshishat-Kupper M, Mog S, McCart E, Prasanna P, Davis T, Landauer M. 2008. Genistein protects against biomarkers of delayed lung sequelae in mice surviving high-dose total body irradiation. J Radiat Res. 49:361–372.
- Day RM, Davis TA, Barshishat-Kupper M, McCart EA, Tipton AJ, Landauer MR. 2013. Enhanced hematopoietic protection from radiation by the combination of genistein and captopril. Int Immunopharmacol. 15:348–356.
- Farese AM, Williams DE, Seiler FR, MacVittie TJ. 1993. Combination protocols of cytokine therapy with interleukin-3 and granulocyte-macrophage colony-stimulating factor in a primate model of radiation-induced marrow aplasia. Blood. 82:3012–3018.
- Farrell CL, Rex KL, Kaufman SA, Dipalma CR, Chen JN, Scully S, Lacey DL. 1999. Effects of keratinocyte growth factor in the squamous epithelium of the upper aerodigestive tract of normal and irradiated mice. Int J Radiat Biol. 75:609–620.
- Fendler W, Malachowska B, Meghani K, Konstantinopoulos PA, Guha C, Singh VK, Chowdhury D. 2017. Evolutionarily conserved serum microRNAs predict radiation-induced fatality in nonhuman primates. Sci Transl Med. 9:eaal2408.
- Finch PW, Mark Cross LJ, McAuley DF, Farrell CL. 2013. Palifermin for the protection and regeneration of epithelial tissues following injury: new findings in basic research and pre-clinical models. J Cell Mol Med. 17:1065–1087.
- Garofalo MC, Ward AA, Farese AM, Bennett A, Taylor-Howell C, Cui W, Gibbs A, Prado KL, Macvittie TJ. 2014. A pilot study in rhesus macaques to assess the treatment efficacy of a small molecular weight catalytic metalloporphyrin antioxidant (AEOL 10150) in mitigating radiation-induced lung damage. Health Phys. 106:73–83.
- Georges GE, Kuver RP, Jordan R, Aragon A, Yang Y, Lesnikova M, Lesnikov V, Sale GE, McDonald GB. 2012. Post-exposure oral 17,21-beclomethasone dipropionate (BDP) improves survival in a canine gastrointestinal acute radiation syndrome (GI-ARS) model. Poster presented at: 58th Annual Meeting of the Radiation Research Society; San Juan (PR).
- Gerber SA, Cummings RJ, Judge JL, Barlow ML, Nanduri J, Johnson DE, Palis J, Pentland AP, Lord EM, Ryan JL. 2015. Interleukin-12 preserves the cutaneous physical and immunological barrier after radiation exposure. Radiat Res. 183:72–81.
- Ghosh SP, Kulkarni S, Perkins MW, Hieber K, Pessu RL, Gambles K, Maniar M, Kao TC, Seed TM, Kumar KS. 2012. Amelioration of radiation-induced hematopoietic and gastrointestinal damage by Ex-RAD(R) in mice. J Radiat Res. 53:526–536.
- Ghosh SP, Pathak R, Kumar P, Biswas S, Bhattacharyya S, Kumar VP, Hauer-Jensen M, Biswas R. 2016. Gamma-tocotrienol modulates radiation-induced MicroRNA expression in mouse spleen. Radiat Res. 185:485–495.
- Ghosh SP, Perkins MW, Hieber K, Kulkarni S, Kao TC, Reddy EP, Reddy MV, Maniar M, Seed T, Kumar KS. 2009. Radiation protection by a new chemical entity, ex-Rad: efficacy and mechanisms. Radiat Res. 171:173–179.
- Gianoncelli A, Bonini SA, Bertuzzi M, Guarienti M, Vezzoli S, Kumar R, Delbarba A, Mastinu A, Sigala S, Spano P, et al. 2015. An integrated approach for a structural and functional evaluation of biosimilars: implications for erythropoietin. BioDrugs. 29:285–300.
- Gluzman-Poltorak Z, Mendonca SR, Vainstein V, Kha H, Basile LA. 2014a. Randomized comparison of single dose of recombinant human IL-12 versus placebo for restoration of hematopoiesis and improved survival in rhesus monkeys exposed to lethal radiation. J Hematol Oncol. 7:31.
- Gluzman-Poltorak Z, Vainstein V, Basile LA. 2014b. Recombinant interleukin-12, but not granulocyte-colony stimulating factor, improves survival in lethally irradiated nonhuman primates in the absence of supportive care: evidence for the development of a frontline radiation medical countermeasure. Am J Hematol. 89:868–873.
- Gluzman-Poltorak Z, Vainstein V, Basile LA. 2015. Association of hematological nadirs and survival in a nonhuman primate model of hematopoietic syndrome of acute radiation syndrome. Radiat Res. 184:226–230.
- Grace MB, Blakely WF, Landauer MR. 2007. Genistein-induced alterations of radiation-responsive gene expression. Radiat Measur. 42:1152–1157.
- Grace MB, Singh VK, Rhee JG, Jackson WE 3rd, Kao TC, Whitnall MH. 2012. 5-AED enhances survival of irradiated mice in a G-CSF-dependent manner, stimulates innate immune cell function, reduces radiation-induced DNA damage and induces genes that modulate cell cycle progression and apoptosis. J Radiat Res. 53:840–853.
- Gridley DS, Makinde AY, Luo X, Rizvi A, Crapo JD, Dewhirst MW, Moeller BJ, Pearlstein RD, Slater JM. 2007. Radiation and a metalloporphyrin radioprotectant in a mouse prostate tumor model. Anticancer Res. 27:3101–3109.
- Gudkov SV, Popova NR, Bruskov VI. 2015. Radioprotectors: history, trends and prospects. Biofizika. 60:801–811.
- Gupta S. 2013. FDA advisory committee meeting: Study drug Leukine. Available at: http://www.fda.gov/downloads/advisorycommittees/committeesmeetingmaterials/drugs/medicalimagingdrugsadvisorycommittee/ucm350156.pdf [accessed 2 Oct 2016].
- Ha CT, Li XH, Fu D, Xiao M, Landauer MR. 2013. Genistein nanoparticles protect mouse hematopoietic system and prevent proinflammatory factors after gamma irradiation. Radiat Res. 180:316–325.
- Hammond WP, Boone TC, Donahue RE, Souza LM, Dale DC. 1990. A comparison of treatment of canine cyclic hematopoiesis with recombinant human granulocyte-macrophage colony-stimulating factor (GM-CSF), G-CSF interleukin-3, and canine G-CSF. Blood. 76:523–532.
- Hauer-Jensen M. 2014. Toward development of interleukin-11 as a medical countermeasure for use in radiological/nuclear emergencies. Dig Dis Sci. 59:1349–1351.
- Health and Human Services. 2016. HHS enhances nation’s health preparedness for radiological threats. Available at: https://www.hhs.gov/about/news/2016/10/06/hhs-enhances-nation-s-health-preparedness-radiological-threats.html [accessed 20 Oct 2016].
- Henry M, Jackson Foundation for the Advancement of Military Medicine Inc., Kumar KS, Srinivasan V, Seed TM. 2004. Radiation protection by gamma-tocotrienol. PCT/US2002/004522; WO/2004/086412.
- Huang R, Yu H, Kuang A. 2002. Radioprotective effects on head and neck tumors of amifostine – a broad-spectrum cytoprotection. Sheng Wu Yi Xue Gong Cheng Xue Za Zhi. 19:708–711.
- Humanetics Pharmaceuticals. 2016. BIO 300: development programs. Edina (MN): Humanetics Pharmaceuticals. Available at: http://humanetics.a03.neon.ittrium.com/development-programs [accessed 5 July 2016].
- Hussar DA. 2005. New drugs 05, part I. Nursing. 35:54–61.
- International Atomic Energy Agency (IAEA). 1988. The radiological accident in Goiânia. Available at: http://www-pub.iaea.org/MTCD/Publications/PDF/Pub815_web.pdf [accessed 2 Oct 2016].
- International Atomic Energy Agency (IAEA). 1990. The radiological accident in San Salvador. Available at: http://www-pub.iaea.org/books/IAEABooks/3718/The-Radiological-Accident-in-San-Salvador [accessed 20 Feb 2014].
- International Atomic Energy Agency (IAEA). 1993. The radiological accident in Soreq. Available at: http://www-pub.iaea.org/books/IAEABooks/3798/The-Radiological-Accident-in-Soreq [accessed 20 Feb 2014].
- International Atomic Energy Agency (IAEA). 1996. The radiological accident at the irradiation facility in Nesvizh. Available at: http://www-pub.iaea.org/books/IAEABooks/4712/The-Radiological-Accident-at-the-Irradiation-Facility-in-Nesvizh [accessed 10 Feb 2014].
- Jackson IL, Vujaskovic Z, Down JD. 2010. Revisiting strain-related differences in radiation sensitivity of the mouse lung: recognizing and avoiding the confounding effects of pleural effusions. Radiat Res. 173:10–20.
- Jackson IL, Vujaskovic Z, Down JD. 2011. A further comparison of pathologies after thoracic irradiation among different mouse strains: finding the best preclinical model for evaluating therapies directed against radiation-induced lung damage. Radiat Res. 175:510–518.
- Jackson IL, Xu P, Hadley C, Katz BP, McGurk R, Down JD, Vujaskovic Z. 2012. A preclinical rodent model of radiation-induced lung injury for medical countermeasure screening in accordance with the FDA animal rule. Health Phys. 103:463–473.
- Johnke RM, Sattler JA, Allison RR. 2014. Radioprotective agents for radiation therapy: future trends. Future Oncol. 10:2345–2357.
- Kang AD, Cosenza SC, Bonagura M, Manair M, Reddy MV, Reddy EP. 2013. ON01210.Na (Ex-RAD®) mitigates radiation damage through activation of the AKT pathway. PLoS One. 8:e58355.
- Karacetin D, Yucel B, Leblebicioglu B, Aksakal O, Maral O, Incekara O. 2004. A randomized trial of amifostine as radioprotector in the radiotherapy of head and neck cancer. BUON. 9:23–26.
- Kazzi ZN, Heyl A, Ruprecht J. 2012. Calcium and zinc DTPA administration for internal contamination with plutonium-238 and americium-241. Curr Pharm Biotechnol. 13:1957–1963.
- Kehlet RA, Robinson SI, Young RW, Feister A. 1996. Selection of a drug for prevention of radiation-induced nausea and vomiting. In: Reeves GI, Jarrett DG, Seed TM, King GL, Blakely WF, editors. Triage of irradiated personnel. Armed forces radiobiology research institute workshop. Bethesda, MD: Armed Forces Radiobiology Research Institute.
- Kim H, Peterson TG, Barnes S. 1998. Mechanisms of action of the soy isoflavone genistein: emerging role for its effects via transforming growth factor b signaling pathways. Am J Clin Nutr. 68S:1418S–1425S.
- Koukourakis MI, Panteliadou M, Abatzoglou IM, Sismanidou K, Sivridis E, Giatromanolaki A. 2013. Postmastectomy hypofractionated and accelerated radiation therapy with (and without) subcutaneous amifostine cytoprotection. Int J Radiat Oncol Biol Phys. 85:e7–13.
- Krivokrysenko VI, Shakhov AN, Singh VK, Bone F, Kononov Y, Shyshynova I, Cheney A, Maitra RK, Purmal A, Whitnall MH, et al. 2012. Identification of granulocyte colony-stimulating factor and interleukin-6 as candidate biomarkers of CBLB502 efficacy as a medical radiation countermeasure. J Pharmacol Exp Ther. 343:497–508.
- Krivokrysenko VI, Toshkov IA, Gleiberman AS, Krasnov P, Shyshynova I, Bespalov I, Maitra RK, Narizhneva NV, Singh VK, Whitnall MH, et al. 2015. The Toll-like receptor 5 agonist Entolimod mitigates lethal acute radiation syndrome in non-human primates. PLoS One. 10:e0135388.
- Kruk I, Aboul-Enein HY, Michalska T, Lichszteld K, Kladna A. 2005. Scavenging of reactive oxygen species by the plant phenols genistein and oleuropein. Luminescence. 20:81–89.
- Kulkarni S, Singh PK, Ghosh SP, Posarac A, Singh VK. 2013. Granulocyte colony-stimulating factor antibody abrogates radioprotective efficacy of gamma-tocotrienol, a promising radiation countermeasure. Cytokine. 62:278–285.
- Kumar KS, Papas A, Seed TM, Srinivasan V. 2006. Radiation protection by gamma-tocotrienol. USA Patent application PCT/US2004/00452.
- Landauer MR, Srinivasan V, Seed TM. 2003. Genistein treatment protects mice from ionizing radiation injury. J Appl Toxicol. 23:379–385.
- Lauritano D, Petruzzi M, Di Stasio D, Lucchese A. 2014. Clinical effectiveness of palifermin in prevention and treatment of oral mucositis in children with acute lymphoblastic leukaemia: a case-control study. Int J Oral Sci. 6:27–30.
- Liu Q, Jiang B, Jiang LP, Wu Y, Wang XG, Zhao FL, Fu BH, Istvan T, Jiang E. 2008. Clinical report of three cases of acute radiation sickness from a (60)Co radiation accident in Henan Province in China. JRR. 49:63–69.
- Lord BI, Molineux G, Pojda Z, Souza LM, Mermod JJ, Dexter TM. 1991. Myeloid cell kinetics in mice treated with recombinant interleukin-3, granulocyte colony-stimulating factor (CSF), or granulocyte-macrophage CSF in vivo. Blood. 77:2154–2159.
- Loria RM, Conrad DH, Huff T, Carter H, Ben-Nathan D. 2000. Androstenetriol and androstenediol. Protection against lethal radiation and restoration of immunity after radiation injury. Ann NY Acad Sci. 917:860–867.
- MacVittie TJ, Monroy RL, Farese AM, Seiler FR, Williams D. 1991. Cytokine therapy in canine and primate models of radiation-induced marrow aplasia. Behring Inst Mitt. 90:1–13.
- MacVittie TJ, Farese AM, Smith WG, Baum CM, Burton E, McKearn JP. 2000. Myelopoietin, an engineered chimeric IL-3 and G-CSF receptor agonist, stimulates multilineage hematopoietic recovery in a nonhuman primate model of radiation-induced myelosuppression. Blood. 95:837–845.
- Mahmood J, Jelveh S, Calveley V, Zaidi A, Doctrow SR, Hill RP. 2011. Mitigation of radiation-induced lung injury by genistein and EUK-207. Int J Radiat Biol. 87:889–901.
- Monroy RL, Davis TA, MacVittie TJ. 1990. Granulocyte-macrophage colony-stimulating factor: more than a hemopoietin. Clin Immunol Immunopathol. 54:333–346.
- Monroy RL, Skelly RR, Taylor P, Dubois A, Donahue RE, MacVittie TJ. 1988. Recovery from severe hematopoietic suppression using recombinant human granulocyte-macrophage colony-stimulating factor. Exp Hematol. 16:344–348.
- Murigi FN, Mohindra P, Hung C, Salimi S, Goetz W, Pavlovic R, Jackson IL, Vujaskovic Z. 2015. Dose optimization study of AEOL 10150 as a mitigator of radiation-induced lung injury in CBA/J mice. Radiat Res. 184:422–432.
- Nabholtz JM, Cantin J, Chang J, Guevin R, Patel R, Tkaczuk K, Vodvarka P, Lindsay MA, Reese D, Riva A, et al. 2002. Phase III trial comparing granulocyte colony-stimulating factor to leridistim in the prevention of neutropenic complications in breast cancer patients treated with docetaxel/doxorubicin/cyclophosphamide: results of the BCIRG 004 trial. Clin Breast Cancer. 3:268–275.
- Nagayama H, Misawa K, Tanaka H, Ooi J, Iseki T, Tojo A, Tani K, Yamada Y, Kodo H, Takahashi TA, et al. 2002. Transient hematopoietic stem cell rescue using umbilical cord blood for a lethally irradiated nuclear accident victim. Bone Marrow Transplant. 29:197–204.
- Nash RA, Schuening FG, Seidel K, Appelbaum FR, Boone T, Deeg HJ, Graham TC, Hackman R, Sullivan-Pepe M, Storb R. 1994. Effect of recombinant canine granulocyte-macrophage colony-stimulating factor on hematopoietic recovery after otherwise lethal total body irradiation. Blood. 83:1963–1970.
- National Institute of Allergic and Infectious Diseases. 2015. Pegfilgrastim approved for treating acute radiation syndrome. Available at: https://www.niaid.nih.gov/topics/radnuc/Pages/pegfilgrastim.aspx [accessed 18 Aug 2016].
- Neelis KJ, Hartong SC, Egeland T, Thomas GR, Eaton DL, Wagemaker G. 1997. The efficacy of single-dose administration of thrombopoietin with coadministration of either granulocyte/macrophage or granulocyte colony-stimulating factor in myelosuppressed rhesus monkeys. Blood. 90:2565–2573.
- Neumedicines. 2014. HemaMax™ (rHuIL-12) for acute radiation syndrome. Pasadena, CA: Neumedicines. Available at: http://www.neumedicines.com/ [accessed 27 Oct 2014].
- Nothdurft W, Selig C, Fliedner TM, Hintz-Obertreis P, Kreja L, Krumwieh D, Kurrle R, Seiler FR, Weinsheimer W. 1992. Haematological effects of rhGM-CSF in dogs exposed to total-body irradiation with a dose of 2.4 Gy. Int J Radiat Biol. 61:519–531.
- Oki T, Sowa Y, Hirose T, Takagaki N, Horinaka M, Nakanishi R, Yasuda C, Yoshida T, Kanazawa M, Satomi Y, et al. 2004. Genistein induces Gadd45 gene and G2/M cell cycle arrest in the DU145 human prostate cancer cell line. FEBS Lett. 577:55–59.
- Orrell RW. 2006. AEOL-10150 (Aeolus). Curr Opin Investig Drugs. 7:70–80.
- Para AE, Bezjak A, Yeung IW, Van Dyk J, Hill RP. 2009. Effects of genistein following fractionated lung irradiation in mice. Radiother Oncol. 92:500–510.
- Patchen ML, Fischer R, MacVittie TJ, Seiler FR, Williams DE. 1993. Mast cell growth factor (C-kit ligand) in combination with granulocyte-macrophage colony-stimulating factor and interleukin-3: in vivo hemopoietic effects in irradiated mice compared to in vitro effects. Biotherapy. 7:13–26.
- Pathak R, Bachri A, Ghosh SP, Koturbash I, Boerma M, Binz RK, Sawyer JR, Hauer-Jensen M. 2016. The vitamin E analog gamma-tocotrienol (GT3) suppresses radiation-induced cytogenetic damage. Pharm Res. 33:2117–2125.
- Pearlstein RD, Higuchi Y, Moldovan M, Johnson K, Fukuda S, Gridley DS, Crapo JD, Warner DS, Slater JM. 2010. Metalloporphyrin antioxidants ameliorate normal tissue radiation damage in rat brain. Int J Radiat Biol. 86:145–163.
- Peebles DD, Soref CM, Copp RR, Thunberg AL, Fahl WE. 2012. ROS-scavenger and radioprotective efficacy of the new PrC-210 aminothiol. Radiat Res. 178:57–68.
- Potten CS. 1995. Interleukin-11 protects the clonogenic stem cells in murine small-intestinal crypts from impairment of their reproductive capacity by radiation. Int J Cancer. 62:356–361.
- Potten CS. 1996. Protection of the small intestinal clonogenic stem cells from radiation-induced damage by pretreatment with interleukin 11 also increases murine survival time. Stem Cells. 14:452–459.
- Praetorius NP, Mandal TK. 2008. Alternate delivery route for amifostine as a radio-/chemo-protecting agent. J Pharm Pharmacol. 60:809–815.
- Reeves G. 2014. Overview of use of G-CSF and GM-CSF in the treatment of acute radiation injury. Health Phys. 106:699–703.
- Sanofi-aventis U.S. LLC. 2013. Leukine. Available at: http://products.sanofi.us/Leukine/Leukine.html [accessed 20 Oct 2016].
- Santini V. 2001. Amifostine: chemotherapeutic and radiotherapeutic protective effects. Expert Opin Pharmacother. 2:479–489.
- Schuchter LM. 1996. Exploration of platinum-based dose-intensive chemotherapy strategies with amifostine (Ethyol). Eur J Cancer. 32A(Suppl.4):S40–S42.
- Seed TM. 2005. Radiation protectants: current status and future prospects. Health Phys. 89:531–545.
- Seed TM, Inal CE, Singh VK. 2014. Radioprotection of hematopoietic progenitors by low dose amifostine prophylaxis. Int J Radiat Biol. 90:594–604.
- Selig C, Nothdurft W, Kreja L, Fliedner TM. 1991. Influence of combined treatment with interleukin 1 and erythropoietin or GM-CSF and erythropoietin on the regeneration of hemopoiesis in the dog after total body irradiation – a preliminary report. Behring Inst Mitt. 90:86–92.
- Singh VK, Christensen J, Fatanmi OO, Gille D, Ducey EJ, Wise SY, Karsunky H, Sedello AK. 2012a. Myeloid progenitors: A radiation countermeasure that is effective when initiated days after irradiation. Radiat Res. 177:781–791.
- Singh VK, Ducey EJ, Brown DS, Whitnall MH. 2012b. A review of radiation countermeasure work ongoing at the Armed Forces Radiobiology Research Institute. Int J Radiat Biol. 88:296–310.
- Singh VK, Fatanmi OO, Wise SY, Newman VL, Romaine PL, Seed TM. 2016a. The potentiation of the radioprotective efficacy of two medical countermeasures, gamma-tocotrienol and amifostine, by a combination prophylactic modality. Radiat Prot Dosimetry. 172:302–310.
- Singh VK, Hauer-Jensen M. 2016. Gamma-tocotrienol as a promising countermeasure for acute radiation syndrome: Current status. IJMS. 17:e663.
- Singh VK, Kulkarni S, Fatanmi OO, Wise SY, Newman VL, Romaine PL, Hendrickson H, Gulani J, Ghosh SP, Kumar KS, et al. 2016b. Radioprotective efficacy of gamma-tocotrienol in nonhuman primates. Radiat Res. 185:285–298.
- Singh VK, Newman VL, Seed TM. 2015a. Colony-stimulating factors for the treatment of the hematopoietic component of the acute radiation syndrome (H-ARS): a review. Cytokine. 71:22–37.
- Singh VK, Olabisi AO. 2017. Nonhuman primates as models for the discovery and development of radiation countermeasures. Expert Opin Drug Discov. 12:695–709.
- Singh VK, Romaine PL, Newman VL, Seed TM. 2016c. Medical countermeasures for unwanted CBRN exposures: part II radiological and nuclear threats with review of recent countermeasure patents. Expert Opin Ther Pat. 26:1399–1408.
- Singh VK, Romaine PL, Seed TM. 2015b. Medical countermeasures for radiation exposure and related injuries: Characterization of medicines, FDA-approval status and inclusion into the Strategic National Stockpile. Health Phys. 108:607–630.
- Singh VK, Shafran RL, Inal CE, Jackson WE 3rd, Whitnall MH. 2005. Effects of whole-body gamma irradiation and 5-androstenediol administration on serum G-CSF. Immunopharmacol Immunotoxicol. 27:521–534.
- Soligenix Inc. 2016. OrbeShield™ for gastrointestinal acute radiation syndrome (GI ARS). Princeton, NJ: Soligenix, Inc. Available at: http://www.soligenix.com/pipeline/vaccinesbiodefense/orbeshield-for-gastrointestinal-acute-radiation-syndrome-gi-ars/ [accessed 10 July 2016].
- Soref CM, Hacker TA, Fahl WE. 2012. A new orally active, aminothiol radioprotector-free of nausea and hypotension side effects at its highest radioprotective doses. Int J Radiat Oncol Biol Phys. 82:e701–e707.
- Stickney DR, Dowding C, Authier S, Garsd A, Onizuka-Handa N, Reading C, Frincke JM. 2007. 5-androstenediol improves survival in clinically unsupported rhesus monkeys with radiation-induced myelosuppression. Int Immunopharmacol. 7:500–505.
- Stickney DR, Dowding C, Garsd A, Ahlem C, Whitnall M, McKeon M, Reading C, Frincke J. 2006. 5-androstenediol stimulates multilineage hematopoiesis in rhesus monkeys with radiation-induced myelosuppression. Int Immunopharmacol. 6:1706–1713.
- Stickney DR, Groothuis JR, Ahlem C, Kennedy M, Miller BS, Onizuka-Handa N, Schlangen KM, Destiche D, Reading C, Garsd A, et al. 2010. Preliminary clinical findings on NEUMUNE as a potential treatment for acute radiation syndrome. J Radiol Prot. 30:687–698.
- Suman S, Datta K, Chakraborty K, Kulkarni SS, Doiron K, Fornace AJ Jr, Sree Kumar K, Hauer-Jensen M, Ghosh SP. 2013. Gamma tocotrienol, a potent radioprotector, preferentially upregulates expression of anti-apoptotic genes to promote intestinal cell survival. Food Chem Toxicol. 60:488–496.
- Suman S, Datta K, Doiron K, Ren C, Kumar R, Taft DR, Fornace AJ Jr, Maniar M. 2012a. Radioprotective effects of ON 01210.Na upon oral administration. J Radiat Res. 53:368–376.
- Suman S, Maniar M, Fornace AJ Jr, Datta K. 2012b. Administration of ON 01210.Na after exposure to ionizing radiation protects bone marrow cells by attenuating DNA damage response. Radiat Oncol. 7:6.
- Tacyildiz N, Ozyoruk D, Yavuz G, Unal E, Dincaslan H, Dogu F, Sahin K, Kucuk O. 2010. Soy isoflavones ameliorate the adverse effects of chemotherapy in children. Nutr Cancer. 62:1001–1005.
- Tamulevicius P, Wang M, Iliakis G. 2007. Homology-directed repair is required for the development of radioresistance during S phase: interplay between double-strand break repair and checkpoint response. Radiat Res. 167:1–11.
- Tanikawa S, Nakao I, Tsuneoka K, Nara N. 1989. Effects of recombinant granulocyte colony-stimulating factor (rG-CSF) and recombinant granulocyte-macrophage colony-stimulating factor (rGM-CSF) on acute radiation hematopoietic injury in mice. Exp Hematol. 17:883–888.
- The Radiation Emergency Assistance Center/Training Site. 2013. The medical aspects of radiation incidents. Available at: https://orise.orau.gov/files/reacts/medical-aspects-of-radiation-incidents.pdf [accessed 28 Oct 2016].
- U.S. Department of Health & Human Services. 2013. HHS boosts stockpile of products to treat acute radiation syndrome. Available at: http://www.hhs.gov/news/press/2013pres/09/20130926a.html [accessed 12 Feb 2014].
- U.S. Food and Drug Administration. 2015. FDA approves Neupogen for treatment of patients with radiation-induced myelosuppression following a radiological/nuclear incident. Silver Spring (MD): U.S. Food and Drug Administration. Available at: http://www.fda.gov/EmergencyPreparedness/Counterterrorism/MedicalCountermeasures/AboutMCMi/ucm443245.htm [accessed 6 July 2016].
- Vadhan-Raj S, Goldberg JD, Perales MA, Berger DP, van den Brink MR. 2013. Clinical applications of palifermin: amelioration of oral mucositis and other potential indications. J Cell Mol Med. 17:1371–1384.
- Valachovicova T, Slivova V, Sliva D. 2004. Cellular and physiological effects of soy flavonoids. Mini Rev Med Chem. 4:881–887.
- van der Vijgh WJ, Peters GJ. 1994. Protection of normal tissues from the cytotoxic effects of chemotherapy and radiation by amifostine (Ethyol): preclinical aspects. Semin Oncol. 21:2–7.
- Vasin MV, Antipov VV, Chernov GA, L’Vova TS, Koroleva LV, Semenova LA, Liutykh VP, Komarova SN, Gaidamakin NA, Vilkina GA. 1996. Studies of the radiation-protective effects of indralin on the hematopoietic system of different species of animals. Radiats Biol Radioecol. 36:168–189.
- Vasin MV, Semenov LF, Suvorov NN, Antipov VV, Ushakov IB, Ilyin LA, Lapin BA. 2014. Protective effect and the therapeutic index of indralin in juvenile rhesus monkeys. J Radiat Res. 55:1048–1055.
- Waddick KG, Song CW, Souza L, Uckun FM. 1991. Comparative analysis of the in vivo radioprotective effects of recombinant granulocyte colony-stimulating factor (G-CSF), recombinant granulocyte-macrophage CSF, and their combination. Blood. 77:2364–2371.
- Wasserman TH, Brizel DM. 2001. The role of amifostine as a radioprotector. Oncology (Williston Park, NY). 15:1349–1354.
- Whitcomb RC Jr, Ansari AJ, Buzzell JJ, McCurley MC, Miller CW, Smith JM, Evans DL. 2015. A public health perspective on the U.S. response to the Fukushima radiological emergency. Health Phys. 108:357–363.
- Whitnall MH, Elliott TB, Harding RA, Inal CE, Landauer MR, Wilhelmsen CL, McKinney L, Miner VL, Jackson WE 3rd, Loria RM, et al. 2000. Androstenediol stimulates myelopoiesis and enhances resistance to infection in gamma-irradiated mice. Int J Immunopharmacol. 22:1–14.
- Whitnall MH, Villa V, Seed TM, Benjack J, Miner V, Lewbart ML, Dowding CA, Jackson WE 3rd. 2005. Molecular specificity of 5-androstenediol as a systemic radioprotectant in mice. Immunopharmacol Immunotoxicol. 27:1–18.
- Whitnall MH, Wilhelmsen CL, McKinney L, Miner V, Seed TM, Jackson WE 3rd. 2002. Radioprotective efficacy and acute toxicity of 5-androstenediol after subcutaneous or oral administration in mice. Immunopharmacol Immunotoxicol. 24:595–626.
- Wu HJ, Chan WH. 2007. Genistein protects methylglyoxal-induced oxidative DNA damage and cell injury in human mononuclear cells. Toxicol in Vitro. 21:335–342.
- Yamamoto LG. 2013. Risks and management of radiation exposure. Pediatr Emerg Care. 29:1016–1026.
- Zhang Y, Zhang X, Rabbani ZN, Jackson IL, Vujaskovic Z. 2012. Oxidative stress mediates radiation lung injury by inducing apoptosis. Int J Radiat Oncol Biol Phys. 83:740–748.