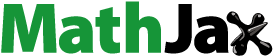
Abstract
Purpose
Total body irradiation of the Gottingen minipig results in a characteristic hematopoietic response, including anemia, neutropenia, lymphocytopenia, and thrombocytopenia. Currently, there are no well-characterized large or small animal models for radiation-induced thrombocytopenia. The study described here using the Gottingen minipig was focused on understanding which aspects of the coagulation cascade leads to radiation-induced coagulopathy. In this study, multiple clinical pathology parameters were determined prior to and for 45-days following total body irradiation using a 6 MV photon linear accelerator.
Materials and methods
Following irradiation, frequent analyses of conventional hematology and coagulation parameters provided time-course information on the onset and recovery of thrombocytopenia. In addition, thromboelastography (TEG) was utilized to monitor coagulation dysfunction, namely clotting time, clot formation time, clot strength, and fibrinolysis. Coagulation factor activity levels were measured for factors II, V, VII, VIII, IX, X, XI, XII, XIII, Protein C, fibrin monomers, antiplasmin and D-dimer using a Siemen’s coagulation analyzer to provide time course information of changes in activity post irradiation exposure.
Results
These analyses revealed that in total body irradiated minipigs, TEG tracings demonstrate long R (time to initial clot formation) and K (time to achieve a certain clot strength) times, and low alpha-angle (rate of clot formation) and MA (overall stability of the clot) during onset of thrombocytopenia (typically post irradiation day 10–15). Low alpha-angle and MA directly correlated with decreased platelet counts. A long R time is suggestive of a deficiency in clotting factors and was compared to measured activity levels of individual coagulation factors. The data indicates that coagulation factors are significantly changed early after irradiation exposure prior to thrombocytopenia and factors VIII, XI, XII and XIII are markedly altered during the critical point of thrombocytopenia.
Conclusion
These data support the continued use of multiple approaches to evaluate the coagulation cascade in order to provide the most meaningful interpretation of the hematopoietic changes that occur post irradiation.
Introduction
Concerns over nuclear and radiological threats have prompted the need to improve methods to protect the general population from the health hazards associated with exposure to ionizing radiation. The development of effective medical countermeasures requires efficacy studies be conducted in an animal model(s) predictive of the human response (‘the Animal Rule’ 21 CFR 314.600 for drugs). However, the reliance on non-human efficacy data places an enormous importance on appropriately developed and well-characterized animal models. Currently, there is no well-characterized large or small animal model for radiation-induced coagulopathy. Preliminary coagulation studies in rodents have been reported, but not yet replicated (i.e. Chernyshenko et al. Citation2019). The need for both small and large animal models is acute. NASA scientists have recognized that disseminated intravascular coagulation (DIC) type reactions are expected in extended space flight operations (Blue et al. Citation2017). Radiation-induced coagulopathy has been reported in cancer patients and in Hiroshima and Nagasaki atomic bomb victims (Ohkita Citation1975; Stupp et al. Citation2005; Robins et al. Citation2006; Lai et al. Citation2008; Gutin et al. Citation2009).
The Biomedical Advanced Research and Development Authority (BARDA) has reported on the development of a harmonized total body irradiation model in the male Gottingen minipig (Esker et al. Citation2015). The work described here focused on evaluating individual factors involved in the blood coagulation pathway to elucidate the major components that impact the development and progression of radiation-induced coagulopathy (hyper- and hypo-coagulation) in the minipig. This information is considered critical to understand this radiation-injury pathway and to facilitate the development of medical countermeasures that mitigate the hematopoietic and vascular injury resulting hemorrhage and multi-organ dysfunction. The need for a translatable animal model is especially relevant as it is understood that human exposure to clinical level radiation doses can lead to an uncontrolled inflammatory response resulting also in significant vascular injury (Weintraub et al., Citation2010). Putative mechanisms have been reviewed by Venkatesulu et al. (Citation2018) and conclude that endothelial injury is an increasingly recognized consequence of cancer treatment as well.
Materials and methods
Animals
Twenty (20) male Gottingen minipigs, approximately 5–7 months of age and 9–15 kg (at the time of irradiation), were obtained from Marshall Bioresources (North Rose NY). Four additional age-matched male animals were obtained for use as sham control (non-irradiated) comparisons. Animals were pair housed in pens that comply with the Animal Welfare Act and recommendations set forth in the Guide for the Care and Use of Laboratory Animals (National Research Council Citation2011). Animals were individually housed if necessary due to declining condition or aggression. Pens were attached in units, allowing pigs to see and touch neighboring animals. Individual water lines with lixit valves were provided to each pen, allowing water consumption ad libitum. A variety of veterinary approved toys were provided for enrichment. Animals were offered PMI LabDiet® K599 Certified Lab Mini Pig Diet in a ration based on body weight twice daily. Animals were offered fresh fruits, vegetables, and other dietary supplements throughout the course of the study. The light cycle was 12 hour light and 12 hour dark and temperature and humidity were as recommended for minipigs: 16–27 °C and 30–70%, respectively. Animals acclimated to the facility for at least 14 days prior to irradiation.
Supportive care consisted of fluids, antibiotics, and analgesics administered to all animals regardless of indication beginning post irradiation Day 3 and continuing through Day 30. Fluid support involved mixing approximately 240 mL of tap water or fruit juice (excluding citrus juice) with each regular food ration and allowing the food to soften for approximately 10 minutes. Antibiotic support consisted of amoxicillin suspension administered oral (10 mg kg−1 twice a day (BID)) and soluble gentamicin administered oral (2 mg kg−1 once a day (SID)). Gentamicin was mixed with fruit juice (excluding citrus juice). All animals received oral analgesic (tramadol 2–4 mg kg−1 BID). Animals that refused oral administration of tramadol received buprenorphine (0.01 mg kg−1 by subcutaneous injection, BID). Animals that demonstrated pain or distress not relieved by tramadol (BID) or buprenorphine at a dose of 0.01 mg kg−1 were administered tramadol three times a day (TID) or buprenorphine at a dose of 0.02 mg kg−1.
Animal protocols were approved by the Institutional Animal Care and Use Committee at Altasciences. The facility is accredited by the Association for Assessment and Accreditation of Laboratory Animal Care International, is registered with the USDA, and holds an Office of Laboratory Animal Welfare assurance.
Irradiation
Body measurements (length and width measured at the widest location along the length of the animal) were taken with the animal in a prone position with the legs tucked into the body. Measurements were provided to the Medical Physicist prior to the irradiation date. Animals were not fed in the morning of irradiation. Prior to transport to the Linear Accelerator (Linac; Varian CLINAC 21EX, Palo Alto CA), the animals were administered atropine (0.05–0.5 mg kg−1 by intramuscular injection), and then anesthetized with ketamine/xylazine. Sedated animals were positioned on a Styrofoam block on a turntable placed on the Linac treatment table in a prone position with the legs tucked into the body and the animal perpendicular to the Gantry. The Gantry was at a 90-degree angle and the couch moved in/out to accommodate the entire animal within the beam. An acrylic beam spoiler plate was placed between the animal and the beam.
Each animal was exposed to 2.1 Gy given by irradiation with the Linac using a bilateral technique by the 6 MV photon beam, with approximately 50% dose contribution from both the right and left lateral beams. All irradiations occurred between 9 am and 1 pm. Real-time exposure measurements were confirmed using a Sun Nuclear Corporation (Melbourne FL) In-Vivo Dosimetry system. The diode was placed on the acrylic plate between the animal and the photon beam in close proximity to the animal, ensuring that it did not shield any portion of the animal. Diode readings were corrected to midline dose based on dosimetry calculations. The nominal dose rate was 75 ± 5 cGy min−1.
The radiation exposure level was selected based on the institutional lethality profile estimated to correlate to the LD50 (). A Medical Physicist performed a phantom exposure to confirm accuracy of the exposure level. Monitor Units (MUs) were calculated based on animal dimensions provided prior to irradiation using the formula:
where TMR is the tissue maximum ratio, based on effective field size and calculation depth; Output at 215 cm is the radiation output in cGy measured using a Virtual Water Phantom at 215 cm with the appropriate animal positioning apparatus, and the Normalization Factor is the output measured on the day of irradiation.
Observations and parameter measurements
Cageside clinical observations were performed twice daily throughout the study. The first observation occurred in the morning prior to room cleaning and the second observation occurred approximately 6 hours after the morning observation. The clinical observations focused specifically on evaluating level of activity (normal, decreased, non-responsive), animal posture (normal, hunched, recumbent or refused to stand), stool consistency (normal, soft, loose and/or liquid, hard, none present), blood in stool (blood observed, none present), emesis (none present, present, profuse), blood in cage (none present, spotting, pooling), respiratory activity (normal, increased, labored), alopecia (normal, slight, moderate, extreme, complete), and convulsions (observed, none present). A qualitative evaluation of food consumption was made once daily throughout the study. Food consumption was made based on the approximate amount of food remaining within 30 minutes following feeding and was reported as none (no consumption), low (approximately less than 50% consumption), or normal (approximately greater than 50% consumption). Body weights were measured the day prior to irradiation, daily post irradiation Days 1–30, and every third day thereafter. Rectal temperature was measured daily to post-irradiation Day 30, and weekly thereafter.
Blood was collected the day prior to irradiation, 2 and 6 hours post irradiation, and post irradiation Days 1, 2, 4, 7, 10, 15, 20, 25, 35, and 45 by venipuncture from a peripheral vein from restrained, conscious animals for hematology, serum chemistry, coagulation, thromboelastography (TEG), and coagulation factor analyses. Hematology samples were analyzed using an Advia 120 analyzer (Siemens Medical Solutions, Malvern PA), and blood smears were examined microscopically. Coagulation samples were analyzed using a STACompact (Diagnostica Stago, Parsippany NJ) coagulation analyzer to determine prothrombin time (PT), activated partial thromboplastin time (aPTT) and fibrinogen levels in citrated plasma. Thromboelastography was performed using kaolin-activated citrated whole blood samples and a TEG 5000® thromboelastography hemostasis analyzer (Haemonetics, Braintree MA). The following parameters were measured: reaction time (R), clot formation time (K), angle (α), maximum amplitude (MA), and clot lysis 30 minutes after MA (LY30). Samples for serum chemistry were analyzed using an AU680 analyzer. Coagulation factors (Factor II, V, VII, VIII, IX, X, XI, XII, XIII, Protein C, D-dimer, antiplasmin, and soluble fibrin (fibrin monomer)) were analyzed as activated factors using a Siemen’s BCS XP coagulation analyzer (Siemens Medical Solutions, Malvern PA).
Euthanasia criteria
The criteria for euthanasia were explicitly defined by Standard Operating Procedure (SOP) to avoid bias. Absolute criteria included any single one of the following observations: Indication of unrelieved pain or distress following administration of two consecutive increased series of analgesic administration (tramadol three times a day or buprenorphine at 0.02 mg kg−1), inactivity (i.e. non-responsive to touch or inability to remain standing when placed in an upright position), respiratory distress (e.g. open mouth breathing, cyanotic appearance, labored breathing), uncontrolled hemorrhage from any orifice, severe dehydration as evidenced by a skin tent time > 3 seconds, sunken eyes, rapid and weak pulse, and/or cold extremities, neurologic distress (e.g. seizure activity that is non-responsive to medication, paralysis). Non-absolute euthanasia criteria included any combination of two or more of the following observations: tachypnea (i.e. ≥60 breaths per minute), loss of body weight ≥25% of Day −1 baseline value for two consecutive days, observations of a severe injury or condition (e.g. progressive tissue necrosis, non-healing wound), hypothermia (rectal temperature ≤35.0 °C), hyperthermia (rectal temperature ≥41.0 °C), complete anorexia for 48 hours. Moribund animals and animals that survived to post irradiation Day 45 were euthanized by intravenous injection of a commercial euthanasia solution.
Necropsy and histopathology
A macroscopic examination was performed at necropsy. Tissues for histopathology examination included bone marrow smear, bone, brain, heart, intestine (cecum, colon, duodenum, ileum, jejunum, rectum), kidneys, liver, lungs, pancreas, spleen and thymus. Tissues and any gross lesions were fixed in 10% Neutral Buffered Formalin, processed to paraffin blocks, sectioned and stained with Hematoxylin and Eosin for microscopic examination by a veterinary pathologist.
Statistical analysis
Summary statistics included average and standard deviations. Survival rate was calculated over the course of the study using the Kaplan-Meier estimator (Fleming and Harrington Citation1984) and by a dose normal probit fit. Comparison of continuous marker variables over time (pre- versus post-irradiation changes) was carried out using one-sample t-test, the one-sample permutation test, the Wilcoxon signed rank test, or the linear mixed model, as appropriate. Body weights and coagulation factor data were analyzed as a percent change from baseline (pre-irradiation) values on a per animal basis.
Results
All animals were exposed to 6 MV photon irradiation at a nominal dose rate of 75 ± 5 cGy min−1. Machine outputs were cross-checked with a validated (21 CFR Part 58; 21 CFR Part 11) Sun Nuclear Daily Quality Assurance system and real-time exposure measurements were confirmed using a validated Sun Nuclear In-vivo Diode Dosimetry system. A summary of the in-vivo diode measurements showed that the percent of midline tissue dose versus prescription ranged from 2.7% to 5.2% (average 3.8%).
In total, 6 of 20 animals died or were euthanized early due to moribund criteria (). The mean survival time of decedents was 21 days and mortality ranged from 18–25 days post irradiation (median 20 days). Cageside observations for decedents included petechia and/or ecchymosis (typically beginning on Day 15), decreased activity (typically occurring 1–2 days prior to day of death), and sporadic incidences of soft stool. In contrast, only two animals that survived to Day 45 were observed with petechia and/or ecchymosis (both starting on Day 15) and no surviving animals were reported with decreased activity. Soft stool was observed in a number of surviving animals (). Food consumption was observed as normal for all surviving animals. Several of the moribund animals had low or no food consumption on the day they met moribund criteria for euthanasia, however food consumption was observed as normal for most of the animals. Body temperatures fluctuated between 35 and 38 °C with intermittent higher values. A body temperature greater than 41 °C was recorded for one of the decedents during the last two days the animal was alive.
Table 1. Frequency (%) and day(s) of appearance of cage side observations typically associated with total body irradiation in the minipig.
Based on histopathology, the primary cause of death appeared to be hemorrhage in several organs, including brain, heart, intestines, kidneys, liver and lungs. The most striking difference between surviving animals and decedents was the overall decreased cellularity of the bone marrow, estimated to be 95% or greater in moribund animals. Mild focal fibrin in alveoli of the lung likely related to vascular leakage associated with hemorrhage was observed for two decedents, one of which was euthanized for respiratory distress and cyanotic appearance (the second animal was euthanized for recumbency).
Hematological parameters were determined for all animals pre- and post-irradiation. All animals displayed characteristic hematological responses, including neutropenia, lymphopenia, thrombocytopenia, and anemia (). Hematology results reported here are analogous to that reported by Moroni et al. (Citation2011). Blood smear evaluation revealed polychromasia and macrocytes in individual surviving animals, suggestive of a regenerative response; a regenerative response was not consistently observed in decedents.
Figure 2. (a) Average hematology parameters in male Gottingen minipigs following total body irradiation of 6 MV Linac-derived photons at a dose rate of approximately 0.75 Gy min–1. a) red blood cell (RBC) count. For decedents, each data point represents the average of n = 6 animals, with the exception of Day 20 (n = 4) and Day 25 (n = 1). A single animal succumbed at Day 18 and is provided independently (‘terminal sample’). The Sham Control group consisted of n = 4 animals. For ease in illustrating changes, the group standard deviations are not presented, however any statistical significance is noted by * (p < .0001). (b) platelet count. (c) neutrophil count (ANC). (d) absolute lymphocyte count (ALC).

Serum chemistry changes post irradiation were typically mild and transient in animals that survived to Day 45, including sporadic mild increases in creatine kinase (CK). For moribund animals, alternations noted included a slight decrease in albumin levels, a moderate increase in BUN levels, increased sodium and chloride levels, increased creatinine levels, and increased phosphorous levels (data not shown). Several of these changes (increased BUN, Na, Cl) may be indicative of dehydration. However, hematocrit, albumin and hemoglobin levels were not elevated in these animals, suggesting dehydration was not extensive, if present at all.
Conventional coagulation testing (fibrinogen, aPTT, PT) for surviving animals were generally unremarkable at all time points evaluated (data not shown). Similarly, for decedents, coagulation alterations were generally only detected in the blood sample collected at the time of moribund euthanasia. The alterations in these terminal samples consisted of slight to moderate elevation of fibrinogen concentrations and a mild PT prolongation.
Coagulation was further assessed using TEG to evaluate dysfunction in coagulation, namely clotting times (R and K), clot strength, and fibrinolysis. A typical TEG time course tracing for a surviving animal is provided in and contrasted to the time course tracing from a decedent () showing delayed clot formation time and absence of fibrinolysis post day 10. The average rate of clot formation (α-angle), time to initial fibrin formation (R), and stability of the clot (MA) differed between surviving animals and decedents, albeit in a non-statistically significant manner (). Evaluation of the TEG tracings indicate that all decedents, as well as several surviving animals, were thrombocytopenic (low α-angle and low MA), deficient in fibrinogen (long K time), and deficient in clotting factors (long R time). A graphical overlay of average MA and platelet levels illustrates the correlation between these two measurements (). It is worth noting that despite the long K time observed with TEG analyses, standard coagulation analyses did not indicate a fibrinogen deficiency regardless of survival outcome.
Figure 3. (a) TEG analysis results for an animal that survived to post irradiation Day 45. The individual tracings are coded by color. (b) TEG analysis results for an animal that was euthanized for moribund condition on post irradiation Day 25. The individual tracings are coded by color.

Figure 4. (a) Average TEG parameters in male Gottingen minipigs following total body irradiation. Shown are changes in a) rate of clot formation (α-angle). For decedents, each data point represents the average of n = 6 animals, with the exception of Day 20 (n = 4) and Day 25 (n = 1). A single animal succumbed at Day 18 and is provided independently (‘terminal sample’). The Sham Control group consisted of n = 4 animals. For ease in illustrating changes, the group standard deviations are not presented. (b) time to initial fibrin formation (R). (c) overall stability of the clot (MA).

Figure 5. Comparison of average clot stability (MA) measured using TEG to average platelet levels for surviving animals (purple and dark blue lines) and decedents (light blue and red lines). Note that MA values are plotted on the right axis due to magnitude differences between MA values and platelet counts. For ease in illustrating changes, the group standard deviations are not presented.

Individual coagulation factors II, V, VII, VIII, IX, X, XII, XIII, Protein C, fibrin monomers, antiplasmin and D-dimer were measured throughout the course of the study for each animal. Reproducibility of each assay was established using n = 20 control (non-irradiated) pig plasma samples repeated in duplicate. Baseline blood samples were obtained from all animals on two separate occasions prior to day of irradiation. Results were compared using a paired t-test. The results indicate that the differences between the two baseline activity levels were not statistically different. Therefore, both baseline results were averaged to calculate a single baseline activity level for each animal. Post irradiation results at all time points were normalized per animal against the respective average baseline to determine the percent change from baseline. The resulting positive and negative changes from baseline vary for each factor and at different time points, reflecting the complexity of the data (e.g. ).
Figure 6. Example of individual coagulation factor values for a single irradiated animal (Animal 3001, survivor to Day 45) over time. Date for each factor was normalized to the average baseline value for this animal to represent the data as a percent change from baseline.

Comparison of TEG, hematology and coagulation factors can help illuminate the underlying mechanism of coagulopathy dysfunction in this model. This is well illustrated by for non-surviving animal 3004 (euthanized for moribund condition post irradiation Day 18). For this animal, the TEG tracing () indicates increased time to initial clot formation (R time), decreased clot stability (low MA) and slow rate of clot formation (low α-angle) beginning at post irradiation Day 7 and progressing through post irradiation Day 15, with some improvement (increased MA) indicated with the moribund collection (Day 18). This is supported by comparison of platelet count with MA and α-angle values (). The slight increase in platelet count observed at Day 18 compared to Day 15 is reflected by both the MA and α-angle values. The TEG tracing also demonstrates a long time to initial clot formation (R time) observed at both post irradiation Days 15 and 18, suggestive of a deficiency in clotting factors as well as absent fibrinolysis post day 10. The change in each coagulation factor over time for this animal () suggests that the TEG tracing alterations observed on post irradiations Days 15 and 18 may be associated with change in activity of coagulation factors II, VII, VIII, XI, XIII, and antiplasmin.
Figure 7. (a) Clinical pathology comparison of blood parameters for Animal 3004, euthanized for moribund condition on post-irradiation Day 18, including a) TEG analysis results for time points as indicated by color coding. (b) Clinical pathology comparison of blood parameters for Animal 3004, euthanized for moribund condition on post-irradiation Day 18, including b) platelet levels versus MA and α-angle values from TEG analysis (note: MA and α-angle are plotted on the right axis due to magnitude differences between MA and α-angle values and platelet counts). (c) individual coagulation factor values for each time point. Data for each factor was normalized to the average baseline value for the animal to represent the data as a percent change from baseline.

Comparison of average change in coagulation factor activity levels from baseline following irradiation was compared between surviving animals and decedents. The greatest differences between these subsets of animals were observed with factors XIII (), XII (), protein C () and antiplasmin (). The difference between coagulation factor XIII levels between surviving animals and decedents was statistically significant at post irradiation Day 20. Regardless of statistical significance, with the exception of antiplasmin, the trend indicates coagulation factor activity levels for VII, XII, XIII, and protein C decrease as the animal approaches moribund condition. Interestingly, average activity levels of factor XII observed in decedents appear to decrease as early as post irradiation Day 4. In contrast, average antiplasmin activity levels increased in decedents compared to surviving animals, possibly indicative of a fibrinolytic shutdown.
Figure 8. (a) Average change in coagulation factor activity levels over baseline in male Gottingen minipigs following total body irradiation. Shown are the changes in the a) Factor XIII. For decedents, each data point represents the average of n = 6 animals, with the exception of Day 20 (n = 4) and Day 25 (n = 1). A single animal succumbed at Day 18 and is provided independently (‘terminal sample’). The Sham Control group consisted of n = 4 animals. For each in illustrating changes, the group standard deviations are not presented, however any statistical significance is noted by * (p < .002). (b) Factor XII. (c) protein C. (c) antiplasmin.

Discussion
The minipig has been proposed as an alternative large animal model to the canine and NHP for evaluation of medical countermeasures for treatment of acute radiation syndrome. Development of a coagulopathy-related model would have particular utility in characterizing the underlying mechanisms associated with coagulopathy (Olsen et al. Citation1999; Pliszczak-Krol et al. Citation2016) and associated multi-organ dysfunction (Gando Citation2010) as well as hemorrhage. This study was conducted as part of the overall BARDA consortium approach to evaluate multiple circulating markers associated with coagulopathy following total body irradiation of the Gottingen minipig. In the study described, 6 of 20 (30%) of the animals irradiated with 2.1 Gy either died or were euthanized for moribund condition. Based on clinical pathology analyses (hematology and TEG), it appears that all 6 animals developed a coagulopathy-related injury, including low platelet count, delayed clot formation, and decreased clot stability. These findings are consistent with cageside observations of petechia and/or ecchymosis occurring several days prior to death. Observation of petechiation (occurrence initially reported post irradiation Days 11–19) correlated with platelet nadir and occurred in all decedents and 3 surviving animals. Ecchymosis was observed at post irradiation Day 18 in a single animal euthanized on Day 20.
Although this study was focused on development of a large animal model of radiation-induced coagulopathy, the hematopoietic syndrome is characterized by hypoplasia or aplasia of the bone marrow. At the total body irradiation doses utilized here, most bone marrow progenitors are susceptible to cell death, leading to a hematologic crisis in the first weeks after exposure characterized by pancytopenia (Waselenko et al. Citation2004). The time to appearance of each hematopoietic symptom is dependent on the various transit times from stem cell to mature functioning cell. As illustrated by , development of thrombocytopenia in the decedent occurs concurrent with anemia and neutropenia, predisposing animals to infection and bleeding risk. Thus, the hematopoietic injury likely involves a combination of destruction of megakaryocyte progenitor cells and consumptive processes that use platelets, such as vascular injury or coagulopathy.
The data presented here should be considered preliminary due to a minimal number of samples from decedents for comparison to surviving animals, particularly at post irradiation Days 20 and beyond. Any lack of statistical significance should not be interpreted as a proof of no difference, as the precision of statistical comparisons was limited by the limited power inherent with the small sample size of non-surviving animals. However, despite this shortcoming, the information contained within these data does provide intriguing insight into the mechanism of coagulation dysfunction post radiation exposure. For example, identification of changes within TEG tracings over time coupled with fluctuations illustrated by individual coagulation factors implicates both the intrinsic and extrinsic pathways of the coagulation cascade. These preliminary findings continue to be assessed in on-going studies.
Disclosure statement
No potential conflict of interest was reported by the author(s).
Additional information
Funding
Notes on contributors
Karla D. Thrall
Karla D Thrall, PhD, DABT, is the Senor Director of Radiation Biology at Altasciences Preclinical Seattle, Everett WA.
Saikanth Mahendra
Saikanth Mahendra, MS, DABR, is a board-certified Medical Physicist at Northwest Medical Physics Center, Lynnwood, WA.
M. Keven Jackson
M. Keven Jackson, DVM, DACVP, is a board-certified research pathologist at Altasciences Preclinical Seattle, Everett WA.
References
- Blue R, Chancellor J, Suresh R, Carnell L, Reyes D, Nowadly C, Antonsen E. 2017. Challenges in clinical management of radiation-induced illnesses. IN: Exploration Spaceflight; [accessed 2020 Sep 16]. https://ntrs.nasa.gov/search.jsp?R=20170009925_2019-04-26T15:52:06+00:00Z.
- Chernyshenko V, Snezhkova E, Mazur M, Chernyshenko T, Platonova T, Sydorenko O, Lugovskoy E, Nikolaev V. 2019. Blood coagulation parameters in rats with acute radiation syndrome receiving activated carbon as a preventative remedy. UkrBiochemJ. 91(2):52–62.
- Esker J, Moyer B, Raulli RE, Grace M. 2015. Development of a large animal model of H-ARS in minipigs: natural history and biomarker results for radiation injury from multiple institutions using a harmonized model. 61st Annual Meeting of the Radiation Research Society; Weston FL.
- Fleming TR, Harrington DP. 1984. Estimation of the survival distribution in censored data. Comm in Stats Theory Methods 13:2469–2486.
- Gando S. 2010. Microvascular thrombosis and multiple organ dysfunction syndrome. Crit Care Med. 38(2 Suppl):S35–S42.
- Gutin PH, Iwamoto FM, Beal K, Mohile NA, Karimi S, Hou BL, Lymberis S, Yamada Y, Chang J, Abrey LE. 2009. Safety and efficacy of bevacizumab with hypofractionated stereotactic irradiation for recurrent malignant gliomas. Int J Radiat Oncol Biol Phys. 75(1):156–163.
- Lai A, Filka E, McGibbon B, Nghiemphu PL, Graham C, Yong WH, Mischel P, Liau LM, Bergsneider M, Pope W, et al. 2008. Phase II pilot study of bevacizumab in combination with temozolomide and regional radiation therapy for up-front treatment of patients with newly diagnosed glioblastoma multiforme: interim analysis of safety and tolerability. Int J Radiat Oncol Biol Phys. 71(5):1372–1380.
- Moroni M, Coolbaugh TV, Lombardini E, Mitchell JM, Moccia KD, Shelton LJ, Nagy V, Whitnall MH. 2011. Hematopoietic radiation syndrome in the Gottingen minipig. Radiat Res. 176(1):89–101.
- National Research Council (NRC). 2011. Guide for the care and use of laboratory animals. 8th Ed. Washington (DC): National Academies Press.
- Ohkita T. 1975. Review of thirty years study of Hiroshima and Nagasaki atomic bomb survivors, Part II: biological effects; A: acute effects. J Radiat Res. 16(supplement):49–66.
- Olsen AK, Hansen AK, Jespersen J, Marckmann P, Bladbjerg EM. 1999. The pig as a model in blood coagulation and fibrinolysis research. Scand J Lab Anim Sci. 26:214–224.
- Pliszczak-Krol A, Rzasa A, Gemra M, Krol J, Luczak G, Zyzak A, Zalewski D, Iwaszko-Simonik A, Graczyk S. 2016. Age-related changes of platelet and plasma coagulation parameters in young pigs. J Vet Diag Invest. 28(5):561–567.
- Robins HI, Won M, Seiferheld WF, Schultz CJ, Choucair AK, Brachman DG, Demas WF, Mehta MP. 2006. Phase 2 trial of radiation plus high-dose tamoxifen for glioblastoma multiforme: RTOG protocol BR-0021. Neuro-Oncology. 8(1):47–52.
- Stupp R, Mason WP, van den Bent MJ, Weller M, Fisher B, Taphoorn MJ, Belanger K, Brandes AA, Marosi C, Bogdahn U, et al. 2005. Radiotherapy plus concomitant and adjuvant temozolomide for glioblastoma. N Engl J Med. 352(10):987–996.
- Venkatesulu BP, Mahadevan LS, Aliru ML, Yang X, Bodd MH, Singh PK, Yusuf SW, Abe J, Krishnan S. 2018. Radiation-induced endothelial vascular injury: a review of possible mechanisms. J Am Coll Cardiol Basic Trans Sci. 3(4):563–572.
- Waselenko JK, MacVittie TJ, Blakely WF, Pesik N, Wiley AL, Dickerson WE, Tsu H, Confer DL, Coleman CN, Seed T, et al. 2004. Medical management of the acute radiation syndrome: recommendations of the strategic national stockpile radiation working group. Ann Intern Med. 140(12):1037–1051.
- Weintraub NL, Jones WK, Manka D. 2010. Understanding radiation-induced vascular disease. J Am Coll Cardiol. 55(12):1237–1239.