Abstract
The role of the side chain of sterols and the sterol ring structure on the formation of ordered phases of the type observed in membrane rafts has been examined in aqueous dispersions of binary mixtures of sphingomyelin and androsterol. Comparisons have been made with binary systems of cholesterol, stigmasterol, β-sitosterol, and ergosterol with either sphingomyelin or dipalmitoylphosphatidylcholine. Thermotropic phase behaviour and structure of the mixed aqueous dispersions were characterized by differential scanning calorimetry, synchrotron X-ray diffraction, freeze-fracture electron microscopy, and Fourier-transform infrared spectroscopy. We show that: (i) Androsterol is less efficient in promoting the formation of liquid-ordered phase than other naturally occurring sterols which possess a side chain, (ii) cholesterol is the most efficient sterol of those investigated in forming liquid-ordered phase, (iii) the molecular stoichiometry of egg sphingomyelin and androsterol in the liquid-ordered phase is about 2:1, and (iv) sphingomyelin can form more stable liquid-ordered phase than glycerophospholipid in binary systems containing androsterol.
Abbreviations | ||
XRD | = | X-ray diffraction |
SAXS | = | small-angle X-ray scattering |
WAXS | = | wide-angle X-ray scattering |
DSC | = | differential scanning calorimetry |
FFEM | = | freeze-fracture electron microscopy |
FTIR | = | Fourier-transform infrared spectroscopy |
Lβ′ | = | lamellar-gel phase |
Pβ′ | = | ripple-gel phase |
Lα | = | liquid-crystal phase |
Lo | = | liquid-ordered phase |
SM | = | sphingomyelin |
Introduction
Cholesterol is an essential component of eukaryotic plasma membranes and is also found in lower concentrations in certain intracellular membranes in vesicular communication with the plasma membrane Citation[1–4]. One putative structural role for cholesterol is as a key and integral component of membrane microdomains such as caveolae and rafts. The incorporation of sterols into membranes induces a more ordered packing of the fluid acyl chains of phospholipids in the hydrophobic section of the bilayer, increases mechanical strength of the structure, and reduces their permeability to solutes Citation[5].
Cholesterol is the principal steroid of mammals and other homeothermic organisms; β-sitosterol and stigmasterol (phytosterols) have an ethyl group at C24, and stigmasterol contains an additional trans double bond between C22 and C23. Ergosterol (fungal sterol) has a methyl group at C24, and contains two more double bonds at C7 and C22, respectively. Androsterol is devoid of a sterol side chain at C17.
It has also been shown that both plant and fungal sterols can promote the formation of tightly packed lipid domains as is found with cholesterol Citation[1], Citation[6–9]. To better understand the essential structural features of sterols that are required to support mammalian cell growth, mutant strains of Chinese hamster ovary cells defective in sterol biosynthesis have been cultured with different sterols Citation[1]. It was found that sterols with minor modifications of the side chain such as campesterol, β-sitosterol, and desmosterol, supported long-term growth of the mutant cells, but sterols with more complex modifications of the side chain or the sterol nucleus itself (androsterol) did not. While androsterol has precisely the same structure and stereochemistry of the fused ring system as the parent cholesterol molecule except it lacks the C17 alkyl side chain. The above observations demonstrate the importance of the side chains of sterols in supporting cell growth.
Sphingolipids are components of the membranes of all living organisms and show a preference for partitioning into liquid-ordered (Lo) states in biological membranes. Model membrane studies have shown that the molecular characteristics of sphingolipids, particularly the long saturated N-acyl chains and the rigid structure at amide group are important in mediating this behaviour Citation[2], Citation[10–15]. Sphingomyelin (SM) and glycerophosphorylcholine (PC) both contain a phosphocholine polar group, but the backbone of SM is sphingosine, while the backbone of PC is glycerol. The difference in tendency to form Lo phases resides in the way sterols interact with the respective backbone of these lipids.
Saturated molecular species of phosphatidylcholines, such as the dipalmitoyl derivative of phosphatidylcholine (DPPC), are known to have similar physical properties to that of sphingolipids Citation[3], Citation[11]. So DPPC/sterol mixtures have been selected by many research groups as simple models of biomembrane microdomains or the basic structure of lipid rafts. To understand the conditions required to form membrane rafts, a number of phase diagrams of binary systems have been reported. For example, a partial phase diagram of DPPC/cholesterol has been constructed by Vist and Davis Citation[16] and has received additional experimental and theoretical support Citation[17–19]. Hsueh's group and our group have constructed partial phase diagrams of DPPC/ergosterol, DPPC/stigmasterol and DPPC/androsterol, respectively Citation[20–22].
One aim of the present work is to describe the phase behaviour of egg sphingomyelin/androsterol binary system and to compare their properties with other naturally-occurring sphingomyelin/sterol binary systems and to compare these with published phase diagrams. The characterization of these mixtures has been performed using differential scanning calorimetry (DSC), synchrotron X-ray diffraction (XRD), freeze-fracture electron microscopy (FFEM), and Fourier-transform infrared spectroscopy (FTIR) techniques. We have determined phase transition enthalpies and thermotropic changes in the structure of these mixed dispersions as a function of temperature and provide a detailed picture of the role of sterol structure on interaction with the polar lipids.
Materials and methods
Materials
Chicken egg-yolk sphingomyelin (SM) and glucocerebrosides (GlcCer) were purchased from Sigma Chemicals (St Louis, MO, USA); the egg SM is highly enriched in long-chain saturated fatty acids, particularly in 16:0 (82.6%), and contains small amounts of 18:0 (7.4%) Citation[23]. In DSC and FTIR experiments, the egg SM used is purchased from Avanti Polar Lipids, Inc. USA. The chains of this egg SM are all saturated and are rich in 16:0 (84%), and contain small amounts of 18:0 (6%), 20:0 (2%), 22:0 (4%), and 24:0 (4%). Cholesterol and androsterol were obtained from Steraloids Inc (Rhode Island, USA). Ergosterol, stigmasterol, and β-sitosterol were purchased from MP Biomedicals, Inc. (Germany). SM/sterol mixtures with designated mole ratios were dissolved in chloroform, dried under a stream of oxygen-free dry nitrogen, and stored in vacuum overnight to remove any remaining traces of solvent. The lipid films were hydrated with Tris-HCl buffer consisting of 50 mM Tris-HCl, 150 mM NaCl, and 0.1 mM CaCl2 (pH = 7.2). The lipid to solvent ratio is 1/4 (by wt.). The dispersions were mixed with repeated vortex and thermal cycling between 65°C and -20°C to ensure homogeneous dispersion. Samples with 2.5, 5, 7.5, 10, 15, 20, 25, 30, 35, and 40 mole% androsterol were prepared for examination.
In order to compare the structural effect of the sterol side chain substituent on phase behaviour of sphingomyelin bilayers, binary mixtures of cholesterol, stigmasterol, β-sitosterol, and ergosterol in proportions of 20 and 33 mole% in sphingomyelin have been examined by DSC and FTIR. The samples for DSC experiments were prepared as described above and for FTIR experiments, the pure SM and mixed lipid samples of 20 and 33mole% sterol in sphingomyelin were hydrated with D2O in order to perform a precise image analysis.
Experimental methods
X-ray diffraction (XRD) experiments. Static synchrotron X-ray diffraction experiments were performed at Station BL40B2 of SPring-8, Japan. The XRD data were recorded with an image plate detector. The wavelength was 0.10 nm and camera length was 400 mm. X-ray scattering intensity patterns were recorded during 30 s exposure of the sample to the synchrotron beam. A standard silver behenate sample was used for calibration of diffraction spacings. Each sample was sealed in a copper sample holder with thin mica windows about 1 mm apart. The sample holder, with a volume of about 20 µl, was mounted on a programmable Linkam thermal stage (Linkam Scientific Instruments, UK,±0.1°C). Samples were heated from 30–51°C at 0.55°C/min. X-ray powder diffraction intensity data were analyzed and integrated by a program Fit2D.
Electron density distributions across the unit cell of the lamellar repeat of SM or its mixtures with androsterol dispersed in water were calculated as follows. Integrated intensities I(h) for a range of diffraction orders (h) were obtained from small-angle scattering intensity profiles. Electron density profiles in a one-dimension space x, in arbitrary unit, were calculated from Fourier reconstructions using the X-ray structure factors Citation[24], Citation[25]1 where F(h) is the structure factor and equates to the root of [h2I(h)], g(h) is the phase of F(h) of the hth order diffraction, and d is the repeat spacing of the multibilayers.
To calculate a true electron density profile, it is first necessary to assign phase angles to each of the diffraction orders. One way to solve this problem is to adopt a model-building approach. In the camera configuration used in this study, five orders of diffraction were collected from the dispersions of SM containing different fractions of androsterol. If we assume that the multilamellar structures of the dispersions are centrosymmetric, the phase angles of the structure factor F(h) are restricted to either π or 0. This implies that g could only be −1 or 1 Citation[26], Citation[27].
Thus there are 32 combinations of phases for one dispersion sample in electron density analysis. Examination of all possible phase sets indicates that the only plausible combination of phases consistent with a bilayer repeat is (–+–), which is in agreement with previous results of egg sphingomyelin systems Citation[28].
Differential scanning calorimetry (DSC)
Calorimetry was performed using a Mettler-Toledo DSC821e differential scanning calorimeter with a high-sensitivity sensor (HSS7). Samples (20 µl) were examined using a scan rate of 0.5°C/min and at least three scans were performed to verify reproducibility.
Freeze-fracture electron microscopy (FFEM)
Samples and tools used for sample manipulation were equilibrated at the desired temperature for at least 30 min. A small drop of the sample dispersion was placed on Au plates and manually plunged into liquid nitrogen. The freeze-fracture procedure was carried out in a Balzers BAF 400D freeze-fracture apparatus. Fracture surfaces were shadowed with platinum/carbon. Platinum and carbon evaporation was done from 45° and 90° angles, respectively. Replicas were floated on a solvent of methanol-chloroform (1:3, by vol.). Images of the replicas were recorded with a JEOL/JEM-1200EX transmission electron microscope.
Fourier-transform infrared spectroscopy (FTIR)
FTIR spectra were recorded using a Nicolet 5700 spectrometer. Samples were coated onto the inner surfaces of a pair of CaF2 windows, which were mounted on a temperature-controlled sample holder. The lipid dispersions were sealed with silicone grease between the two CaF2 windows to prevent evaporation of the water. Samples were heated from 20–70°C at 0.5°/min. Spectra were recorded in the range of 4000–900 cm−1, with a resolution of 2 cm−1.
A spectral image analysis method has been employed to evaluate spectral changes over a range of wavenumbers Citation[22], Citation[29]. For two data sets X and Y such as two FTIR spectra, a parameter, correlation coefficient C(X, Y), is expressed with the following equation:2 where SXY is the covariance between X and Y, SXX and SYY are the covariances of X and Y themselves. The correlation coefficient reflects the similarity between two selected spectra. It is zero if X and Y are statistically independent and is 1 when X and Y are the most significantly correlated. The parameter can remove system errors caused by the inconsistencies of the Fourier transform and solvent suppression Citation[29–31].
In this work, the spectrum parameter correlation coefficient, C, has been evaluated by comparing each spectrum with the first spectrum. The spectral region used for this analysis is 2800–3000 cm−1, which covers the characteristic bands of the symmetrical and asymmetrical stretching vibrations of CH2 and CH3. The parameter could be used to identify phase transitions triggered by either temperature or concentration.
Results and discussion
Thermal analysis of SM/androsterol systems
DSC thermograms of egg sphingomyelin dispersions, with and without different sterols, recorded during a heating scan at 0.5°C/min between 10 and 65°C are presented in . In the absence of the sterol, SM dispersions display a relatively sharp endothermic transition at 39.5°C, which is designated as the peak temperature of the gel to Lα main transition according to McMullen and McElhaney Citation[32]. The phase transition temperature is in good agreement with published data Citation[33–35].
Figure 1. DSC thermograms of mixtures of egg SM dispersions containing different sterols with 5:1 (solid line) and 2:1 (dotted line) SM/sterol molar ratios. Samples equilibrated at 10°C were heated to 65°C at a scanning rate of 0.5°C/min−1.
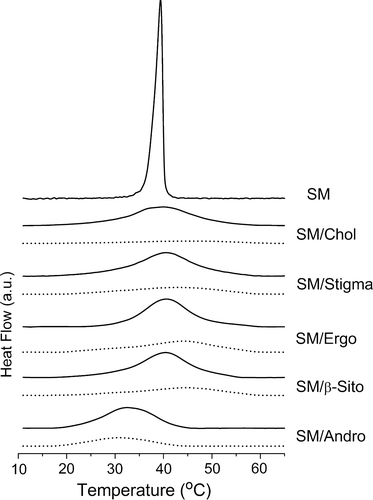
In general, the endothermic peak of the main phase transition of the phospholipid is broadened by the presence of sterols. It can be seen from that all five sterols, cholesterol, ergosterol, stigmasterol, β-sitosterol, and androsterol broaden the endothermic peak of the main phase transition of SM and this effect is directly related to the proportion of sterol in the mixture. The broad peak in mixtures of 17 mole% sterol in SM, as previously described, can be resolved into two components, representing the melting of sterol-poor and sterol-rich domains, respectively Citation[22], Citation[28], Citation[36]. By contrast, the thermograms of the samples containing 33 mole% sterols are broader, of lower enthalpy, and probably originate from transition of a single phase.
Sterols that possess a hydrocarbon chain substituent (cholesterol, stigmasterol, β-sitosterol, and ergosterol) all induce a shift of the endothermic peak of the main transition of SM to higher temperatures. This effect can be seen from a comparison of phase transition temperatures (peak temperature) and transition enthalpies of the different sterols dispersed with SM which are presented in . The data show that in mixtures containing a higher proportion of sterol (33 mole%) the phase transition temperature is higher than that recorded in mixtures with lower proportions of sterol. This infers that at temperatures below the phase transition the phospholipids are in a phase state that is more stable and ordered resulting in a shift in the melting temperature of hydrocarbon chains to higher temperatures.
Table I. Temperatures (peak temperature) and phase transition enthalpies of SM and SM/sterol dispersions.
It is interesting to note that the enthalpy of SM dispersion with 33 mole% cholesterol is the smallest of all the binary systems of sterols codispersed with SM that were examined. Similar findings have been observed for binary mixtures of sterols with DPPC Citation[36]. This is in agreement with results published by McElhaney's group, who showed that increasing proportions of cholesterol cause a progressive broadening of the gel to liquid crystal phase transition and a decrease in the enthalpy change of transition Citation[28]. It suggests that the binary system of SM and cholesterol above molar ratio of 2:1 reaches a stable phase.
In conspicuous contrast to the effect of sterols possessing hydrocarbon side chains androsterol induces a shift of the main transition temperature of SM to lower temperatures. The extent of this reduction in temperature is directly related to the proportion of androsterol in the mixture.
To further investigate the effect of androsterol on the thermotropic phase behaviour of SM, a systematic study of a range of sterol mixtures was undertaken. DSC thermograms of the codispersions are presented in A. The thermal data clearly show the relationship between broadening of the endothermic peak and reduction in phase transition temperature and the proportion of androsterol in the binary mixture. These effects of androsterol on the peak temperature and onset temperature of the DSC heating endotherms are summarized in B. The effect of androsterol on the thermotropic behaviour of SM is contrary to that of natural or enantiomeric cholesterol Citation[28], which causes a progressive upshift of main transition temperature proportional to the cholesterol concentration. These results imply that the side chain of sterol plays an important role in preserving the degree of order of the surrounding lipid chains and for maintenance of cohesive forces between them. It may be noted from mixtures containing less than 10 mole% androsterol that the endothermic peaks are asymmetric so as to indicate a preferential interaction of the sterol with the lower melting-point molecular species (N-palmitoyl sphingomyelin) of egg SM. Additionally, the significant decrease of main transition temperature in SM/androsterol indicated that there is no interdigitated bilayer phase in the system. And the lower transition enthalpies suggest that long hydrocarbon chains could prefer adding more gauche conformation isomer to occupy the surrounding space resulting from hydrophobic mismatch between the androsterol molecule and the host phospholipid bilayer. Furthermore, the relatively broad transition endotherm typical of sphingomyelins extracted from biological material (egg, brain, milk) showed no sign of a pretransition endotherm even at scan rates as low as 0.5°C/min.
Figure 2. (A) DSC thermograms of SM/androsterol mixtures containing indicated molar percentages of androsterol at a scanning rate of 0.5°C/min; (B) plots of the peak (•) and onset (▴) temperatures of the DSC endotherms of SM/androsterol as a function of sterol concentration. Mean±SEM are shown for three replicates.
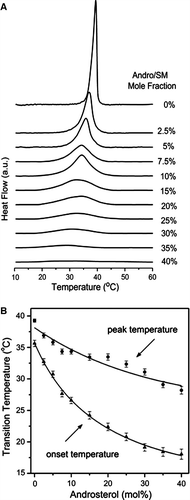
Structural analysis of SM/androsterol mixtures
Lamellar structure determination
X-ray diffraction patterns were recorded over the temperature range from 30–51°C at a heating rate 0.5°C/min. At least five orders of diffraction have been recorded in the small-angle region for each of the mixed lipid dispersions. The reciprocal spacings of the first five diffraction orders show a ratio of 1:2:3:4:5, showing that all the dispersions are multilamellar. Representative small-angle (SAXS) and wide-angle X-ray scattering (WAXS) intensity patterns recorded from a codispersion of egg SM with 20 mol% androsterol over the temperature range from 30–51°C are presented in A. The unaltered peak at S = 0.63 nm−1 in the scattering intensity profiles originates from the mylar window of the vacuum tube.
Figure 3. (A) Small-angle (SAXS) and wide-angle (WAXS) scattering intensity patterns recorded from a binary mixture of SM containing 20 mol% androsterol during a heating scan at 0.55°C/min. The temperature interval of the recorded diffraction patterns was 3°C. Electron micrograph of freeze-fracture replicas showing multilamellar structures of (B) egg SM, quenched from 31°C and (C) egg SM containing 20 mol% androsterol, quenched from 35°C. Bar = 200 nm.
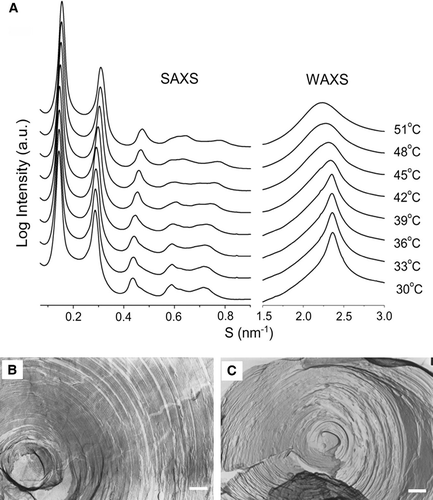
Detailed analysis of the data revealed that the spacing of the first-order SAXS pattern decreased from about 6.98 nm at 30oC to about 6.46 nm at 51°C. Conversely, the d-spacing of the WAXS intensity patterns increased from about 0.422 nm at 30°C to about 0.465 nm at 51°C. Compared with DSC results and previous XRD information of other sterols with phospholipids, the diffuse scattering band centered around 0.46–0.47 nm in wide angle pattern implied the lamellar structure with fluid hydrocarbon chains typical of a liquid-crystal phase (Lα) Citation[21], Citation[25]. The sharp diffraction in the WAXS pattern at 0.422 nm at low temperature indicated that lipids are in compact and ordered packing arrangement of temperatures around the onset of the main endothermic transition recorded by calorimetry.
Freeze-fracture electron microscopy provides a direct method of verifying the structures of the lipid dispersions. All codispersions of SM and androsterol showed lamellar structures and two representative electron micrographs are presented in B and 3C. The replicas show multilamellar dispersions of egg SM quenched from 31°C (B) and SM codispersed with 20 mol% androsterol, quenched from 35°C (C).
The properties of phase states in SM/androsterol binary mixtures
Besides pretransition in DSC endotherms, there are several ways to identify a ripple phase: (i) The lamellar periodicity of ripple phase is invariably greatest amongst Lβ, Pβ, and Lα phases Citation[37–39]; (ii) compared with the SAXS diffraction patterns of lamellar-gel phase, the shape of the Bragg peaks are broader Citation[21]; (iii) The (01) diffraction pattern of the undulation structure can be observed at a smaller angle than the first-order of the lamellar repeat with a reciprocal spacing approaching 0.05 nm−1 Citation[40], Citation[41]; and (iv) FFEM is a convincing way to visualize the ripple phase Citation[42], Citation[43].
In SM/androsterol binary mixtures, pretransition was not detected with DSC in this work. Previous DSC studies have shown that although the pretransition of DPPC or DMPC was abolished by the presence of more than 6 mol% cholesterol Citation[16], Citation[44], the ripple phase was clearly evident in dispersions containing 15 mol% Citation[45] or 16 mol% Citation[46] cholesterol when examined using freeze-fracture electron microscopy. We have not been able to detect a pretransition endotherm by DSC in binary mixtures of SM/androsterol even in mixtures containing as little as 2.5 mole% of androsterol.
To characterize the ripple structure of egg SM codispersed with androsterol we have used SAXS and FFEM. SAXS intensity patterns of binary mixtures of egg SM containing 10 mole% androsterol (A) show bilayer structures with a lamellar d-spacing of about 9–11 nm and broad Bragg peaks that indicate disorder in the structure consistent with ripple structure. These data suggest that ripple structure is present in binary mixtures of egg SM containing 10 mole% androsterol. The evidence of ripple structures in lipid mixtures containing higher proportion of androsterol is provided by FFEM.
Figure 4. (A) SAXS/WAXS intensity patterns recorded at 30°C, 33°C, and 36°C from dispersions of egg SM containing 10 mole% and 15 mole% androsterol. Electron micrographs of freeze-fracture replicas prepared from (B) pure egg SM thermally quenched from 31°C and (C) a dispersion of a binary mixture of egg SM containing 20 mole% androsterol thermally quenched from 35°C. Bar = 100 nm.
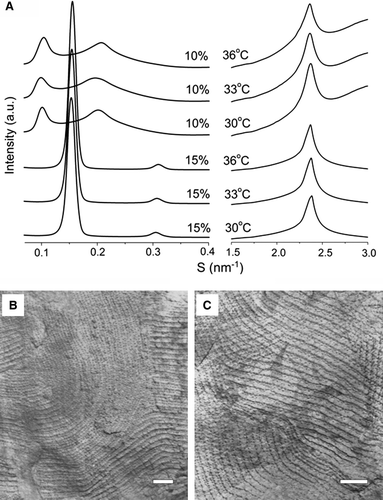
Freeze-fracture replicas were prepared from the dispersions of pure SM and SM/androsterol mixtures and examined by electron microscopy. Representative images of freeze-fracture replicas are presented in B and 4C. These show well-characterized ripple structure in both dispersions of pure sphingomyelin thermally quenched from 31°C (B), and codispersions of egg sphingomyelin containing 20 mole% androsterol thermally quenched from 35°C (C). The ripple periodicity was found to be 25.5 nm in pure egg sphingomyelin and slightly larger when the dispersion contained androsterol (27.9 nm). The type of ripple structure is identical to that reported for brain sphingomyelin which is characterized by an asymmetric ripple periodicity Citation[43]. Despite the regular appearance of the ripple structure revealed in freeze-fracture replicas there was no evidence of Bragg reflections in the SAXS patterns that correspond to the observed periodicities.
In general, it has been found that the temperature of the pretransition from lamellar-gel phase to ripple gel phase decreases with increasing sterol concentration Citation[21], Citation[22]. As a result, the boundary of lamellar-gel phase to ripple-gel phase will be below 31°C in the phase diagram of SM/androsterol. Therefore, the phase transition detected by DSC ( and A) is the main transition from ripple gel phase to Lα. An asymmetric ripple gel phase characterizing Pβ′ in mixtures of brain SM/cholesterol or C24-SM/cholesterol bilayers has been reported Citation[43], Citation[47], Citation[48] and the ripple-gel phase of pure egg SM assigned as Pβ′ structure. The asymmetric WAXS intensity patterns recorded from mixtures of egg SM/androsterol also support the assignment of the phase as Pβ′.
With increasing concentration of sterol, the DSC thermograms become broader and the enthalpy is reduced. To study the structural features of these multilamellar dispersions the periodicity (d-spacing) of SM with 20, 30, and 40 mol% androsterol was examined by synchrotron X-ray diffraction at temperatures between 30 and 52°C. The d-spacings are plotted as a function of temperature in A. The mixture of SM with 20 mol% androsterol undergoes a marked decrease in lamellar d-spacing with increasing temperature (from 6.98–6.46 nm). When the proportion of androsterol is increased to 30 mol% or more the temperature dependence of the lamellar periodicity decreases significantly. The decrease in d-spacing is from 6.75–6.64 nm for 30 mol% and 6.68–6.53 nm for 40 mol% androsterol in SM over the temperature range examined. These data suggest that the structures of the lipid mixtures containing 30 mol% or more androsterol are relatively stable and the change in observed d-spacing could arise mainly from trans and gauche conformational transitions in long hydrocarbon chain of sphingomyelin during heating.
Figure 5. (A) Lamellar d-spacing of dispersions of egg SM containing 20, 30, and 40 mole% androsterol plotted as a function of temperature; (B) electron density profiles for 2:1 SM:androsterol and 2:1:0.3 SM:androsterol:glucosylceramide in excess water at 36°C; (C) the WAXS patterns of egg SM with 30 and 35 mole% androsterol, respectively; (D) the relationship between crystal peaks observed in the WAXS region in 35 mol% SM/androsterol and pure androsterol.
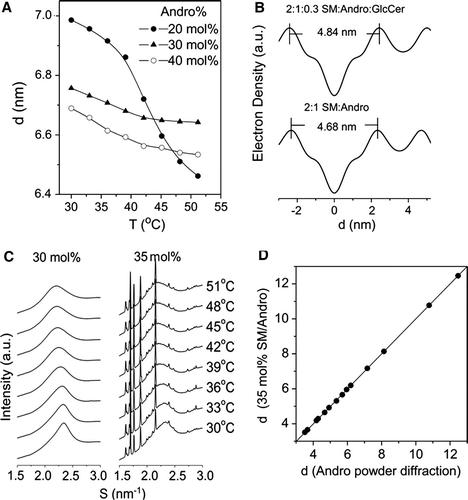
The WAXS patterns of the Lo phase formed from egg SM and sterols have been characterized recently and a WAXS peak in a codispersion of around 0.43 nm was reported for SM containing 33 mole% cholesterol Citation[49]. In the present study a codispersion of SM with 30 mol% androsterol gives a scattering maximum in the WAXS region of 0.433 nm at 36°C. This implies that androsterol has a condensing effect on the SM multibilayer structures. The results of Langmuir isotherm analysis and detergent solubility by Triton X-100 show that androsterol can moderately lower the lateral elasticity of SM and enhance the detergent resistance of SM in SM/androsterol mixtures, although this tendency is weaker than with cholesterol Citation[50]. Accordingly, the phase of SM with over 30 mol% androsterol could be assigned as liquid-ordered phase, Lo.
To further characterise the Lo phase in this system, electron density profiles were calculated to compare the bilayer thickness of SM with different sterols. The bilayer thickness, dp − p, of the mixture of SM with 30 mol% androsterol (about 2:1 SM:androsterol) shown in B is 4.68 nm. This is similar to the reported thickness of a SM/cholesterol mixture (dp − p=4.8 nm) Citation[51].
The lipid raft model of Simons and Ikonen proposed that microdomains of membrane rafts are rich in sphingolipids (both sphingomyelin and glycosphingolipids) and cholesterol Citation[52–54]. Therefore, a ternary mixture of 2:1:0.3 SM:androsterol:glucosylceramide was examined for comparison with the binary mixture of 2:1 SM:androsterol in B. The bilayer thickness, dp − p, is 4.84 nm in this codispersion which is a little greater than that of binary mixtures of 2:1 SM:androsterol and 2:1 SM:cholesterol possibly because of the long hydrocarbon chains in GlcCer. In addition, the repeat spacing of the scattering maxima in the WAXS region of the ternary mixture is 0.428 nm similar to that of 2:1 SM:androsterol (0.433 nm) at 36°C, and both systems show a coincident spacing of 0.457 nm at 51°C. Therefore, the systems of 2:1 SM:androsterol and 2:1:0.3 SM:androsterol:GlcCer have similar structural characteristics and form condensed phase states.
Interestingly, a saturated effect was observed in synchrotron XRD patterns. The WAXS patterns of dispersions of SM with 30 and 35 mole% androsterol are shown in C. The mixture of SM with 30 mole% androsterol had a typical hydrocarbon WAXS pattern, while a number of sharp diffraction peaks can be seen in 35 mol% androsterol mixture. The set of these crystal peaks and the powder diffraction recorded from pure androsterol are compared in D. The sharp WAXS peaks observed in the codispersion of SM and 35 mole% androsterol clearly arise from identical crystalline androsterol. This suggests that proportions of androsterol between 30 mole% and 35 mole% form the Lo phase and excess androsterol separates out as crystals, indicating a stoichiometry of 2:1 SM:androsterol. The saturation effect is also detected in codispersions of SM and stigmasterol in which proportions of sterol of 35 mol% or more induced a set of sterol crystal diffraction peaks in WAXS patterns (data not shown).
In order to compare the properties of androsterol with other phospholipid/sterol systems a partial phase diagram of the SM/androsterol mixtures in excess water has been constructed and is shown in . The boundaries of the diagram have been defined on the basis of evidence obtained from the different methods used to study the binary mixtures.
Based on the shape and reciprocal spacing of XRD patterns in A, there is an abrupt change between 10–15 mol% sterol in the temperature range from 30–36°C, therefore an average value of 12.5 mol% androsterol was taken to delineate the Pβ′/(Pβ′+Lo) boundary.
From the information in C a saturation concentration of androsterol in forming Lo phase is seen at between 30–35 mol% sterol in the temperature range 30–51°C, therefore the right side boundaries of Pβ′+Lo and Lα+Lo were delineated with a concentration of 32.5 mol% androsterol. With higher proportions of androsterol Lo phase coexists with androsterol crystals.
The boundary of Pβ′/Lα is defined by the peak temperature of the main transition endotherms in DSC thermograms of SM and SM with 2.5, 5, 7.5, and 10 mol% androsterol mixtures.
The boundary of Lα/Lα+Lo is determined by the endpoint of the main transitions in DSC thermograms of SM with 15, 20, 25, and 30 mol% androsterol mixtures.
The three-phase line was determined by the point of intersection of the boundaries of Pβ′/Lα, Lα/Lα+Lo, and Pβ′/Pβ′+Lo. The ripple phase detected by FFEM in 20 mol% SM/androsterol at 35°C also supports the rationality of the three-phase line. The phase diagram of SM/androsterol may be contrasted with that of DPPC/androsterol Citation[22] because saturation with sterol is not observed in codispersions of the sterol with DPPC in the region of 35 mole% androsterol and Lo is present over a wide range of androsterol concentrations.
Figure 6. A partial phase diagram sketch of egg SM/androsterol system in excess water. See text for details.
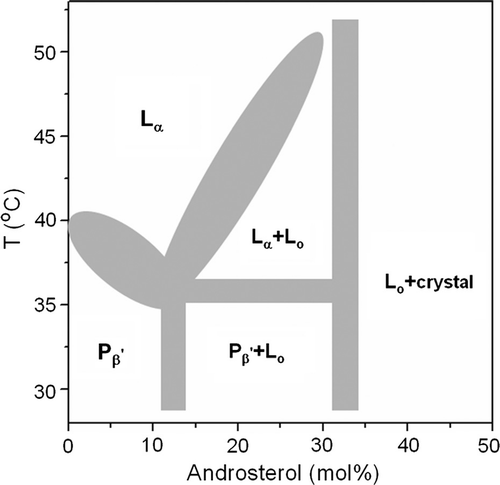
It has been pointed out that lipid physicochemical properties are the driving forces governing the creation of membrane domains and the organization of molecules within them Citation[15]. The relatively stable molecular stoichiometry of Lo phase formed by SM and androsterol could result from a particular interaction between the two lipids. The relevant structural features of sphingomyelin are: (i) Functional groups amine NH, hydroxyl OH, and carbonyl C = O in the interface as hydrogen bond donor and acceptor with themselves or with sterols; and (ii) the rigid ceramide moiety structure. By contrast, diacylglycerophosphatides like phosphatidylcholine can act only as H-bond acceptor Citation[55]. Many studies have indicated that there is a stronger interaction in SM/sterol than PC/sterol. For instance, cholesterol condenses sphingomyelin monolayers to a greater extent than monolayers of other phospholipids; the rate of cholesterol desorption is much smaller from sphingomyelin bilayers than from other types of phospholipid bilayers; water permeability of sphingomyelin/cholesterol bilayers is lower than that of other lipid bilayers Citation[47], Citation[50], Citation[56]. Androsterol has the functional group OH and has precisely the same structure and stereochemistry of the rigid planar fused ring system as cholesterol, so it could exercise constraints on the motion of hydrophobic tails of adjacent phospholipids and promote the formation of Lo phase, although less efficiently than cholesterol because it lacks a hydrocarbon side chain. In addition, the condensed phase state of SM/sterol is more stable than that in DPPC/sterol Citation[36], Citation[57] mixtures as evidenced by the WAXS pattern and the temperature dependence of reciprocal spacings in SAXS region, especially at high temperatures. It is likely that the hydrogen bond donor and acceptor groups of sphingolipids enhance lateral interactions and stability of the membrane microdomains.
Comparison with other SM/sterol systems
Compared with phase diagrams of binary systems, SM/cholesterol Citation[58], DPPC/cholesterol Citation[16], DPPC/ergosterol Citation[20], DPPC/stigmasterol Citation[21], and DPPC/androsterol Citation[22], the mixtures of 5:1 SM (or DPPC)/sterol should be comprised of two components, sterol-poor and sterol-rich phase, respectively. When the proportion of sterol approaches 30 mole%, a liquid-ordered phase predominates the phase structure. This is also reflected in endothermic peaks of the main transition of 5:1 SM/sterol and 2:1 SM/sterol mixtures shown in . The thermograms of 5:1 SM/sterol have great enthalpies and broad transition peaks, which should be comprised of two components, the melting of sterol-poor and sterol-rich domains. By contrast, the thermograms of 2:1 SM/sterol are broader and have lower enthalpies.
To better understand the structural effect of the sterol side chain on the phase behaviour of sphingomyelin, image analysis was conducted through FTIR spectra Citation[22]. FTIR spectra were recorded over the temperature range 20–70°C with a heating rate of 0.5°C/min. A wave number range from 2800–3000 cm−1 has been selected to perform image analysis. Correlation coefficients of SM and 5:1 and 2:1 SM/sterol mixtures are thus evaluated from each spectrum and the first spectrum on heating.
With increasing temperature, the correlation coefficient curve of pure SM undergoes an abrupt drop near the main transition temperature about 40°C. Above and below the transition temperature, the SM system shows two relatively linear regions assigned as stable gel and Lα phase, respectively, which is in agreement with calorimetric results. In the 5:1 SM/sterol mixtures, the obvious phase transition is preserved in the SM/sterol mixtures; cholesterol gives the smallest change in correlation coefficient curves in A. Depending on the extent of change in the correlation coefficient with temperature, the order of natural sterols on their ability to modulate the phase behaviour of sterol/SM mixtures is: cholesterol > phytosterol (sitosterol and stigmasterol) > fungal sterol (ergosterol).
With the higher proportion of sterols (B) the changes in correlation coefficients are smaller over the temperature range and they show an almost linear trend. This indicates that 2:1 SM/sterol mixtures could have formed stable phase (Lo phase), which is relatively independent of temperature and distinct from a 2:1 DPPC/sterol system Citation[36]. This can be explained by the difference in molecular structure between SM and DPPC and in particular the ability of SM/sterol mixtures to form an extended hydrogen-bond network. Based on the extent of change in correlation coefficient curves, the natural sterols on their ability to stabilize the Lo phase of 2:1 SM/sterol mixtures are still in the order: cholesterol > phytosterol > fungal sterol.
The SM/androsterol systems are clearly different from all the SM mixtures with sterols possessing a side chain that are presented in . Thus the onset of the phase transition occurs at a much lower temperature, in agreement with the DSC results observed in , which shows that addition of androsterol to SM reduces the transition temperature.
Conclusions
Phase behaviour of binary systems of sphingomyelin and sterols have been examined and compared. Different sterol molecular structures influence the phase transition behaviour of SM in different ways. We can draw several conclusions from the present study.
There is a saturation effect in incorporation of androsterol into SM bilayers which suggests that the molecular stoichiometry of SM and androsterol in Lo phase is 2:1.
SM can form more stable Lo phase than glycerophospholipid in binary systems containing androsterol.
Sterols with a hydrocarbon chain substituent at C17 limit the motion of the adjacent lipid chains more effectively and form more stable phases than sterol lacking a side chain (androsterol).
Cholesterol forms more stable associations with SM than phytosterol (β-sitosterol and stigmasterol) or ergosterol thus the order in stabilizing Lo phase is: cholesterol > sitosterol > stigmasterol > ergosterol > androsterol.
Acknowledgements
This work was supported by grants from the Natural Science Foundation of China (NSFC: 20633080 & 20675046) and the Human Frontier Science Program (RGP0016/2005C). Assistance of Dr Takahashi from Gunma University and Dr Inoue from SPring-8 in the synchrotron station facility setup is gratefully acknowledged. Declaration of interest: The authors report no conflicts of interest. The authors alone are responsible for the content and writing of the paper.
References
- Xu F, Rychnovsky SD, Belani JD, Hobbs HH, Cohen JC, Rawson RB. Dual roles for cholesterol in mammalian cells. Proc Natl Acad Sci USA 2005; 102: 14551–14556
- Simons K, Ikonen E. Cell biology – how cells handle cholesterol. Science 2000; 290: 1721–1726
- Ohvo-Rekilä H, Ramstedt B, Leppimaki P, Slotte JP. Cholesterol interactions with phospholipids in membranes. Prog Lipid Res 2002; 41: 66–97
- Liscum L, Munn NJ. Intracellular cholesterol transport. Biochim Biophys Acta 1999; 1438: 19–37
- Bloch K E. Sterol structure and membrane function. CRC Crit Rev Biochem 1983; 14: 47–92
- Peskan T, Westermann M, Oelmuller R. Identification of low-density Triton X-100-insoluble plasma membrane microdomains in higher plants. Eur J Biochem 2000; 267: 6989–6995
- Mongrand S, Morel J, Laroche J, Claverol S, Carde JP, Hartmann MA, Bonneu M, Simon-Plas F, Lessire R, Bessoule JJ. Lipid rafts in higher plant cells: Purification and characterization of Triton X-100-insoluble microdomains from tobacco plasma membrane. J Biol Chem 2004; 279: 36277–36286
- Borner GHH, Sherrier DJ, Weimar T, Michaelson LV, Hawkins ND, MacAskill A, Napier JA, Beale MH, Lilley KS, Dupree P. Analysis of detergent-resistant membranes in Arabidopsis. Evidence for plasma membrane lipid rafts. Plant Physiol 2005; 137: 104–116
- Martin SW, Glover BJ, Davies JM. Lipid microdomains-plant membranes get organized. Trends Plant Sci 2005; 10: 263–265
- Brown DA, London E. Functions of lipid rafts in biological membranes. Annu Rev Cell Dev Biol 1998; 14: 111–136
- Stottrup BL, Keller SL. Phase behaviour of lipid monolayers containing DPPC and cholesterol analogs. Biophys J 2006; 90: 3176–3183
- Wang TY, Silvius JR. Sphingolipid partitioning into ordered domains in cholesterol-free and cholesterol-containing lipid bilayers. Biophys J 2003; 84: 367–378
- Hakomori S, Handa K, Iwabuchi K, Yamamura S, Prinetti A. New insights in glycosphingolipid function: “glycosignaling domain,” a cell surface assembly of glycosphingolipids with signal transducer molecules, involved in cell adhesion coupled with signaling. Glycobiology 1998; 8: ix–xviii
- Pascher I. Conformation and hydrogen bonding of ceramide and their implication on membrane stability and permeability. Biochim Biophys Acta 1976; 455: 433–451
- Sonnino S, Prinetti A, Mauri L, Chigorno V, Tettamanti G. Dynamic and structural properties of sphingolipids as driving forces for the formation of membrane domains. Chem Rev 2006; 106: 2111–2125
- Vist MR, Davis JH. Phase equilibria of cholesterol/dipalmitoylphosphatidylcholine mixtures: 2H nuclear magnetic resonance and differential scanning calorimetry. Biochemistry 1990; 29: 451–464
- Linseisen FM, Thewalt JL, Bloom M, Bayerl TM. Deuterium-NMR and DSC study of SEPC-cholesterol mixtures. Chem Phys Lipids 1993; 65: 141–149
- Murtola T, Falck E, Patra M, Karttunen M, Vattulainen I. Coarse-grained model for phospholipid/cholesterol bilayers. J Chem Phys 2004; 121: 9156–9165
- Ipsen JH, Karlstroem G, Mouritsen OG, Wennerstroem H, Zuckermann MJ. Phase equilibria in the phosphatidylcholine-cholesterol system. Biochim Biophys Acta 1987; 905: 162–172
- Hsueh YW, Gilbert K, Trandum C, Zuchermann M, Thewalt J. The effect of ergosterol on dipalmitoylphosphatidylcholine bilayers: A deuterium NMR and calorimetric study. Biophys J 2005; 88: 1799–1808
- Wu RG, Chen L, Yu ZW, Quinn PJ. Phase diagram of stigmasterol-dipalmitoylphosphatidylcholine mixtures dispersed in excess water. Biochim Biophys Acta 2006; 1758: 764–771
- Gao WY, Wu RG, Yu ZW, Quinn PJ. Phase diagram of androsterol-dipalmitoylphosphatidylcholine mixtures dispersed in excess water. J Phys Chem B 2008; 112: 8375–8382
- Hsu FF, Turk J. Structural determination of sphingomyelin by tandem mass spectrometry with electrospray ionization. J Am Soc Mass Spectrom 2000; 11: 437–449
- McIntosh TJ, Magid AD, Simon SA. Cholesterol modifies the short-range repulsive interactions between phosphatidylcholine membranes. Biochemistry 1989; 28: 17–25
- Chen L, Yu ZW, Quinn PJ. The partition of cholesterol between ordered and fluid bilayers of phosphatidylcholine: A synchrotron X-ray diffraction study. Biochim Biophys Acta 2007; 1768: 2873–2881
- Yu ZW, Quinn PJ. Phase stability of phosphatidylcholines in dimethylsulfoxide solutions. Biophys J 1995; 69: 1456–1463
- Yu ZW, Quinn PJ. The effect of dimethyl sulphoxide on the structure and phase behaviour of palmitoleoylphosphatidylethanolamine. Biochim Biophys Acta 2000; 1509: 440–450
- Mannock DA, McIntosh TJ, Jiang X, Covey DF, McElhaney RN. Effects of natural and enantiomeric cholesterol on the thermotropic phase behaviour and structure of egg sphingomyelin bilayer membranes. Biophys J 2003; 84: 1038–1046
- Yan YB, Jiang B, Zhang RQ, Zhou HM. Two-phase unfolding pathway of ribonuclease A during denaturation induced by dithiothreitol. Protein Sci 2001; 10: 321–328
- Yan YB, Zhang RQ, Zhou HM. Biphasic reductive unfolding of ribonuclease A is temperature dependent. Eur J Biochem 2002; 269: 5314–5322
- Yan YB, Wang Q, He HW, Hu XY, Zhang RQ, Zhou HM. Two-dimensional infrared correlation spectroscopy study of sequential events in the heat-induced unfolding and aggregation process of myoglobin. Biophys J 2003; 85: 1959–1967
- McMullen TPW, McElhaney RN. New aspects of the interaction of cholesterol with dipalmitoylphosphatidylcholine bilayers as revealed by high-sensitivity differential scanning calorimetry. Biochim Biophys Acta 1995; 1234: 90–98
- Bruzik KS, Tsai MD. A calorimetric study of the thermotropic behaviour of pure sphingomyelin diastereomers. Biochemistry 1987; 26: 5364–5368
- Bar LK, Barenholz Y, Thompson TE. Effect of sphingomyelin composition on the phase structure of phosphatidylcholine-sphingomyelin bilayers. Biochemistry 1997; 36: 2507–2516
- Ramstedt B, Slotte JP. Comparison of the biophysical properties of racemic and d-erythro-N-acyl sphingomyelins. Biophys J 1999; 77: 1498–1506
- Gao WY, Chen L, Wu FG, Yu ZW. Liquid ordered phase of binary mixtures containing dipalmitoylphosphatidylcholine and sterols. Acta Phys – Chim Sin 2008; 24: 1149–1154
- Matuoka S, Kato S, Hatta I. Temperature change of the ripple structure in fully hydrated dimyristoylphosphatidylcholine/cholesterol multibilayers. Biophys J 1994; 67: 728–736
- Cevc G. Polymorphism of the bilayer membranes in the ordered phase and the molecular origin of the lipid pretransition and rippledlemallae. Biochim Biophys Acta 1991; 1062: 59–69
- Tristram-Nagle S, Moore T, Petrache HI, Nagle JF. DMSO produces a new subgel phase in DPPC, DSC and X-ray diffraction study. Biochim Biophys Acta 1998; 1369: 19–33
- Stamatoff J, Feuer B, Guggenheim HJ, Tellez G, Yamane T. Amplitude of rippling in the Pβ phase of dipalmitoylphosphatidylcholine bilayers. Biophys J 1982; 38: 217–226
- Wack DC, Webb WW. Synchrotron x-ray study of the modulated lamellar phase Pβ′′ in the lecithin-water system. Phys Rev A 1989; 40: 2712–2730
- Meyer HW, Richter W. Freeze-fracture studies on lipids and membranes. Micron 2001; 32: 615–644
- Meyer HW, Bunjes H, Ulrich AS. Morphological transitions of brain sphingomyelin are determined by the hydration protocol: Ripples rearrange in plane, and sponge-like networks disintegrate into small vesicles. Chem Phys Lipids 1999; 99: 111–123
- Koynova R, Caffrey M. Phases and phase transitions of the phosphatidylcholines. Biochim Biophys Acta 1998; 1376: 91–145
- Hicks A, Dinda M, Singer MA. The ripple phase of phosphatidylcholines: Effect of chain length and cholesterol. Biochim Biophys Acta 1987; 903: 177–185
- Copeland BR, McConnell HM. The rippled structure in bilayer membranes of phosphatidylcholine and binary mixtures of phosphatidylcholine and cholesterol. Biochim Biophys Acta 1980; 599: 95–109
- McIntosh TJ, Simon SA, Needham D, Huang CH. Structure and cohesive properties of sphingomyelin/cholesterol bilayers. Biochemistry 1992; 31: 2012–2020
- Hui SW, Stewart TP, Yeagle PL. Temperature-dependent morphological and phase behaviour of sphingomyelin. Biochim Biophys Acta 1980; 601: 271–281
- Chachaty C, Rainteau D, Tessier C, Quinn PJ, Wolf C. Building up of the liquid-ordered phase formed by sphingomyelin and cholesterol. Biophys J 2005; 88: 4032–4044
- Li XM, Momsen MM, Brockman HL, Brown RE. Sterol structure and sphingomyelin acyl chain length modulate lateral packing elasticity and detergent solubility in model membranes. Biophys J 2003; 85: 3788–3801
- Gandhavadi M, Allende D, Vidal A, Simon SA, McIntosh TJ. Structure, composition, and peptide binding properties of detergent soluble bilayers and detergent resistant rafts. Biophys J 2002; 82: 1469–1482
- Simons K, Ikonen E. Functional rafts in cell membranes. Nature 1997; 387: 569–572
- Dietrich C, Bagatolli LA, Volovyk ZN, Thompson NL, Levi M, Jacobson K, Gratton E. Lipid rafts reconstituted in model membranes. Biophys J 2001; 80: 1417–1428
- Yuan CB, Furlong J, Burgos P, Johnston LJ. The size of lipid rafts: An atomic force microscopy study of ganglioside GM1 domains in sphingomyelin/DOPC/cholesterol membranes. Biophys J 2002; 82: 2526–2535
- Róg T, Pasenkiewicz-Gierula M. Cholesterol-sphingomyelin interactions: A molecular dynamics simulation study. Biophys J 2006; 91: 3756–3767
- Lund-Katz S, Laboda HM, McLean LR, Phillipis MC. Influence of molecular packing and phospholipid type on rates of cholesterol exchange. Biochemistry 1988; 27: 3416–3423
- Clarke JA, Heron AJ, Seddon JM, Law RV. The diversity of the liquid ordered (Lo) phase of phosphatidylcholine/cholesterol membranes: A variable temperature multinuclear solid-state NMR and X-ray diffraction study. Biophys J 2006; 90: 2383–2393
- de Almeida RFM, Fedorov A, Prieto M. Sphingomyelin/phosphatidylcholine/cholesterol phase diagram: Boundaries and composition of lipid rafts. Biophys J 2003; 85: 2406–2416