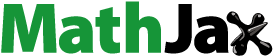
Abstract
The aim was to develop novel fibres by enzymatic synthesis, to determine their total dietary fibre by AOAC method 2009.01 and to estimate their potential digestibility and assess their digestibility in vivo using glycaemic and insulinaemic responses as markers in mice and randomised clinical trial models. We found that fibre candidates to which α-(1,2) branching was added were resistant to digestion in the mouse model, depending on the amount of branching. These results show that in vivo models are needed to reliably assess the digestibility of α-glycosidic-linked oligomeric dietary fibre candidates, possibly due to absence of brush border α-glucosidase activity in the current in vitro assessment. α-(1,3)-linked and α-(1,6)-linked glucose oligomers were completely digested in humans and mice. In conclusion, it is possible to develop dietary soluble fibres by enzymatic synthesis. Adding α-(1,2) branching increases their resistance to digestion in vivo and can thus improve their suitability as potential fibre candidates.
Clinical Trial Registry: ClinicalTrials.gov, NCT02701270
Introduction
Many health benefits have been attributed to soluble fibre intake, including the regulation of weight gain and glycaemia (Slavin Citation2005). Epidemiological evidence strongly supports that dietary fibres reduce the risk of obesity (Slavin Citation2005). Their consumption is inversely associated with body mass index (BMI), and an increase in fibre intake might help regulate glucose metabolism (Howarth et al. Citation2001; Slavin Citation2005). However, the actual intake of dietary fibres in many populations in Europe and the USA does not meet current recommendations (Agostoni et al. Citation2010; Jones Citation2013). One option for reducing this gap in dietary fibre intake is to develop novel soluble fibres that can be added easily to a variety of foods.
Dietary fibres are defined as carbohydrates with a degree of polymerisation (DP) of three or more monomeric units that are not digested or absorbed in the human small intestine. These fibres belong to the following categories: edible carbohydrate polymers that occur naturally in food; edible carbohydrate polymers that have been obtained from raw food material by physical, enzymatic or chemical means and that have a beneficial physiological effect as demonstrated by generally accepted scientific evidence; and edible synthetic carbohydrate polymers, which have a beneficial physiological effect as demonstrated by generally accepted scientific evidence (European Commission Citation2008). Indigestibility is a key basic characteristic of dietary fibre and must be demonstrated for any novel soluble fibre candidate (Raninen et al. Citation2011). In the caecum-colon, most fibres are hydrolysed to small oligomers and monomers, which are further metabolised by anaerobic bacteria (Delzenne & Roberfroid Citation1994; Roberfroid Citation1997). This metabolic process provides bacteria with energy for proliferation and generates gases (H2, CO2, CH4), which are metabolically useless to the host, and short-chain fatty acids (SCFAs), such as acetate, propionate, butyrate and L-lactate, some of which are believed to contribute to the beneficial physiological effects of fibres (Delzenne & Roberfroid Citation1994). Thus, although they do not supply the body with monosaccharides, fibres are indirect energy substrates and metabolic regulators (Delzenne & Roberfroid Citation1994).
We produced several novel soluble fibres candidates (FC) by enzymatic synthesis from sucrose as the raw material, yielding fructose and α-glycosidic-linked oligomeric dietary FC: α-(1,3)-linked glucose and α-(1,6)-linked glucose. The aim of our study was to determine how these FC support the growth of select probiotic, pathogenic and intestinal microbes; assess their capacity to produce gas in in vitro faecal suspensions; measure their fermentation in a colon simulator; and examine their digestibility in various models: the in vitro AOAC method 2009.01 (McCleary et al. Citation2010), used as an initial screen to determine total dietary fibre; a mouse model in which the glycaemic response of male mice was evaluated following a single dose of 2000 mg/kg body weight (bw); and an acute, single-blind, controlled, randomised and crossover clinical trial using the classical 240-min glucose tolerance test, with glycaemic and insulinaemic responses following ingestion of 20 g of fibre. Polydextrose (PDX), a glucose polymer and soluble fibre with low digestibility (Canfora & Blaak Citation2015), was used as a target reference for the development of fibre candidates. Based on the findings from the mouse model and human intervention study, we expanded our work to α-glycosidic-linked oligomeric dietary FC with α-(1,6)-linked glucose and additional α-(1,2) branching.
Materials and methods
HPLC analytical methods
Sucrose, glucose, fructose and leucrose were quantitated by HPLC using two Aminex HPX-87C columns (Bio-Rad, Hercules, CA) in series. The chromatographic conditions were 85 °C in the column; 40 °C in the sample, refractive index detector and injector compartments; deionised water as the eluent (flow rate of 0.6 mL/min) and an injection volume of 10 μL. Soluble oligosaccharides were quantitated by HPLC using an Aminex HPX-42A column (Bio-Rad, Hercules, CA) using the same conditions as above. Oligosaccharide samples from DP2 to DP7 were obtained from Sigma-Aldrich Corp. (St. Louis, MO): maltoheptaose (DP7, Cat.# 47872), maltohexanose (DP6, Cat.# 47873), maltopentose (DP5, Cat.# 47876), maltotetraose (DP4, Cat.# 47877), isomaltotriose (DP3, Cat.# 47884) and maltobiose (DP2, Cat.#47288). Each oligosaccharide was calibrated by refractive index detection.
Determination of glycosidic linkages
Glycosidic linkages of FC were examined by 1H NMR spectroscopy, and methylation was analysed by mass spectrometry (GC/MS); the former method analyses the intact soluble fibre but is sometimes unable to detect or distinguish between low concentrations of similar types of linkages (e.g. α-(1,3,4) from α-(1,3,6)) and the latter requires hydrolysis of chemically derivatised soluble fibre, which can result in small changes in the chemical composition of a sample.
One-dimensional 1H NMR data were acquired on a Varian Unity Inova system (Agilent Technologies, Santa Clara, CA) that was operated at 500 MHz using a high-sensitivity cryoprobe. Water suppression was obtained by carefully placing the observed transmitter frequency to resonance for the residual water signal in a “presat” experiment and then using the “tnnoesy” experiment with a full-phase cycle (multiple of 32) and a mix time of 10 ms.
Typically, dried samples were taken up in 1.0 mL D2O and sonicated for 30 min. From the soluble portion of the sample, 100 μL was added to a 5-mm NMR tube with 350 μL D2O and 100 μL D2O that contained 15.3 mM 4,4-dimethyl-4-silapentane-1-sulphonic acid sodium salt as an internal reference and 0.29% NaN3 as a bactericide. The abundance of each type of anomeric linkage was measured by integrating the peak area at the corresponding chemical shift. The percentage of each type of anomeric linkage was calculated from the abundance of the particular linkage and the total abundance of anomeric linkages from oligosaccharides.
The distribution of glycosidic linkages in glucans was determined separately by methylation analysis, or partial methylation analysis (Pettolino et al. Citation2012). This technique has several minor variations but always entails the following steps: (1) methylation of all free hydroxyl groups of the glucose units, (2) hydrolysis of the methylated glucan to individual monomer units, (3) reductive ring-opening to eliminate anomers and create methylated glucitols, in which the anomeric carbon is typically tagged with a deuterium atom to create distinctive mass spectra, (4) acetylation of the free hydroxyl groups (created by hydrolysis and ring opening) to create partially methylated glucitol acetates, also known as partially methylated products and (5) analysis of the resulting partially methylated products by gas chromatography, coupled to mass spectrometry or flame ionisation detection.
The partially methylated products include non-reducing terminal glucose units, linked units and branching points. The individual products are identified by retention time and GC/MS. The distribution of partially methylated products is the percentage (area %) of each product in the total peak area of all partially methylated products. The gas chromatographic conditions were as follows: a RTx-225 column (30 m × 250 μm ID ×0.1-μm film thickness, Restek Corp., Bellefonte, PA); helium as the carrier gas (0.9 mL/min constant flow rate); oven temperature programme starting at 80 °C (hold for 2 min) and then ramping 30 °C/min to 170 °C (hold for 0 min) and 4 °C/min to 240 °C (hold for 25 min); 1-μL injection volume (split 5:1) and detection using electron impact mass spectrometry (full-scan mode).
Preparation of fibre candidate 1 (samples FC1)
To prepare samples from group FC1, a 250-mL reaction that contained 450 g/L sucrose, 12.5 mL of a Bacillus subtilis crude protein extract that harboured glucosyltransferase GTF0974 from Streptococcus salivarius 57.I (GENBANK® Gene Index: 387760974 (Cheng et al. Citation2015)) and 0.188 mL of a Trichoderma reesei crude protein extract ultrafiltration (UF) concentrate that contained mutanase MUT3325 from Penicillium marneffei ATCC® 18224 (GENBANK® Gene Index: 212533325 (Cheng et al. Citation2015)) in distilled, deionised H2O was stirred at pH 5.5 and 47 °C for 21 h and heated to 90 °C for 30 min to inactivate the enzymes. The resulting mixture was centrifuged, and FC1 fibre was isolated from the supernatant by size exclusion chromatography (SEC) at 40 °C using Diaion UBK 530 (Na+ form) resin (Mitsubishi Chemical Holdings Corp., Tokyo, JP). The SEC fractions that contained oligosaccharides with a DP ≥3 were combined and concentrated by rotary evaporation to 5 wt% dry solids (DS) and freeze-dried to produce FC1 as a dry solid: sample FC1-1. The reaction and isolation procedure was repeated to produce a second sample of FC1: FC1-2. The reaction was repeated using glucosyltransferase from Streptococcus sp. C150 GTF-S (GENBANK® Gene Index: 495810459 (Cheng et al. Citation2015) instead of GTF0974, and the SEC-purified aqueous oligosaccharide fractions were combined, concentrated and spray-dried to generate a third sample of FC1: FC1-3.
Preparation of fibre candidate 2 (samples FC2)
Samples from group FC2 were prepared as FC1 samples, except that the reaction contained 12.5 mL of a Bacillus subtilis crude protein extract that harboured glucosyltransferase from Streptococcus mutans MT4239 [GENBANK® Gene Index: 3130088 (Cheng et al. Citation2015)] with additional C-terminal truncations in part of the glucan-binding domains [GTF0088-T1 (Cheng et al. Citation2015)] was employed as sole enzyme catalyst. The SEC fractions that contained oligosaccharides ≥ DP3 were combined and concentrated by rotary evaporation to 5 wt% (DS) and freeze-dried to produce FC2 as a dry solid: sample FC2-1. A second reaction was run using the same procedure, and the SEC-purified aqueous oligosaccharide fractions were combined, concentrated and spray-dried to yield a second sample of FC2: FC2-2.
Preparation of fibre candidates 3, 4 and 5 (samples FC3, FC4 and FC5)
Four 500-mL reactions, each containing 200 g/L sucrose and 9.44 U/mL of glucosyltransferase GTF8117 from Lactobacillus animalis KCTC 3501 [Gene Index: 335358117 (Cheng et al. Citation2015)] were adjusted to pH 5.5 and mixed at 47 °C on a rotary shaker for 18 h. The reactions were then heated at 90 °C for 20 min, cooled to ca. 25 °C and centrifuged; the resulting supernatants were combined and stored at 5 °C prior to use in a subsequent reaction that added α-(1,2)-glucosyl branching to the α-(1,6)-linked dextran polysaccharide reaction product.
Samples from group FC3 were prepared using two 250-mL jacketed resin kettles each charged with 100 mL of the GTF8117 reaction product supernatant (final concentration: 80 g/L of total dissolved solids derived from sucrose), 33.3 mL of a 600 g/L sucrose stock solution (final concentration of 80 g/L sucrose) and 91.7 mL distilled water. The mixtures were heated in situ at 80 °C for 30 min and cooled to 30 °C. Then, 25 mL of an Escherichia coli BL21 DE3 extract that contained 5.0 U/mL of the α-(1,2)-branching glucosyltransferase GTFJ18T1 (a truncated version of GTFJ18 from Leuconostoc mesenteroides subsp. mesenteroides J18, comprising part of a glucan-binding domain (GBD) and the CD2 catalytic domain with a-(1,2) branching activity; GENBANK® Gene Index: 356644413 (Cheng et al. Citation2015)), was added, and the pH was adjusted immediately to 5.5 with 0.5% sodium hydroxide. The pH in the reaction was continuously controlled at 5.5 with a pH electrode that was connected to a peristaltic pump that fed 0.5% sodium hydroxide into the mixture as needed. After 44 h, two separate reactions (samples FC3-1 and FC3-2) were heated to 90 °C for 20 min, the heat-treated mixture was centrifuged and the resulting supernatant was analysed by SEC for molecular weight.
The supernatants from reactions FC3-1 and FC3-2 were combined, and the α-(1,2)-branched polysaccharides were purified and isolated by UF using a 5 × 103 g/mol molecular weight cut-off (MWCO) polyethersulfone (PES) membrane (Pall Centramate™ LV, Pall Corp., Port Washington, NY). HPLC analysis of the UF retentate indicated no detectable monosaccharides, disaccharides or DP2 to DP8 oligosaccharides. The UF retentate was adjusted to ca. 5 wt% dissolved solids, and the resulting solution was lyophilised to produce the soluble α-(1,2)-branched polysaccharides as a dry solid (sample FC3-3) which was analysed by 1H NMR spectroscopy and GC/MS to determine the anomeric linkages in the polysaccharides.
Samples from group FC4 (FC4-1, FC4-2 and FC4-3) were prepared as the FC3 samples, except that the reaction time was decreased from 44 h to 4 h for each of the two reactions prior to combination and product isolation by UF (i.e. samples FC4-1 and FC4-2).
Group FC5 samples (FC5-1, FC5-2 and FC5-3) were also prepared as the FC3 samples, except that the two reaction mixtures (FC5-1 and FC5-2) contained 175 mL of the GTF8117 reaction product supernatant (final concentration: 140 g/L of total dissolved solids derived from sucrose), 4.2 mL of a 600 g/L sucrose stock solution (final concentration of 10 g/L sucrose), 45.8 mL distilled water and 25 mL of GTFJ18T1 enzyme solution; the reaction time was 20 h.
Growth of select microbes
To determine how FC1-2 and FC2-1 supported the growth of select probiotic, pathogenic and intestinal microbes, Clostridium perfringens ATCC 3626, Clostridium difficile DSM 1296, Escherichia coli ATCC 11775, Salmonella typhimurium EELA, Lactobacillus acidophilus NCFM and Bifidobacterium animalis subsp. lactis Bi-07 were cultured anaerobically in a Bioscreen® C system (Labsystems, Helsinki, FI) as described (Mäkeläinen et al. Citation2010). PDX and dextrose were used as low and high fermentable controls, respectively. Briefly, the test strains were grown in the appropriate media, to which the test substances were added as the sole energy and carbon source at 1% (w/v). Growth was measured automatically every 30 min, based on the absorbance at 600 nm, and expressed as area under the curve values (OD600 × 24 h) ± SD. Measurements were performed in triplicate.
In vitro gas production
To measure gas production in vitro, FC1-2 and FC2-1 were added to a faecal suspension to a final concentration of 1% (w/v). PDX and dextrose were used as low and high fermentable controls, respectively. The faecal suspension was prepared by diluting a fresh faecal sample from a healthy human donor 1:3 in synthetic ileal fluid under anaerobic conditions and filtered through 0.3-mm metal mesh, after which it was incubated anaerobically for 24 h at 37 °C as described (Mäkivuokko et al. Citation2005). Nine millilitres of the suspension were mixed with 1 ml 10% (w/v) test substance in a gastight, rubber-capped vial that was connected to gastight glass syringe. Gas formation was read after 3 and 24 h. Results are presented as the average of at least three independent observations with faecal material from several donors.
Colon simulation
Fermentation of samples FC1-2 and FC2-1, as well as the low digestible PDX control, was mimicked using a colon simulation model as described (Mäkivuokko et al. Citation2005; Mäkeläinen et al. Citation2010). Each simulator consisted of four semi-continuously connected glass vessels. The vessels in a unit (V1-V4) model the various sections of the human colon, from the proximal to distal regions, each with a different pH and flow rate. Vessels V1-V4 represent the ascending, transverse, descending and sigmoid colon, respectively. At the start of a simulation, each unit was inoculated with pre-incubated faecal microbes, which constituted the microbiota of the colonic model. Faecal donors had not used antibiotics or laxatives for at least 3 months before donation or probiotic bacteria for 3 weeks before donation. The test substrates were added to a synthetic ileal medium (2%, w/v) (Mäkivuokko et al. Citation2005) and fed to the colon model for 48 h in 3-h cycles, during which the fermented fluids and microbes transitioned and fresh medium was supplied. Anaerobic conditions were maintained by flushing the simulator with gaseous nitrogen. At the end of the simulations, the microbial slurry was collected from all vessels and frozen until the metabolic analysis to determine the influence of the test components on the activity of the microbiota. Simulations were performed in triplicate for each test component and blank (no component added). SCFAs and branched-chain fatty acids (BCFAs) were determined by gas chromatography as described (Ouwehand et al. Citation2009).
Potential digestibility in vitro
The potential digestibility of samples from groups FC1 (FC1-1, FC1-2 and FC1-3), FC2 (FC2-1 and FC2-2), FC3 (FC3-3), FC4 (FC4-3) and FC5 (FC5-3) were assessed using the test protocol adapted from the Megazyme Integrated Total Dietary Fiber Assay AOAC 2009.01 method (McCleary et al. Citation2010). The final enzyme concentrations were the same as in the AOAC method: 50 units/mL of pancreatic α-amylase and 3.4 units/mL of amyloglucosidase. The substrate concentration in each reaction was 25 mg/mL per the AOAC method. The total volume of each reaction was 1 mL, instead of 40 mL as suggested by the original protocol. Every sample was analysed in duplicate with and without the two digestive enzymes.
The detailed procedure is as follows: the enzyme stock solution was prepared by dissolving 20 mg of purified porcine pancreatic α-amylase [150,000 units/g; AOAC Method 2002.01 (McCleary & Monaghan Citation2002)] from the Integrated Total Dietary Fiber Assay Kit in 29 mL sodium maleate buffer (50 mM, pH 6.0 plus 2 mM CaCl2) and stirring for 5 min, after which 60 μL amyloglucosidase solution (AMG, 3300 units/mL) from the same kit was added. Then, 0.5 mL of the enzyme stock solution was mixed with 0.5 mL soluble fibre sample (50 mg/mL) in a glass vial, and the digestion reaction was incubated at 37 °C with 150 rpm orbital motion on a shaking incubator for exactly 16 h. Duplicate reactions were run in parallel for each fibre sample. The control reactions were performed in duplicate by mixing 0.5 mL maleate buffer (50 mM, pH 6.0 plus 2 mM CaCl2) and 0.5 mL soluble fibre sample (50 mg/mL) and incubating them at 37 °C with 150 rpm orbital motion on a shaking incubator for exactly 16 h.
After 16 h, all samples were removed from the incubator, and 75 μL of 75 M Trizma® (Sigma-Aldrich Chemie GmbH, Munich, Germany) base solution was added immediately to terminate the reaction. The vials were placed immediately into a heating block at 95–100 °C and incubated for 20 min with occasional shaking (by hand). The total volume of each reaction mixture was 1.075 mL after quenching. The amount of glucose that was released in each reaction was quantified by HPLC with Aminex HPX-87C columns (BioRad, Hercules, CA) as described in General Methods. Maltodextrin (DE4-7, Sigma-Aldrich Cat. #419672, St. Louis, MO) was used as the positive control for the enzymes. The digestibility was calculated per the following formula:
where, in the denominator, the weight of available glucose that can be released per weight of fibre is determined by multiplying the weight of fibre by the molecular weight of free glucose (180 g/mol) and dividing the result by the molecular weight of the individual glucosyl residues that constitute the fibre (162 g/mol); the equivalent of one water molecule (18 g/mol) is lost in the formation of each anomeric linkage in the fibre.
Assessment of digestibility in mice
The digestibility of samples FC1-2, FC2-1, FC3-3, FC4-3 and FC5-3 was measured as the glycaemic response compared with high-digestibility (PSF and dextrose), low-digestibility (PDX) and blank (water) controls in two mouse experiments.
Male C57Bl/6J mice were acquired from The Jackson Laboratory (Bar Harbor, ME) and maintained per the Guide for the Care and Use of Laboratory Animals (National Research Council, 2011) under the oversight of the Institutional Animal Care and Use Committee (IACUC; protocols AT312-P & AT315-P) at DuPont Haskell Global Centers for Health and Environmental Sciences (Newark, DE), an Association for Assessment and Accreditation of Laboratory Animal Care-accredited test facility. Animals were housed individually in solid-bottom cages with bedding and nesting material, with ad libitum access to water and certified feed (except when fasted). Animal rooms were maintained on a 12-h light/dark cycle (fluorescent light) at 22 ± 4 °C and a relative humidity of 50 ± 20%. Testing was conducted in mice at age 9–12 weeks.
Two experiments were conducted using separate shipments of animals. In Experiment #1, six groups of 12 mice were dosed by oral gavage with one of six treatments: two test substances [FC1 and FC2 (samples FC1-2 and FC2-1, respectively)], chromatographic purified (DP >2) Sucromalt (XTEND® Sucromalt™, Cargill, Minneapolis, MN) fraction (PSF) as a positive control, vehicle control (deionised water), PDX as a target reference (Litesse®, DuPont, Terre Haute, IN) and dextrose as a standard reference control. In Experiment #2, seven groups of 12 mice were dosed by oral gavage with one of seven treatments: three test substances [FC3, FC4 and FC5 (samples FC3-3, FC4-3 and FC5-3, respectively)], a vehicle control (deionised water), PDX as a target reference, dextrose as a reference control and the FC1 test substance (sample FC1-2), which served as a positive control in this experiment, based on the results of Experiment 1. In both experiments, the dose was 2000 mg/kg bw for all treatments except for the water control, which was administered at the same dose volume (10 mL/kg) as the other treatments.
Animal were fasted for 6 h prior to dosing and continued to be fasted throughout the 2-h observation period. The experiments were performed after habituating the animals to the testing procedure, including daily gavage with water for 3 days, and three baseline glucometer readings within approximately 1 h prior to dosing. Post-dose glucometer readings were taken after approximately 20, 40, 60 and 120 min. The Alpha Trak 2 glucometer (Abbott Laboratories, Chicago, IL) was used to measure glucose in at least 0.3 microliters of blood, obtained by pricking the tail vein with a sterile needle. For each animal, the iAUC was calculated using the trapezoidal rule (Brouns et al. Citation2005). The relative iAUC response to the dextrose control is expected to reflect digestibility, calculated as follows:
Assessment of digestibility in humans
Our objective was to assess the digestibility of FC1 (sample FC1-3) and FC2 (sample FC2-2) by evaluating the glycaemic and insulinaemic responses of healthy adults following a single dose as compared to high-digestibility (dextrose) and low-digestibility (PDX) controls. The clinical trial was conducted at Leatherhead Food Research Ltd. (Surrey, UK) and was sponsored by DuPont Nutrition and Health (Reigate, UK). The protocol was approved by the NRES Committee South East Coast – Brighton & Sussex, was performed according to the Declaration of Helsinki (WMA Citation2001), followed Good Clinical Practice guidelines (ICH Citation1996) and was registered at ClinicalTrials.gov (NCT02701270). The intervention took place in the autumn of 2015. The site and laboratories were audited by Medigno Oy (Veikkola, FI), and the study was reported per the CONSORT statement (Schulz et al. Citation2010).
The study had an acute, randomised, single-blind, controlled and crossover design. Twelve healthy participants were included after being informed and giving written consent; they were aged between 18 and 65 years; and their BMI was between 18 and 27 kg/m2. Eligible participants were willing to give blood and assured us that they would not donate blood during the study; they did not report more than 10 h of intense sports activities or consumption of over 21 units of alcohol per week. Participants were excluded if they had any health condition that prevented them from fulfilling the study requirements, had a history of diabetes or high blood pressure, had fasting blood glucose levels of less than 4.4 or more than 6.1 mmol/L or disliked the investigational products or had allergies toward any of them. Female participants were excluded if they were lactating, pregnant or planning to become pregnant during the study. Also, subjects were excluded if they were involved in other clinical trials or consumed anything except plain water during the last 12 h prior to the test; in the latter case, the participant was asked to return for an extra session. All adverse events were controlled for during the study.
In the formulations, the two experimental fibres and PDX (low-digestibility control) were balanced to contain the same amount of fibre (20.00 g) and available carbohydrates (1.74 g). The dextrose (high-digestibility control) did not contain fibre, but a total of 21.74 g of available carbohydrates. All test substances were white- to cream-coloured powders and odourless and had a bland and neutral taste. All four formulations were mixed with 250 mL water with several drops of flavouring for the test challenges.
On the days of the visits, after an investigational product challenge, the glycaemic and insulinaemic responses were measured by finger prick blood sampling at 0, 15, 30, 45, 60, 90, 120, 150, 180, 210 and 240 min per a well-established method (Brouns et al. Citation2005); because FC1-3 and FC2-2 are experimental fibres that were tested for the first time in humans, the experimental length was increased from the typical 120 min to 240 min to rule out any possible delay in absorption. Blood samples were collected into small tubes that contained lithium-heparin following a finger prick and centrifuged at 3000 rpm for 10 min to separate the plasma. Glucose was analysed on a 2300 STAT Plus™ Glucose & Lactate Analyzer (YSI Inc., Yellow Springs, OH), and insulin was measured using the Human Insulin ELISA kit (Thermo Fisher Scientific Inc., Waltham, MA). Glycaemic and insulinaemic responses were expressed as iAUC values for 4 h using the trapezoidal rule (Brouns et al. Citation2005) and as the relative response to the dextrose as a percentage.
Statistical analysis
Treatments in the colon simulator and gas formation were compared against the blank and PDX control by Student’s t-test using Excel (Microsoft, Redmond, WA). The numbers of subjects in the animal and clinical trials were based on an established method for glycaemic index testing (Brouns et al. Citation2005), which claims that a sample size of 10 subjects is sufficient to detect differences in glucose responses. However, 12 subjects were included in each study to account for an estimated 10% dropout rate. In the clinical trial, the 12 volunteers were randomised by date of entry and the order of events, applying Latin square randomisation. The randomisation list was generated by the statistician at Leatherhead Food Research Ltd. and only the volunteers were blinded. We assumed that the gender ratio would be 1:1 and that BMI and age limits, as set in the inclusion criteria, would not affect the outcome of the study. One-way ANOVA with a 95% confidence level was used to analyse the animal and clinical trials (Snedecor & Cochran Citation1967). Transformations were applied (log, square root or rank order) when appropriate, based on preliminary tests for homogeneity of variance (Levene Citation1960) and normality (Ryan & Joiner Citation1976). Pairwise differences were established by Tukey’s test (Tukey Citation1949). The data were analysed using Minitab® (Minitab Inc., State College, PA) or XLSTAT 2015 (Addinsoft, New York, NY).
Results
Fibre development and characterisation
Five groups of enzymatically produced soluble fibres were developed using sucrose as raw material: FC1, FC2, FC3, FC4 and FC5. These fibres were alpha 1,3- and 1,6-linked glucose oligo/polymers, similar to PDX. Their fibre content was at least 85% (McCleary et al. Citation2013), as measured by AOAC method 2009.01 (McCleary et al. Citation2010), and included residual levels of sugars, such as glucose, fructose, leucrose and sucrose.
Polysaccharides with low molecular weight (e.g. lower than DP ca. 25 or molecular weight lower than 4000) were characterised by SEC. The DP values for the soluble oligosaccharide fibre candidates FC1 and FC2 are listed in . The molecular weight analysis of the α-(1,2)-branched polysaccharides that were produced in reactions for the soluble oligosaccharide fibres FC3, FC4 and FC5 is shown in . The anomeric linkage analysis by 1H NMR and GC/MS of all soluble oligosaccharides fibres that were developed is presented in . 1H NMR determines the anomeric protons of the hydrolysed oligosaccharide or polysaccharide samples, whereas GC/MS measures linkages based on derivatisation of hydrolysed oligosaccharide or polysaccharide sample. The GC/MS method for linkage determination was more sensitive than 1H NMR spectroscopy for detecting low relative concentrations of anomeric linkages.
Table 1. Degree of polymerisation (DP) for soluble FC1 oligosaccharide fibres produced by GTF0974/mut3325 mutanase (FC1-1, FC1-2) or GTF-S/mutanase3325 (FC1-3) and for soluble FC2 oligosaccharide fibres produced by GTF0088T1 (FC2-1 and FC2-2).
Table 2. Molecular weight analysis of α-(1,2)-branched polysaccharides produced in reactions for soluble oligosaccharide fibres FC3 (FC3-1and FC3-2), FC4 (FC4-1and FC4-2) and FC5 (FC5-1 and FC5-2).
Table 3. Anomeric linkage analysis of soluble oligosaccharide fibres by 1H NMR and GC/MS.
Growth of select microbes
Test substances FC1-2 and FC2-1 supported little microbial growth, differing significantly from the blank (without any additional carbon or energy source) and PDX (p < .05), except with regard to Clostridium difficile and Clostridium perfringens, respectively. Growth was substantially lower than in the presence of dextrose (p < .001), except for Clostridium difficile and FC1-2 and Bifidobacterium lactis Bi-07 and FC2-1 (p > .05) ().
Table 4. Growth of select pure cultures with soluble oligosaccharide fibres, expressed as area under curve (OD600 × 24 h).
In vitro gas formation
Incubation of FC1-2 and FC2-1 with a faecal slurry resulted in the formation of slightly more gas than the blank at 3 h (p = .031 and 0.004, respectively). FC1-2, but not FC2-1, produced slightly more gas than the blank at 24 h (p = .039 and 0.095, respectively). There was no difference in gas production compared with PDX at 3 or 24 h ().
Table 5. In vitro gas formation (ml) by faecal slurry containing 1% (w/v) soluble oligosaccharide fibres.
Colon simulation
Compared with the control simulation, in which no oligosaccharides were added to the synthetic ileal medium, FC1-2 and FC2-1 led to the production of more total SCFAs in vessels V3 and V4 (p < .05) and in vessels V1-V4 (p < .05), respectively (). FC1-2 led to greater production of acetate in vessels V3-V4 (p < .01), butyrate in vessel V4 (p < .05) and lactate in vessels V1-V4 (p < .05) compared with the control. FC2-1 increased the production of acetate in vessels V1-V4 (p < .01), butyrate in vessels V3 and V4 (p < .05) and lactate in vessel V2 (p = .01) compared with the control (). Versus PDX, FC1-2 resulted in similar SCFA levels (p = .14), whereas FC2-1 effected higher levels of total SCFAs in vessels V3-V4 (p ≤ .01) (). Total BCFA content was lower for both FC1-2 (p ≤ .001) and FC2-1 (p ≤ .01) compared with the control in all vessels (). FC2-1 also effected lower production of isovalerate (p < .01) and 2-methyl butyrate (p < .05) compared with the control in all vessels (). Relative to PDX, total BCFA production did not differ with FC1-2 (p ≥ 0.1) but was higher with FC2-1 in vessels V3 and V4 (p ≤ .05) ().
Figure 1. (A) Production of short-chain fatty acids (SCFAs) and (B) branched-chain fatty acids (BCFAs) after 48-h colon simulation with oligosaccharide fibres FC1-2 and FC2-1, blank control and polydextrose. V1, V2, V3 and V4 indicate ascending, transverse, descending and sigmoid colon, respectively. Values are expressed as mean ± SD. *p < .05 calculated by pairwise Student’s t-test.

Potential digestibility in vitro
The potential digestibility in vitro of the fibre candidates and the maltodextrin and PDX controls, by the AOAC method 2009.01 (McCleary et al. Citation2010), is presented in .
Table 6. Digestibility of soluble oligosaccharide fibres and polydextrose and maltodextrin controls, according to AOAC method 2009.01 (McCleary et al. Citation2010).
Assessment of digestibility in mice
The overall initial values of blood glucose in the mouse studies were 15.8 (SD 2.6) mmol/L and 14.8 (SD 2.5) mmol/L for Experiments #1 and #2, respectively. The baseline-adjusted curves are shown in . Differences in blood glucose between treatments were observed within the first 20 min of the experiments. In both experiments, blood glucose returned to baseline levels at 120 min. Throughout Experiment #1, no statistically significant differences were observed when FC1-2, FC2-1 and PSF were compared with the dextrose control, whereas the responses to PDX and water were significantly lower than for dextrose at 20, 40 and 60 min (p < .05). In Experiment #2, FC1-2 performed similarly to the dextrose control. FC3-3, FC5-3, PDX and the vehicle (water) performed comparably with each other; all of these treatments were significantly lower than FC1-2 and the dextrose control at 20 min and 40 min (p < .05). The FC4-3 response lay between those of the other treatments at 20 and 40 min, with mean values that were slightly higher than for FC3-3, FC5-3, PDX and the vehicle and lower than for FC1-2 and dextrose (not statistically significant).
Figure 2. (A) Effects of FC1-2, FC2-1, PSF (purified Sucromalt fraction), water, polydextrose and dextrose on glycaemic responses in mice (n = 12) and (B) effects of FC1-2, FC3-3, FC4-3, FC5-3, water, polydextrose and dextrose on glycaemic responses in mice (n = 12). Values are expressed as mean ± standard error. Values with different letters are significantly different (p < .05) by Tukey’s test.

The glycaemic responses for the two mouse studies were expressed as iAUC values, as seen in . In Experiment #1, glycaemic responses did not differ significantly when FC1-2, FC2-1 and PSF were compared with the dextrose control, whereas the responses to PDX and the vehicle (water) were significantly lower than for dextrose (p < .05). In Experiment #2, the glycaemic response to FC1-2 was similar to that of the dextrose control, whereas the responses to FC3-3, PDX and the vehicle were significantly lower than for dextrose (p < .05). The iAUC values for FC4-3 and FC5-3 were similar to each other, slightly higher than those for FC3-3, PDX and the vehicle, and lower than for FC1-2 and dextrose (not statistically significant).
Table 7. Glycaemic responses expressed as incremental area under the curve (iAUC) and response relative to dextrose (%) for FC1-2, FC2-1, FC3-3, FC4-3, FC5-3, PSF, water, polydextrose and dextrose in two mouse studies.
In Experiment #1, the baseline values were lower (data not shown) for the FC1-2 group than for the other treatment groups. Because the responses were calculated relative to baseline, the magnitude of the spike in blood glucose and the iAUC were higher for this group than for dextrose in the same experiment. This difference was not statistically significant and was not repeated in Experiment #2. In addition, there was no reason to expect FC1-2 to produce more blood glucose than the direct administration of dextrose. Thus, the variability for FC1-2 in Experiment #1 was considered to be a spurious artefact.
Digestibility in humans
The formulations of the substances were tested in a clinical trial, as presented in . Twelve participants were enrolled, and their baseline characteristics are shown in . A flow diagram of the trial is depicted in . There were no adverse events during the study. One participant abandoned the study prematurely, and two participants had incomplete datasets during the insulin analysis and were thus unsuitable for inclusion in the statistical analysis of this parameter. Ultimately, 11 participants were included in the glucose analysis, and 9 constituted the insulin analysis.
Figure 3. Consolidated Standards of Reporting Trials (CONSORT) flow diagram of this randomised, single-blind, placebo-controlled and four-arm crossover (1:1:1:1 ratio) study.

Table 8. Formulation of the investigational products tested in the clinical trial.
Table 9. Baseline characteristics of the participants in the clinical trial.
The initial blood glucose and insulin values were 5.2 (SD 0.3) mmol/L and 3.5 (SD 0.7) μU/mL, respectively. The blood glucose and insulin curves, adjusted to baseline, are shown in . During the 4-h experiment, FC1-3 and FC2-2 behaved similarly to the dextrose control. Blood glucose levels in the PDX treatment were significantly lower than in the other groups during the first 45 min of the experiment (p < .05); at 60 min, the differences with other groups disappeared, although there was a small, significant difference versus FC1-3 at 150 min (p < .05). Similarly, PDX effected significantly lower insulin levels during the first 45 min; at 60 min, this difference was maintained only against FC2-2 (p < .05), and after 90 min, there were no differences with other treatments.
Figure 4. Effects of FC1-3, FC2-2, polydextrose and dextrose on (A) glycaemic (n = 11) and (B) insulinaemic (n = 9) responses in healthy humans. Values are expressed as mean ± standard error. Values with different letters are significantly different (p < 0.05) by Tukey’s test.

The glycaemic and insulinaemic responses, expressed as iAUC, to the four treatments are shown in . There was a significant difference for both parameters by one-way ANOVA (p < .001). By pairwise comparison, the glycaemic responses to the two experimental fibres were similar to that of the dextrose control and significantly different compared with PDX (p < .05). Similar results were observed for the iAUC insulinaemic responses in the pairwise comparison (p < .05).
Table 10. Glycaemic and insulinaemic responses expressed as incremental area under the curve (iAUC) and response relative to dextrose (%) for FC1-3, FC2-2, polydextrose and dextrose in the clinical trial.
Discussion
Historically, dietary fibre has been defined as non-digestible carbohydrates and lignin that are intrinsic to and intact in plants (Champ et al. Citation2003). In our study, we produced soluble α-glucan fibres candidates using sucrose and a novel method (Cheng et al. Citation2015). Over half of the composition of the samples from group FC1 had a DP predominately in the range of from 3 to 8 and an estimated average molecular weight of 870 g/mol, whereas samples from group FC2 were more polymerised, with DP predominately in the range of from 3 to 12 and an estimated average molecular weight of 1270 g/mol. The other three fibre candidates (samples from groups FC3, FC4 and FC5) were even larger, with average molecular weights of roughly 2 × 104 g/mol. Thus, the fibre candidates were comparable with other low- to medium-molecular-weight dietary fibres in terms of DP and molecular weight (Wilson et al. Citation2004; Andersson et al. Citation2009).
FC1 samples contained primarily α-(1,3) linkages, compared with α-(1,6) linkages for samples from group FC2; FC3, FC4 and FC5 did not contain α-(1,3) bonds. Samples from groups FC3 and FC4 were primarily composed of α-(1,2), α-(1,2,6), α-(1,6) and α-(1,2) branching linkages. Samples from group FC3 had a homogeneous distribution of these linkages, of which α-(1,2) branching predominated, whereas those from FC4 were richer in α-(1,6) bonds. Samples from group FC5 contained primarily α-(1,6) linkages.
With regard to the substances that were used as highly digestible controls in the in vitro and animal experiments, maltodextrin is formed from units of glucose with α-(1,4) bonds (Eliasson Citation2006), whereas PSF (purified Sucromalt fraction) has an average DP of 10 and comprises glucoses that are linked by alternating α-(1,3) and α-(1,6) linkages, except for the terminal glucose molecules, which are joined by an α-(1,4) bond (Grysman et al. Citation2008; Lee et al. Citation2016). PDX, the low-digestible control, has an average DP of 12, a molecular weight of approximately 2 × 103 g/mol, and all various combinations of α- and β-linked (1,2), (1,3), (1,4) and (1,6) glycosidic linkages, of which (1,6) linkages predominate (Lahtinen et al. Citation2010), but it is not easily digested due to its highly branched polymeric structure (Craig et al. Citation1998).
In vitro, FC1-2 and FC2-1 behaved differently from PDX and the blank control (no carbon source) in supporting the growth of the pure cultures, except for Clostridium difficile and Clostridium perfringens, respectively. On inoculation with faecal slurry, FC1-2 and FC2-1 tended to effect the formation of more gas after 3 and 24 h than the blank control but did not differ from PDX. Fermentation in the colon simulator also led to the production of SCFAs and BCFAs, wherein FC1-2 behaved similarly to PDX. Thus, it appears that FC1-2 and FC2-1 are not easily degraded in pure cultures and that they require complex microbiota for degradation, similar to PDX (Mäkeläinen et al. Citation2007, Citation2010Citation).
The most widely used in vitro assay to determine total dietary fibre content is AOAC method 2009.01 (McCleary et al. Citation2010), which uses pancreatic α-amylase and amyloglucosidase to mimic carbohydrate digestion. In our study, the in vitro method predicted the digestibility of the maltodextrin control well, over 90% of which was hydrolysed. PDX, the low-digestibility control, had a negligible rate of hydrolysis of less than 1%, as expected, based on previous reports (Jie et al. Citation2000). All fibre candidates showed low digestibility in the in vitro assay; however, samples from group FC1 and, in particular, FC2 samples underwent more extensive hydrolysis than the PDX control. Samples from groups FC3, FC4 and FC5 were digested to a similar extent as PDX.
However, due to the absence of brush border enzymes in this assay, the AOAC method 2009.01 has limitations, particularly with regard to glucose-based α-glycosidic-linked oligomeric dietary fibre candidates (Tanabe et al. Citation2014). An improved in vitro method that includes porcine small intestinal enzymes has been proposed to compensate for the lack of brush border enzymes (Tanabe et al. Citation2015); however, as the use of porcine brush border enzyme preparations has not been standardised or widely applied, we decided to use the described in vivo mouse model to further study if our fibre candidates were indeed indigestible; and subsequently we validated the mouse model by testing the same fibre candidates (samples from groups FC1 and FC2) in a human intervention study. Our findings confirm clearly that the current AOAC in vitro method does not predict digestibility in vivo for these types of fibres and can only be used to analyse total dietary fibre chemically and compositionally.
In the two animal experiments, the glycaemic responses to dextrose and water were consistent with our expectations and supported the validity of the test methods. Dextrose resulted in a sharp increase and gradual decline in blood glucose, whereas water alone did not result in any notable rise in blood glucose.
In the first animal experiment, PSF (purified Sucromalt fraction), FC1-2 and FC2-1 were generally similar to the high-digestible dextrose control with regard to the onset and magnitude of the spike in blood glucose. These substances contained primarily α-(1,3) and α-(1,6) linkages. These findings are consistent with previous reports on Sucromalt, which is composed of similar linkages and is hydrolysed to glucose in in vitro and human models (Grysman et al. Citation2008; Lee et al. Citation2016).
In the second animal experiment, the effects of FC1-2 were similar to the high-digestibility dextrose control, which was generally consistent with the previous animal assay. FC3-3, rich in α-(1,2,6), α-(1,6) and particularly α-(1,2) branching linkages, behaved similarly as the PDX (low-digestibility) and vehicle (water) controls, resulting in no increase in blood glucose. This observation is consistent with earlier reports that have indicated that substances that are rich in α-(1,2) linkages (e.g. kojibiose) are hydrolysed more slowly than those that are rich in α-(1,3) (e.g. nigerose) and α-(1,6) linkages (e.g. isomaltose) (Yamamoto et al. Citation1999; Chaen et al. Citation2001; Lee et al. Citation2016). The glycaemic responses to FC4-3 and FC5-3, both of which have abundant α-(1,6) linkages, exceeded those to the PDX and vehicle controls but had lower peaks and smaller iAUC values than the dextrose control.
The clinical trial confirmed that FC1-3 and FC2-2, which are rich in α-(1,3)- and α-(1,6)-linked glucose oligomers, are completely digested in humans, like dextrose. As expected, the low-digestibility PDX control induced a small increase in blood glucose and insulin relative to baseline that was greater than what has been reported (Jie et al. Citation2000), most likely due to the dextrose that was added to balance the total available carbohydrates in the treatments.
Our results suggest that samples from groups FC1 and FC2, which are rich in α-(1,3) and α-(1,6) linkages, are digested like dextrose when glycaemic and insulinaemic responses are used as markers. These observations are consistent with previous in vitro and in vivo experiments on Sucromalt, which is composed of the same types of linkages (Grysman et al. Citation2008; Lee et al. Citation2016). FC3-3 had the lowest rate of digestibility, most likely due to the presence of α-(1,2), α-(1,2,6) and particularly α-(1,2) branching linkages. FC4-3 and FC5-3, containing α-(1,2), α-(1,2,6) and α-(1,2) branching linkages but particularly rich in α-(1,6) linkages, were slightly (1%) digested in vivo. Thus, these results indicate that fibre candidates to which α-(1,2) branching is added are resistant to digestion in the animal model, depending on the amount of branching.
Conclusions
In conclusion, it is feasible to develop fibre candidates by enzymatic synthesis and demonstrate indigestibility using animal models, as well as clinical trials. However, most likely as a consequence of the lack of brush border α-glucosidase activity in the current AOAC method that was used to determine total dietary fibre content in vitro, more physiologically relevant in vivo models are needed to reliably assess the digestibility of α-glycosidic-linked oligomeric dietary fibre candidates. α-(1,6)-linked and α-(1,3)-linked glucose oligomers are completely digested in humans and mice. Adding α-(1,2) branching increases the resistance to digestion in vivo, a key property of a dietary fibre.
Acknowledgements
All authors were employees of DuPont during the study and declare no other conflicts of interest. O.H. coordinated the study. R.D., Z.Y., Q.C., S.C.R., S.S., Z.C.B., B.M.R., K.D.R.-J., J.P.L., R.E.H., M.L.M. and A.D.K. developed the fibre candidates and performed the digestibility in vitro. A.C.O. and S.D.F. designed and conducted the simulations in vitro. P.M., J.M.C.R., Y.P.D. and J.R.D. designed and performed the mouse experiments. K.T. and A.I. coordinated the clinical trial. All authors participated in the preparation and revision of the manuscript and gratefully acknowledge help from the personnel of E.I. DuPont de Nemours & Co. and Leatherhead Food Research Ltd.
Disclosure statement
The study was fully sponsored by E.I. DuPont de Nemours & Co. The authors alone are responsible for the content and writing of this article.
References
- Agostoni C, Bresson J, Fairweather-Tait S. 2010. Scientific opinion on dietary reference values for water. EFSA J. 8:1459.
- Andersson R, Fransson G, Tietjen M, Åman P. 2009. Content and molecular-weight distribution of dietary fiber components in whole-grain rye flour and bread. J Agric Food Chem. 57:2004–2008.
- Brouns F, Brouns F, Bjorck I, Frayn K, Gibbs A, Lang V, Slama G, Wolever T. 2005. Glycaemic index methodology. Nutr Res Rev. 18:145.
- Canfora EE, Blaak EE. 2015. The role of polydextrose in body weight control and glucose regulation. Curr Opin Clin Nutr Metab Care. 18:395–400.
- Chaen H, Nishimoto T, Nakada T, Fukuda S, Kurimoto M, Tsujisaka Y. 2001. Enzymatic synthesis of kojioligosaccharides using kojibiose phosphorylase. J Biosci Bioeng. 92:177–182.
- Champ M, Langkilde A-M, Brouns F, Kettlitz B, Collet YLB. 2003. Advances in dietary fibre characterisation. 1. Definition of dietary fibre, physiological relevance, health benefits and analytical aspects. Nutr Res Rev. 16:71–82.
- Cheng Q, Dicosimo R, Ouwehand A, You Z, Suwannakham S, Nagy K, Payne M, Prasad J. 2015. Enzymatic synthesis of soluble glucan fiber. Patent: WO 2015183721 A1.
- Craig S, Holden J, Troup J, Auerbach M, Frier H. 1998. Polydextrose as soluble fiber: physiological and analytical aspects. Cereal Foods World. 43:370–376.
- Delzenne NM, Roberfroid M. 1994. Physiological effects of non-digestible oligosaccharides. LWT Food Sci Technol. 27:1–6.
- Eliasson A-C. 2006. Carbohydrates in food. CRC Press.
- European Commission. 2008. Commission directive 2008/100/EC of 28 October 2008 amending Council Directive 90/496/EEC on nutrition labelling for foodstuffs as regards recommended daily allowances, energy conversion factors and definitions. Off J Eur Union L248.
- Grysman A, Carlson T, Wolever T. 2008. Effects of sucromalt on postprandial responses in human subjects. Eur J Clin Nutr. 62:1364–1371.
- Howarth NC, Saltzman E, Roberts SB. 2001. Dietary fiber and weight regulation. Nutr Rev. 59:129–139.
- ICH. 1996. ICH harmonised tripartite guideline: guideline for good clinical practice E6 (R1). Proceedings of the International Conference on Harmonisation of Technical Requirements for Registration of Pharmaceuticals for Human Use; 1996; Washington, DC.
- Jie Z, Bang-Yao L, Ming-Jie X, Hai-Wei L, Zu-Kang Z, Ting-Song W, Craig SA. 2000. Studies on the effects of polydextrose intake on physiologic functions in Chinese people. Am J Clin Nutr. 72:1503–1509.
- Jones JM. 2013. Dietary fiber future directions: integrating new definitions and findings to inform nutrition research and communication. Adv Nutr. 4:8–15.
- Lahtinen SJ, Knoblock K, Drakoularakou A, Jacob M, Stowell J, Gibson GR, Ouwehand AC. 2010. Effect of molecule branching and glycosidic linkage on the degradation of polydextrose by gut microbiota. Biosci Biotechnol Biochem. 74:2016–2021.
- Lee B-H, Rose DR, Lin AH-M, Quezada-Calvillo R, Nichols BL, Hamaker BR. 2016. Contribution of the individual small intestinal α-glucosidases to digestion of unusual α-linked glycemic disaccharides. J Agric Food Chem. 64:6487–6494.
- Levene H. 1960. Robust tests for equality of variances. In: Olkin I et al., editors. Contributions to probability and statistics: essays in honor of Harold Hotelling. Vol. 2. Palo Alto, CA: Stanford University Press; p. 278–292.
- McCleary BV, De Vries JW, Rader JI, Cohen G, Prosky L, Mugford DC, Champ M, Okuma K. 2010. Determination of total dietary fiber (CODEX definition) by enzymatic-gravimetric method and liquid chromatography: collaborative study. J AOAC Int. 93:221–233.
- McCleary BV, Monaghan DA. 2002. Measurement of resistant starch. J AOAC Int. 85:665–675.
- McCleary BV, Sloane N, Draga A, Lazewska I. 2013. Measurement of total dietary fiber using AOAC Method 2009.01 (AACC International Approved Method 32-45.01): evaluation and updates. Cereal Chem. 90:396–414.
- Mäkeläinen H, Saarinen M, Stowell J, Rautonen N, Ouwehand AC. 2010. Xylo-oligosaccharides and lactitol promote the growth of Bifidobacterium lactis and Lactobacillus species in pure cultures. Benef Microb. 1:139–148.
- Mäkeläinen HS, Mäkivuokko HA, Salminen SJ, Rautonen NE, Ouwehand AC. 2007. The effects of polydextrose and xylitol on microbial community and activity in a 4-stage colon simulator. J Food Sci. 72:M153–M159.
- Mäkivuokko H, Nurmi J, Nurminen P, Stowell J, Rautonen N. 2005. In vitro effects on polydextrose by colonic bacteria and Caco-2 cell cyclooxygenase gene expression. Nutr Cancer. 52:94–104.
- Ouwehand AC, Tiihonen K, Saarinen M, Putaala H, Rautonen N. 2009. Influence of a combination of Lactobacillus acidophilus NCFM and lactitol on healthy elderly: intestinal and immune parameters. Br J Nutr. 101:367–375.
- Pettolino FA, Walsh C, Fincher GB, Bacic A. 2012. Determining the polysaccharide composition of plant cell walls. Nat Prot. 7:1590–1607.
- Raninen K, Lappi J, Mykkänen H, Poutanen K. 2011. Dietary fiber type reflects physiological functionality: comparison of grain fiber, inulin, and polydextrose. Nutr Rev. 69:9–21.
- Roberfroid MB. 1997. Health benefits of non-digestible oligosaccharides. In: Kritchevsky D, Bonfield C, editors. Dietary fiber in health and disease. Boston, MA: Springer; p. 211–219.
- Ryan T, Joiner B. 1976. Normal probability plots and tests for normality. Minitab Statistical Software: Technical Reports. The Pennsylvania State University, State College, PA. Available from: MINITAB Inc.
- Schulz KF, Altman DG, Moher D. 2010. CONSORT 2010 statement: updated guidelines for reporting parallel group randomised trials. BMC Med. 8:1.
- Slavin JL. 2005. Dietary fiber and body weight. Nutrition. 21:411–418.
- Snedecor GW, Cochran WG. 1967. Variance test for homogeneity of the binomial distribution. In: Statistical methods. 6th ed. Ames, IA: Iowa State University Press; p. 240–241.
- Tanabe K, Nakamura S, Oku T. 2014. Inaccuracy of AOAC method 2009.01 with amyloglucosidase for measuring non-digestible oligosaccharides and proposal for an improvement of the method. Food Chem. 151:539–546.
- Tanabe K, Nakamura S, Omagari K, Oku T. 2015. Determination trial of nondigestible oligosaccharide in processed foods by improved AOAC method 2009.01 using porcine small intestinal enzyme. J Agric Food Chem. 63:5747–5752.
- Tukey JW. 1949. Comparing individual means in the analysis of variance. Biometrics. 5:99–114.
- Wilson TA, Nicolosi RJ, Delaney B, Chadwell K, Moolchandani V, Kotyla T, Ponduru S, et al. 2004. Reduced and high molecular weight barley β-glucans decrease plasma total and non-HDL-cholesterol in hypercholesterolemic Syrian golden hamsters. J Nutr. 134:2617–2622.
- WMA. 2001. World Medical Association Declaration of Helsinki. Ethical principles for medical research involving human subjects. Bull WHO. 79:373.
- Yamamoto T, Unno T, Sugawara M, Goda T. 1999. Properties of a nigerose and nigerosylmaltooligosaccharides-supplemented syrup. J Appl Glycosci. 46:475–482.