Abstract
Protein palmitoylation is a critical posttranslational modification that regulates protein trafficking, localization, stability, sorting and function. In mammals, addition of this lipid modification onto proteins is mediated by a family of 23 palmitoyl acyl transferases (PATs). PATs often palmitoylate substrates in a promiscuous manner, precluding our understanding of how these enzymes achieve specificity for their substrates. Despite generous efforts to identify consensus motifs defining PAT-substrate specificity, it remains to be determined whether additional factors beyond interaction motifs, such as local palmitoylation, participate in PAT-substrate selection. In this review, we emphasize the role of local palmitoylation, in which substrates are palmitoylated and trapped in the same subcellular compartments as their PATs, as a mechanism of enzyme-substrate specificity. We focus here on non-Golgi-localized PATs, as physical proximity to their substrates enables them to engage in local palmitoylation, compared to Golgi PATs, which often direct trafficking of their substrates elsewhere. PAT subcellular localization may be an under-recognized, yet important determinant of PAT-substrate specificity that may work in conjunction or completely independently of interaction motifs. We also discuss some current hypotheses about protein motifs that contribute to localization of non-Golgi-localized PATs, important for the downstream targeting of their substrates.
Introduction
Lipid modifications facilitate sorting of both soluble and transmembrane proteins to specialized membrane domains (Fukata & Fukata, Citation2010; Linder & Deschenes, Citation2007). Normal signaling relies on the proper localization of lipid-modified substrates to discrete microenvironments, necessary for the formation, maintenance, and function of polarized cells (Fukata & Fukata, Citation2010; Holland & Thomas, Citation2017; Linder & Deschenes, Citation2007). For example, neurons rely heavily on the precise and regulated localization of proteins within axonal and dendritic membrane compartments. The polarized distribution of proteins within neuronal domains promotes axonal growth, anterograde and retrograde signaling, synaptic transmission and proper neuronal excitability and synchronization. Similarly, cardiomyocytes depend on the proper organization of specific proteins along intercalated discs and T-tubules to ensure normal cell-to-cell communication and cardiac contractility. Epithelial cells rely on their polarized membranes to enable the directional transport of solutes, a process integral for their ability to absorb, secrete and protect the intestinal lining. This asymmetrical, but precise organization of proteins within membrane domains is critical for normal physiological function of many diverse cell types, and more broadly contributes to the formation and synchronization of whole mammalian organs. Not surprisingly, many of these neuronal, cardiac and epithelial proteins are lipidated to achieve proper targeting to specialized microdomains, necessary for downstream interactions and function.
One type of lipid modification is S-palmitoylation, which anchors proteins to specialized membrane domains. S-palmitoylation is a reversible lipid modification, acting as a dynamic molecular “switch” to modulate protein functionality and physiological pathways within the cell. S-palmitoylation is predominantly characterized by the covalent addition of a 16-carbon fatty acid, palmitate, onto cysteine residues of proteins. This dynamic lipid modification is mediated by a large family of 23 palmitoyl acyl transferases (PATs), which all share a core aspartate-histidine-histidine-cysteine (zDHHC) motif within the active site of the enzyme, located between transmembrane 2 and 3 (Mitchell et al., Citation2006, Citation2010). The highly conserved zDHHC motif is a cysteine rich (CR) domain, which consists of ∼50 amino acids, whose structure is a variant of the Cys4 zinc-finger-like metal ion binding site, followed by a complex Cys–His region (Putilina et al., Citation1999; Rana et al., Citation2019). This CR domain contains the catalytic site of zDHHC proteins (Putilina et al., Citation1999). PATs have four to six transmembrane domains, with the N- and C-terminal regions and the zDHHC-CR domain oriented toward the cytoplasm, as determined by the transmembrane topology of the yeast PAT Akr1p (Politis et al., Citation2005).
A diverse set of transmembrane and soluble proteins are S-palmitoylated, to promote membrane targeting, protein–protein interactions, protein folding and stability, sorting and function (Blaskovic et al., Citation2013; Fukata & Fukata, Citation2010; Guan & Fierke, Citation2011; Iwanaga et al., Citation2009; Nadolski & Linder, Citation2007; Linder & Deschenes, Citation2007). In fact, proteomics studies have estimated that over 10% of the proteome is palmitoylated (Blanc et al., Citation2015; Sanders et al., Citation2015). A number of palmitoylated proteins have been associated with numerous pathologies such as cancer, bacterial and viral infections, neurodegenerative diseases, intellectual disability, as well as neuropsychiatric diseases (Greaves & Chamberlain, Citation2014; Masurel-Paulet et al., Citation2014; Mukai et al., Citation2004, Citation2008; Raymond et al., Citation2007; Sanders et al., Citation2015; Sobocinska et al., Citation2017; Sutton et al., Citation2013; Woodin et al., Citation2001). Understanding basic mechanisms underlying protein palmitoylation and how palmitoylation mechanisms may become aberrant in disease may shed light on disease etiology.
Groundbreaking studies identified seven yeast zDHHC PATs and 23 mammalian zDHHC PATs, which palmitoylate yeast and mammalian proteins, respectively (Roth et al., Citation2006). Although some PATs exhibit substrate specificity, the promiscuity and functional redundancy amongst the yeast and mammalian PATs have complicated our understanding of PAT-substrate specificity. Studies in Saccharomyces cerevisiae identified Akr1 and Erf2p as PATs for casein kinase Yck2 and small GTPase Ras2, respectively (Babu et al., Citation2004; Bartels et al., Citation1999; Lobo et al., Citation2002; Roth et al., Citation2002), representing important single enzyme-substrate pairs. The yeast SNARE Tlg1 is palmitoylated by Swf1 (Valdez-Taubas & Pelham, Citation2005), while Pf4a was shown to palmitoylate the yeast chitin synthase Chs3 (Lam et al., Citation2006). In contrast, the guanine nucleotide-binding protein alpha-2 subunit Gpa2 is capable of being palmitoylated by four yeast proteins, Akr1, Pfa5, Pfa3 and Erf2, suggesting significant functional redundancy and substrate overlap between yeast PATs (Ohno et al., Citation2012). Similarly, the yeast vacuolar fusion factor Vac8 is palmitoylated predominantly by Pfa3 (Hou et al., Citation2005; Smotrys et al., Citation2005), but can also be palmitoylated by Erf2, Pfa4, Pfa5 and Akr1 (Hou et al., Citation2009). Individual deletion of Pfa3, Erf2, Pfa4, Pfa5 or Akr1 in yeast does not affect Vac8 localization, which is an important function of Vac8 palmitoylation. Only simultaneous genetic deletion of all five yeast PATs results in loss of Vac8 palmitoylation and mis-localization of Vac8, highlighting the overlapping substrate recognition by these yeast PATs (Hou et al., Citation2009). Functional redundancy is also seen with mammalian PATs, such that depletion of a single PAT often results in residual levels of substrate palmitoylation. A common example is the scaffolding protein PSD95, whose palmitoylation is mediated by zDHHC-2, -3, -7 and -15 to regulate AMPAR integration and stability at the excitatory postsynaptic membrane (Fukata et al., Citation2004; Jeyifous et al., Citation2016). A dominant-negative designed against zDHHC2 and zDHHC15 inhibited only 40% of PSD95 palmitoylation, suggesting that residual PSD95 palmitoylation is mediated by its remaining PATs (Noritake et al., Citation2009). The stress-regulated exon (STREX) splice variant of large conductance calcium- and voltage-activated potassium (BK) channel is palmitoylated by zDHHC-3, -5, -7, -9 and -17 to govern STREX plasma-membrane association and determine channel regulation by protein kinase A phosphorylation (Tian et al., Citation2010). Silencing zDHHC-3, -5, -7, -9 and -17 individually by siRNA resulted in significant reduction in STREX palmitoylation, but did not completely abolish STREX palmitoylation, highlighting PAT functional redundancy (Tian et al., Citation2010). Similarly, endothelial nitric oxide (eNOS), whose palmitoylation is critical for its subcellular targeting and nitric oxide production, is palmitoylated by zDHHC-2, -3, -7, -8 and -21 (Fernandez-Hernando et al., Citation2006). Individual knockdown of ZDHHC21 only reduces [H]3-palmitate incorporation into eNOS by 60%, with the remaining 40% of eNOS palmitoylation accounted for by the remaining zDHHC-2,-3,-7 and -8 (Fernandez-Hernando et al., Citation2006). Lastly, the scaffolding protein ankyrin-G is palmitoylated by zDHHC5 and zDHHC8 in a functionally redundant manner to achieve its specific targeting and function at the lateral membrane of epithelial cells (He et al., Citation2014). These examples highlight only a few of many substrates palmitoylated by multiple PATs (Greaves et al., Citation2008, Citation2010; Shmueli et al., Citation2010; Tsutsumi et al., Citation2009). These data demonstrate the promiscuity of PATs in their palmitoylation of multiple substrates, as well as the conserved functional redundancy amongst both yeast and mammalian PATs. Although PAT redundancy has been hypothesized to be important for protein regulation at the level of zDHHC activity, it has also complicated the identification of specific substrate-enzyme pairs.
Elegant studies have shown that PAT enzymes exhibit some substrate specificity (Gorleku et al., Citation2011; Huang et al., Citation2009; Lemonidis et al., Citation2015, Citation2017; Tsutsumi et al., Citation2009), but no consensus sequences have been identified to accurately predict substrate profiles for each PAT. How PATs recognize their respective substrates remains a significant question in the acylation field. There are, however, some established characteristics shared amongst palmitoylated cysteines that likely contribute to a PAT’s ability to select its substrate: (a) myristoylation or prenylation sites often flank the palmitoylated cysteine(s), (b) palmitoylated cysteine(s) are often surrounded by basic or hydrophobic amino acids and (c) palmitoylated cysteine(s) are located in the cytoplasmic regions between or near the transmembrane domains (Salaun et al., Citation2010). Thus, regions surrounding the palmitoylated cysteine play important roles for PAT recognition. This is highlighted by Nadolski & Linder (Citation2009), who showed that the yeast vacuolar fusion protein Vac8, which possesses an SH4 domain containing the three palmitoylated cysteines, is predominantly palmitoylated by Pfa3 in vitro. However, isolated SH4 domain from Vac8 tagged to a fluorescent protein (SH4-GFP) is capable of being palmitoylated by all five yeast PATs, consistent with a previous study by Hou et al. (Hou et al., Citation2009; Nadolski & Linder, Citation2009). Thus, the motif containing the palmitoylation site by itself, in this case the SH4 domain, is often not sufficient to confer PAT specificity. This highlights the importance of considering the full protein sequence to identify PAT recognition sequences, which may be distant from the palmitoylated cysteine. For Vac8, it was determined that the 11th armadillo repeat of Vac8 downstream of its SH4 domain contributes to the specific interaction between Pfa3 and Vac8 (Nadolski & Linder, Citation2009). Given that PATs may need to interact with their substrates at the catalytic CR domain as well as a docking site further away from the active site, it may be that full length proteins possess multiple contact sites that confer PAT specificity (Nadolski & Linder, Citation2009). In contrast, the short fusion protein, Vac8 SH4-GFP, likely only engages with the catalytic DHHC motif present in all PATs, without requiring interaction with any other contact site on the PAT in order to be palmitoylated (Nadolski & Linder, Citation2009).
Neuronal substrates such as huntingtin also exhibit distinct motifs that confer substrate-enzyme specificity. The mammalian PATs, zDHHC13 and zDHHC17 contain an N-terminal ankyrin repeat domain, which contributes significantly to their specificity for huntingtin. In COS cells, overexpressed huntingtin is a substrate for zDHHC17 and zDHHC13, but not for zDHHC3, a PAT which lacks ankyrin repeats. A chimera in which the ankyrin-repeat domain from zDHHC17 is fused to zDHHC3 allows zDHHC3 to interact with huntingtin and mediates huntingtin palmitoylation (Huang et al., Citation2009). This suggests huntingtin relies on its binding to ankyrin repeats for palmitoylation. Thus, residues distant from the palmitoylation motif can provide important context for enzyme-substrate specificity.
Although there is clear evidence that PAT-substrate specificity is partially governed by the amino acid sequence flanking the palmitoylated cysteine (Lemonidis et al., Citation2014, Citation2015, Citation2017; Plain et al., Citation2017), some data argues a stochastic palmitoylation model, in which palmitoylation is governed by the accessibility of cysteine residues of membrane proteins located at a certain depth in the inner leaflet of the membrane, rather than by the local sequence motif (Rodenburg et al., Citation2017). The study demonstrates that genetically introduced cysteine residues in membrane proteins, even in prokaryotic membrane proteins, which are not usually palmitoylated, undergo generic palmitoylation so long as the cysteine residue is accessible to the catalytic center of the PAT, suggesting a lack of specificity for S-palmitoylation (Rodenburg et al., Citation2017). Although this model has yet to be studied in proteins in their native environment, it highlights the complexity underlying PAT-substrate recognition and palmitoylation dynamics that the acylation field is attempting to unravel. Nevertheless, this study reveals the importance of physical proximity between the PAT and its substrate, which in itself may be its own contributor of specificity.
A recent review by Ernst et al. (Citation2019) demonstrated the importance of accounting for the spatial context of PATs, specifically PAT localization within distinct Golgi domains, to better understand anterograde sorting of specific substrates en route to the cell surface. With this in mind, we investigate in this review the possibility that PAT spatial context, or PAT subcellular localization, governs substrate targeting following substrate palmitoylation (). We focus here on non-Golgi-localized PATs, as the localization of their substrates is often determined by the localization of their PATs (). This hypothesis, known as local palmitoylation, in which substrates are palmitoylated and specifically targeted to the same subcellular compartments as that of their respective PATs, may be an important determinant of specificity between non-Golgi-localized PATs and their substrates (Fukata et al., Citation2013; Noritake et al., Citation2009). PAT subcellular localization and local palmitoylation of their substrates may be an under-recognized, yet important predictor of substrate profiles and localization.
Figure 1. Palmitoylation and subcellular trafficking. (a) Golgi-targeted zDHHC PATs mediate the palmitoylation of substrates for substrate stabilization at Golgi membranes prior to their packaging into vesicles and forward-trafficking to the plasma membrane. (b) Three main zDHHC PATs, zDHHC2, zDHHC5 and zDHHC8, are active PATs targeted to the plasma membrane (PM), while zDHHC6 is an active PAT present on ER membranes. These non-Golgi PATs are appropriately positioned to palmitoylate substrates locally, and govern substrate targeting to these same membranes. The precise localization of non-Golgi PATs is critical for the proper non-Golgi-membrane localization of their substrates following palmitoylation, and may be an important predictor of PAT-substrate specificity. Created with BioRender.com.
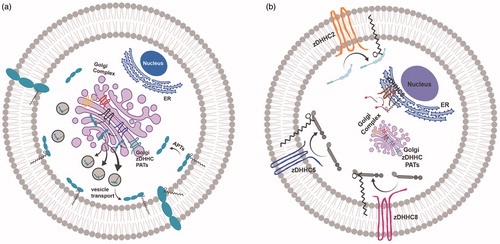
Golgi-localized PATs promote the palmitoylation and forward trafficking of substrates to the plasma membrane
In their important study investigating the subcellular localization of each individual zDHHC PAT by overexpression, Ohno et al. (Citation2006) observed that the majority of PATs localized exclusively to the Golgi. Canonically, the Golgi is recognized as one of the main sorting centers of the secretory pathway (Rocks et al., Citation2010). Most palmitoylated substrates are first palmitoylated at the Golgi and are subsequently trafficked to other subcellular compartments, including the plasma membrane (PM) (). Recently, Ernst et al. (Citation2018) demonstrated that protein palmitoylation promotes anterograde transport of proteins from the Golgi to the PM. Addition of a palmitate onto proteins, mediated by PATs, promotes proteins’ affinity for the highly curved cisternal regions of the Golgi membrane lipid bilayer, where transport occurs, thereby facilitating vesicle budding, sorting and anterograde trafficking of substrates to the PM (Ernst et al., Citation2018). An elegant study by Rocks et al. (Citation2005) highlighted the role of Golgi-localized palmitoylation in directing anterograde trafficking of Ras to the PM. Palmitoylation of farnesylated HRas and NRas in the Golgi targets Ras to the PM. Subsequent de-palmitoylation at the PM drives retrograde trafficking of HRas and NRas back to the Golgi, where Ras can be re-palmitoylated. These palmitoylation/depalmitoylation cycles tightly regulate Ras localization and sorting, as well as fine-tune Ras-dependent signaling (Rocks et al., Citation2005). Ras is palmitoylated by Golgi-localized zDHHC9-GCP16 (Swarthout et al., Citation2005). The precise Golgi localization of zDHHC9 is likely beneficial for direct palmitoylation and subsequent stabilization of Ras at Golgi membranes, before Ras is packaged and trafficked to the PM.
Additionally, palmitoylation of GABA-synthesizing enzyme GAD65 is critical for proper trafficking of GAD65 from Golgi membranes to presynaptic clusters in axon termini (Kanaani et al., Citation2002). Although palmitoylation is not necessary for membrane anchoring of GAD65, palmitoylation promotes sorting of GAD65 to the trans-Golgi network en route to axonal pre-synaptic membranes (Kanaani et al., Citation2008). GAD65 palmitoylation is mediated by Golgi-localized zDHHC17, a Golgi-PAT well positioned to palmitoylate and stabilize GAD65 in the Golgi as it makes its way to the presynaptic membrane surface (Rush et al., Citation2012). Additionally, Golgi-localized PATs such as zDHHC3, -7 and -17 have been shown to enhance endomembrane association of SNARE proteins SNAP25/23, to promote their trafficking to and function at the PM (Greaves et al., Citation2010). Given that Golgi localization of Golgi PAT enzymes play a critical role for Golgi-to-PM protein sorting, it is perhaps unsurprising that many zDHHC PATs reside in the Golgi (Ernst et al., Citation2018; Globa & Bamji, Citation2017; Greaves & Chamberlain, Citation2007; He et al., Citation2014; Ohno et al., Citation2006) (). However, in these cases, the majority of their substrates are trafficked away from the subcellular compartment housing the Golgi PAT.
Plasma membrane PATs mediate local palmitoylation and drive PM-localization of their substrates
Many studies have demonstrated that active PATs also exist in non-Golgi subcellular compartments, including the PM (Brigidi et al., Citation2015; He et al., Citation2014; Howie et al., Citation2014; Ohno et al., Citation2006; Thomas et al., Citation2012) (). In some cases, proteins are palmitoylated first at the Golgi and further palmitoylated in post-Golgi compartments for enhanced regulation and signaling. In other cases, substrates bypass Golgi palmitoylation and undergo palmitoylation in compartments independent of the Golgi to achieve their final destination (He et al., Citation2014). In either case, localization of the relevant PAT seems to play an important role in the ultimate localization of the target protein.
For example, two evolutionarily related and functionally redundant zDHHC PATs, zDHHC5 and zDHHC8, were shown to localize to the PM in HEK293T cells and in polarized epithelial cells (He et al., Citation2014; Ohno et al., Citation2006), and to synaptic membranes in neurons (Brigidi et al., Citation2015; Thomas et al., Citation2012). In hippocampal neurons, zDHHC8 was targeted to the synapse, while zDHHC5 predominantly targeted to dendritic shafts with occasional immunofluorescence signal detected in dendritic spines (Thomas et al., Citation2012). Although Thomas et al. (Citation2012) observed zDHHC5 only at dendritic shafts, Brigidi et al. (Citation2015) have observed zDHHC5 at postsynaptic membranes, likely due to application of pharmacological agents that induce changes in neuronal activity and result in relocalization of zDHHC5 to different neuronal domains, as well as different time-scales of detection. Nevertheless, the unique localization of zDHHC5 and zDHHC8 within neuronal dendritic domains likely contributes to the localization of their substrates following palmitoylation. Indeed, GRIP1b, a known substrate of zDHHC5, colocalizes with zDHHC5 in dendritic puncta in neurons (Thomas et al., Citation2012). Importantly, GRIP1b palmitoylation and localization at dendritic shafts is dependent on both expression as well as proper localization of zDHHC5 at dendritic shafts (Thomas et al., Citation2012). More specifically, zDHHC5-mediated palmitoylation of GRIP1b targets it to dendritic recycling endosomes (REs), where GRIP1b is able to bind to kinesin motor protein KIF5 to mediate the proper trafficking and recycling of GluA2 subunits of α-amino-3-hydroxy-5-methyl-4-isoxazolepropionic acid receptors (AMPARs) (Setou et al., Citation2002) (). Real-time tracking of zDHHC5 demonstrated that zDHHC5 moves on REs as it cycles from dendritic shafts to dendritic spines to palmitoylate its substrates (Brigidi et al., Citation2015), suggesting zDHHC5 may be locally palmitoylating GRIP1b at REs within dendritic shafts (). Although GRIP1b is predominantly palmitoylated by zDHHC5, it can also be palmitoylated by zDHHC8, albeit to a smaller degree since an shRNA specific to Zdhhc8 only slightly reduced GRIP1b palmitoylation in neurons (Thomas et al., Citation2012). Given that zDHHC8 is predominantly localized to dendritic spines, while GRIP1b and zDHHC5 are both predominantly localized to dendritic shafts, including dendritic endosomes, zDHHC5 is judiciously positioned to locally palmitoylate and drive GRIP1b targeting.
Figure 2. Neuronal activity-regulated translocation of zDHHC5 for local palmitoylation of its synaptic substrates. (1a) zDHHC5 cycles from the dendritic shaft to dendritic spines on recycling endosomes (REs) to palmitoylate GRIP1b. (1b) Palmitoylation of GRIP1b by RE-localized zDHHC5 targets GRIP1b to REs to enable GRIP1b binding to KIF5, which mediates the proper trafficking and stabilization of AMPAR GluA2 subunits to the PM (1c). (2a) Under basal conditions, zDHHC5 is stabilized at the postsynaptic membrane through Fyn-mediated phosphorylation, which prevents its association with endocytic machinery. (2b) Upon increase in neuronal activity, zDHHC5 translocates from the postsynaptic membrane to dendritic shafts on REs to recruit its substrates. (2c) Once arrived at the shaft, zDHHC5 palmitoylates its substrate δ-catenin. (2d) Palmitoylated δ-catenin is trafficked to the postsynaptic membrane on REs with zDHHC5. (2e) Palmitoylation-dependent δ-catenin recruitment at post-synaptic membranes binds and stabilizes N-cadherin, leading to enhanced synaptic plasticity. Created with BioRender.com.
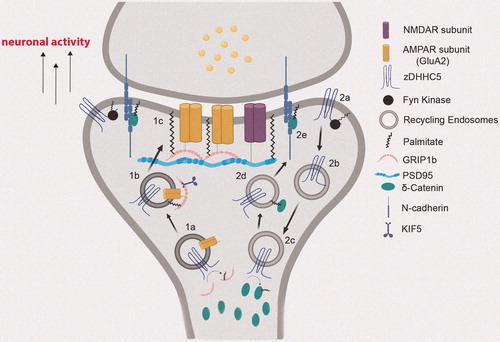
Synaptically targeted zDHHC8 in the neuron is also appropriately positioned to palmitoylate its synaptic substrates in order to promote synaptic transmission and plasticity. Some groups have reported that zDHHC8 resides in vesicle-like clusters along the dendritic shafts in developing hippocampal neurons (Mukai et al., Citation2004), while other groups only detect zDHHC8 at dendritic spines (Thomas et al., Citation2012), perhaps due to species differences or different culture conditions. However, evidence demonstrating that overexpression of zDHHC8 increases spine density and spine stability supports zDHHC8 localization at dendritic spines, at least under overexpression conditions (Moutin et al., Citation2017). Synaptically localized zDHHC8 enables the palmitoylation of the synaptic substrate cdc42. Cdc42 localization in dendritic spines is dependent on its palmitoylation, mediated by the synaptic PAT zDHHC8 (Moutin et al., Citation2017). The regulator of GluA trafficking PICK1 is also a substrate of zDHHC8. zDHHC8-mediated palmitoylation of PICK1 allows for proper cerebellar long-term depression (LTD) induction, a process that occurs in dendritic spines (Thomas et al., Citation2013). Synaptic targeting of zDHHC8 must confer some specificity for its synaptic substrates like PICK1, since zDHHC5, which is not predominantly localized to dendritic spines, did not mediate the palmitoylation of PICK1, despite the presence of the PDZ-binding motif in zDHHC5 and zDHHC8 and the PDZ-containing domain in PICK1 (Thomas et al., Citation2013).
zDHHC5 and zDHHC8 have been shown to be functionally redundant in polarized epithelial cells, likely due to their overlapping localization at the lateral membrane. Ankyrin-G is a known substrate of both zDHHC5 and zDHHC8 in polarized epithelial cells. Of the 23 mammalian PATs, only zDHHC5 and zDHHC8 are specifically localized to the lateral membrane in epithelial cells, where they palmitoylate ankyrin-G to drive its targeting to the lateral membrane (He et al., Citation2014). Proper lateral membrane targeting of ankyrin-G is required for ankyrin-G’s ability to build this membrane through recruitment and scaffolding of important binding partners, such as E-cadherin and βII-spectrin (He et al., Citation2014, Citation2012; Jenkins et al., Citation2015).
Another zDHHC5 substrate which relies on local palmitoylation is the sodium pump regulator phospholemman (PLM). PLM is known to accelerate massive endocytosis (MEND), a process of massive cell surface membrane endocytosis after calcium overload and reperfusion of anoxic cardiac muscle (Lin et al., Citation2013). PLM is a substrate of zDHHC5 (Howie et al., Citation2014). PLM palmitoylation inhibits the sodium pump, which has important implications for calcium homeostasis and cardiac contractility (Tulloch et al., Citation2011). Interestingly, both zDHHC5 and PLM associate by co-immunoprecipitation and localize to caveolin-enriched microdomains at the PM of rat ventricular cardiomyocytes, supporting local palmitoylation of PLM by zDHHC5 at the PM (Howie et al., Citation2014). Although zDHHC2 is also reported to be targeted to the cell surface of cardiac myocytes, it did not associate with PLM, which does not preclude the possibility that zDHHC2 may be capable of palmitoylating PLM (Howie et al., Citation2014). A study demonstrated coexpression of zDHHC5 and zDHHC2 in a human fibroblast-derived cardiac myocyte cell line decreases sodium pump currents, implying increased palmitoylation of PLM, mediated by overexpressed zDHHC2 and zDHHC5. In contrast, dual knockdown of zDHHC2 and zDHHC5 resulted in a 90% increase in sodium pump currents, likely contributed to by loss of PLM palmitoylation as well as reduced MEND, which would recapitulate the ablated MEND phenotype observed in zDHHC5-knockout hearts (Lin et al., Citation2013). These data would suggest zDHHC2 may also be capable of palmitoylating PLM. This is supported by demonstration that dual zDHHC2 and zDHHC5 expression as well as dual knockdown resulted in a significantly greater effect on sodium pump currents, compared to expression or knockdown of zDHHC5 alone (Lin et al., Citation2013). The subcellular localization of the only two known PM-localized zDHHC PATs in cardiac myocytes, zDHHC2 and zDHHC5, is likely critical for specificity towards PLM, whose final destination is also the PM. PLM palmitoylation affects PLM function, but does not affect its ability to associate with the cell surface (Howie et al., Citation2014). Given that PLM is already cell-surface localized prior to its palmitoylation, having a PM-localized PAT like zDHHC5 or zDHHC2 in proximity to mediate local PLM palmitoylation may be favorable.
In neurons, zDHHC2 localizes to mobile dendritic vesicles, as well as the postsynaptic density (PSD) (Fukata et al., Citation2013; Greaves et al., Citation2011; Jeyifous et al., Citation2016; Noritake et al., Citation2009) (). zDHHC2 palmitoylates PSD95, the critical scaffolding protein in the excitatory post-synaptic density (PSD) and regulator of synaptic strength and plasticity (Chen et al., Citation2011). While Golgi-localized zDHHC3 and zDHHC15 palmitoylate PSD95 at the Golgi to promote its membrane trafficking, it is PSD-residing zDHHC2 that palmitoylates and targets PSD95 directly at the PSD, which is required for PSD95-scaffolding of AMPARs and NMDARs at the PSD (Jeyifous et al., Citation2016) (). Given that zDHHC2 is localized to the PSD and that PSD95 must interact with zDHHC2 to be palmitoylated (Jeyifous et al., Citation2016), PSD95 likely undergoes local palmitoylation at the PSD. Fukata et al. (Citation2013) also demonstrated that zDHHC2 puncta co-localize with an antibody that specifically recognizes palmitoylated PDS95 at postsynaptic regions in cultured neurons, providing additional rationale for local palmitoylation of PSD95 at postsynaptic membranes, to generate “PSD95 nanodomains.” These data highlight the importance of proper zDHHC2 localization at the PSD for proper PSD95 palmitoylation and localization at the PSD, critical for the regulation of synaptic strength and plasticity ().
Figure 3. RE- and PSD-localized zDHHC2 locally palmitoylates and targets synaptic substrates to REs and the PSD. (a) PSD95 is constitutively palmitoylated by Golgi-localized zDHHC3 and zDHHC15, which promotes its forward trafficking to the PM. A thioesterase depalmitoylates PSD95 to drive its retrograde trafficking back to the Golgi. (b) Under conditions of decreased neuronal activity, REs containing zDHHC2 deliver zDHHC2 to the PSD. PSD-localized zDHHC2 then interacts with PSD95 to palmitoylate and target PSD95 to the PSD. (c) RE-localized zDHHC2 mediates the palmitoylation of AKAP79/150, to promote the proper delivery of AMPARs at the post-synaptic membrane during LTP. Created with BioRender.com.
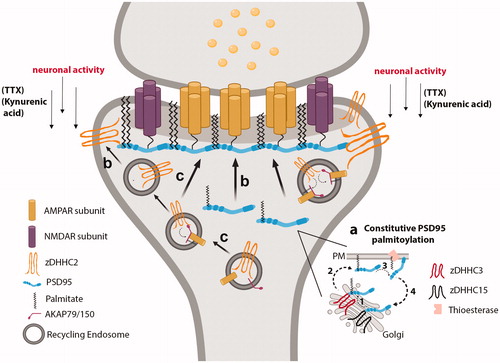
Plasma membrane PATs sense synaptic activity to drive their localization and substrate localization to specific subcellular compartments
If proper surface membrane targeting of non-Golgi PATs determines their substrate’s localization, then understanding how these PATs reach their sites of physiological function may highlight how substrates are selected and where substrates are localized. Some mechanisms regulating the cellular targeting of non-Golgi-associated PATs have been studied. One observed mechanism is the activity-sensing abilities of PATs, such as zDHHC2 and zDHHC5, which govern their specific subcellular localization in response to changing neuronal activity. Noritake et al. demonstrated that zDHHC2 is activity-regulated in the brain: zDHHC2 translocates near the plasma membrane on dendritic vesicles upon decrease in neuronal activity, to increase palmitoylation, localization, and function of its synaptic substrates and maintain synaptic homeostasis (El-Husseini et al., Citation2002; Noritake et al., Citation2009). TIRF microscopy studies demonstrated zDHHC2 rapidly approaches the postsynaptic membrane in response to reduced neuronal activity by kynurenic acid or the voltage-gated sodium channel blocker tetrodotoxin, to increase the palmitoylation and synaptic accumulation of PSD95 at the PSD (Noritake et al., Citation2009) (). Whether zDHHC2-containing vesicles fuse with the membrane or remain intracellular in neurons is unknown. In neuroendocrine PC12 cells, zDHHC2 cycles from REs to the plasma membrane. Fluorescence recovery after photo-bleaching (FRAP) analysis of PC12 cells expressing HA-tagged zDHHC2 shows that zDHHC2 integrates into the plasma membrane, to regulate PSD95 palmitoylation at the PSD (Greaves et al., Citation2011). This is not due to increased enzymatic zDHHC2 activity, but rather from dynamic cycling of zDHHC2 between the PM and Rab11-REs (Greaves et al., Citation2011). Thus, translocation of zDHHC2 between different subcellular compartments in response to activity is not restricted to neuronal cells, and likely contributes to mechanisms of local palmitoylation.
Given that zDHHC2 targeting to the plasma membrane has important implications for PSD95 palmitoylation, PSD formation and integrity, and synaptic strengthening, it would be expected that zDHHC2 targeting to REs would also have important physiological significance. Indeed, zDHHC2 localization at REs enables zDHHC2-mediated palmitoylation and localization of A-kinase anchoring protein (AKAP79/150) in REs (Gold et al., Citation2011; Sanderson et al., Citation2012; Sanderson & Dell'Acqua, Citation2011) (). During long-term synaptic potentiation (LTP), or synapse strengthening, REs within dendritic spines carry synaptic cargo, such as AMPA receptors, and deliver them at synapses through RE exocytosis (Park et al., Citation2006; Park, Citation2004) (). AKAP79/150 facilitates LTP/LTD signaling by regulating the phosphorylation, exocytosis, trafficking, and activity of AMPARs within REs (Jurado et al., Citation2010; Keith et al., Citation2012; Sanderson & Dell'Acqua, Citation2011; Smith et al., Citation2006; Tavalin et al., Citation2002). zDHHC2-mediated palmitoylation of AKAP79/150 is required for its function in regulating dendritic RE exocytosis of AMPA receptors and AMPA receptor-dependent synaptic potentiation (Woolfrey et al., Citation2015) (). Consistent with local palmitoylation, zDHHC2 colocalizes with AKAP79/150 in dendritic REs of cultured hippocampal neurons, revealing that zDHHC2 is well positioned to palmitoylate AKAP79/150 in this specific compartment (Woolfrey et al., Citation2015). AMPARs themselves are palmitoylated (Hayashi et al., Citation2005). Thus far, only Golgi-localized zDHHC3 has been shown to mediate palmitoylation of AMPA receptor subunits (Hayashi et al., Citation2005). Whether zDHHC2 can palmitoylate AMPA receptors remains to be determined. Given that AMPARs and zDHHC2 cycle between the PM and REs, it may be that AMPA receptors cycle in conjunction with zDHHC2 cycling, in response to LTD/LTP, to regulate synaptic transmission.
Specific zDHHC5 localization is also highly driven by synaptic activity, which has important implications for dynamic localization of its substrates. At baseline, zDHHC5 resides in dendritic spines of cultured hippocampal neurons, but cycles between postsynaptic membranes and dendritic shafts on REs in an activity-dependent manner. Following a protocol of chemical LTP (cLTP), zDHHC5 moves from spines to dendritic shafts to recruit and palmitoylate δ-catenin, before translocating and trafficking both itself and palmitoylated δ-catenin back into spines on REs (Brigidi et al., Citation2015). Palmitoylation-dependent δ-catenin recruitment in spines is critical for insertion and stabilization of AMPA receptors into the PSD and synaptic plasticity (Brigidi et al., Citation2014, Citation2015) (). Thus, activity-driven translocation of zDHHC5 to dendritic shafts permits the local palmitoylation of δ-catenin in the dendritic shaft before both proteins cycle back into the spine to enhance synaptic strength and plasticity. This activity-dependent trafficking of zDHHC5 between dendritic spines and shafts is thought to depend on phosphorylation. Under baseline conditions, zDHHC5 is maintained at spine plasma membranes through tyrosine phosphorylation by Fyn kinase within a YxxL motif, thereby rendering zDHHC5 unable to associate with the endocytosis machinery. Upon cLTP activation, Fyn-mediated phosphorylation of zDHHC5 is inhibited through loss of Fyn activation, thereby triggering endocytosis of zDHHC5, and enabling its trafficking to dendritic shafts (Brigidi et al., Citation2015). Thus, phosphorylation is an important regulator of zDHHC5 localization.
To date, there is no evidence demonstrating the activity-sensing capacities of zDHHC8 nor its ability to translocate according to changes in synaptic activity. Perhaps the inability for zDHHC8 to cycle between membrane compartments differentiates substrate specificity between zDHHC5 and zDHHC8. Although zDHHC5 and zDHHC8 operate in a completely functionally redundant manner in the context of ankyrin-G palmitoylation in polarized epithelial cells (He et al., Citation2014), they also exhibit distinct specificity for substrates such as δ-catenin, which is predominantly palmitoylated by zDHHC5 (Brigidi et al., Citation2014, Citation2015). It is possible that distinct substrate profiles (or lack thereof, i.e., functional redundance) for zDHHC5 and zDHHC8 is a cell-type dependent process, depending on cell excitability or polarity. We must keep in mind that PAT identification for a substrate like phospholemman involved co-immunoprecipitation of the substrate with each individual PAT, which precluded identification of zDHHC8 as a potential binding partner due to the difficulties associated with its solubilization. However, PAT-substrate interactions are often transient; thus, failure to coimmunoprecipitate a substrate with its PAT does not rule out the possibility of the PAT-substrate pair. In cases where only zDHHC5 was identified as substrate-specific PAT, there may have been experimental limitations or transient PAT-substrate interactions that precluded the identification of zDHHC8 as capable of palmitoylating that substrate. Nevertheless, whether zDHHC8 can respond to activity needs to be further investigated.
Plasma-membrane PATs contain localization motifs that contribute to their PM subcellular localization
Given that zDHHC2 cycles between the PM and endosomes (), Salaun et al. looked for an existing endocytic sorting signal within the C-terminus of zDHHC2 that could account for zDHHC2’s ability to cycle between PM and REs. The authors identified two atypical, but distinct sequences within the C-terminus of zDHHC2, N357P358 and SxxxL334L335, both of which deviate from the canonical endocytic (Fx)NPxY/F and D/ExxxLL signals, respectively. Both of these play a significant role in zDHHC2 localization as mutation of these individual endocytic sequences to alanine residues resulted in increased retention of mutant zDHHC2 at the PM (Salaun et al., Citation2017). A few phosphorylation sites were also identified in zDHHC2, of which serine-330 is located four amino acids upstream of the dileucine sorting motif. Mutation of this residue in zDHHC2 to an aspartate, to mimic constitutive phosphorylation, results in zDHHC2 internalization and reduces its presence at the PM, whereas mutation to render it phosphorylation-deficient (S330A) results in enhancement of zDHHC2 PM-association. Thus, phosphorylation is able to modulate the effects of the endocytic dileucine motif, which appears to be zDHHC2-specific. Additionally, Ser330 is part of an SQ motif, whose phosphorylation appears to be regulated by synaptic activity (Siddoway et al., Citation2014). That is, synaptic activity would increase phosphorylation of SQ-containing proteins, thereby “activating” the endocytic motif and remove zDHHC2 from the plasma membrane for internalization to REs. Thus, it is possible that phosphorylation dynamics at Ser-330, along with other serine residues in proximity to the dileucine endocytic motif at the C-terminus of zDHHC2, could act to regulate the activity of the sorting motif and thereby the localization of zDHHC2, in an activity-dependent manner (Salaun et al., Citation2017). In fact, it has been more broadly shown that synaptic strength itself can be controlled by phosphorylation dynamics (Woolfrey et al., Citation2015). Depending on whether neuronal activity-dependent phosphorylation dynamics do in fact regulate the endocytic capacities of the sorting signals within zDHHC2, or whether phosphorylation on its own is sufficient to rapidly trigger the trafficking of zDHHC2 from one compartment to the other, these mechanisms could account for zDHHC2’s mobility between the PM and endosomes. It is worth mentioning that other zDHHCs contain phosphorylation sites; as mentioned previously, zDHHC5 phosphorylation regulates its PM-localization (Brigidi et al., Citation2015). Phosphopeptides were identified in zDHHC8 in response to β adrenergic receptor stimulation by mass spectrometry (Lundby et al., Citation2013). Phosphorylation of Golgi-localized zDHHC3 regulates its enzymatic activity and plays a role in neuronal morphogenesis, although it remains unclear whether zDHHC3 phosphorylation regulates zDHHC3 localization (Lievens et al., Citation2016).
Given that clathrin adaptor proteins bind NPxY motifs (Traub & Bonifacino, Citation2013), Salaun et al. (Citation2017) hypothesized the possibility that clathrin adaptor proteins could recognize the atypical NP sorting motif present in zDHHC2 to trigger zDHHC endocytosis, which may or may not be regulated by phosphorylation of proximal serines. The mechanisms underlying the exact role of phosphorylation for zDHHC2 trafficking between PM and endosomes warrant further investigation.
Further studies demonstrated that zDHHC2 enzymatic activity does not drive zDHHC2 localization at the PM of PC12 cells, suggesting that enzyme activity is not responsible for determining how zDHHC2 is targeted to the PM (Greaves et al., Citation2011; Woolfrey et al., Citation2015). Instead, the C-terminal domain of zDHHC2 has been shown to regulate its PM association, given that truncation of the entire C-terminal domain leads to the enzyme redistributing to the ER. Truncating the C-terminus of Golgi-localized zDHHC3, however, did not lead to ER retention, suggesting that not all zDHHCs depend on an intact C-terminal domain to exit the ER (Greaves et al., Citation2011). Rather, this appears to be a zDHHC2-specific phenomenon. Furthermore, zDHHC15, which is homologous to zDHHC2, but is localized to the Golgi, differs in the final half of its C-terminal tail, compared to zDHHC2. The divergent C-terminal sequence between zDHHC2 and zDHHC15 appears to govern the localization, since swapping C-termini reverses the subcellular localization pattern (Greaves et al., Citation2011). Thus, for certain PATs like zDHHC2 and zDHHC15, the C-terminus is a critical trafficking signal.
Polarized epithelial cells provide a feasible cell model system to more thoroughly study the polarized targeting of proteins, as they contain distinctly organized membrane domains with unique protein distributions. Interestingly, overexpressed HA-tagged zDHHC2 in polarized epithelial cells was not found to be PM-associated, but rather localized to intracellular compartments (He et al., Citation2014), highlighting the cell-type-specific targeting of PAT enzymes. In polarized epithelial cells, zDHHC5 and zDHHC8 predominantly localize to the lateral membrane, compared to zDHHC14, which localizes non-specifically throughout the plasma membrane. Unlike zDHHC2 which relies on its intact C-terminus to drive its PM association in nonpolarized cells, the C-termini of zDHHC5 and zDHHC8 do not drive their lateral membrane-targeting in polarized epithelial cells; replacing the C-terminal domain of zDHHC5 with that of zDHHC14 did not abolish lateral membrane localization of zDHHC5 in epithelial cells (He et al., Citation2014). zDHHC5 and zDHHC8 have a conserved AP2μ-binding site or YDNL motif close to a poly-proline SH3-motif as well as dileucine endocytic motifs that can bind endocytosis machinery, such as the clathrin adaptor proteins AP2 (Brigidi et al., Citation2015; Traub & Bonifacino, Citation2013). As Brigidi et al. demonstrated, the ability for zDHHC5 to interact with endocytosis machinery highly influences its ability to cycle between subcellular compartments to regulate synaptic plasticity, and likely affects its ability to locally palmitoylate the substrates it gains access to as it cycles through different subcellular compartments.
Interaction motifs may not be the sole contributor to substrate-enzyme specificity
How non-Golgi PATs like zDHHC2, zDHHC5, or zDHHC8 achieve specificity for their substrates remains incompletely understood. zDHHC2 does not possess any obvious substrate interaction motifs such as a PDZ-binding motif that contribute to substrate recognition (Woolfrey et al., Citation2015). zDHHC5 and zDHHC8 are closely related and distinct from other zDHHC PAT family members as they exhibit greatly extended and disordered C-terminal tails, which in some cases has proven important for substrate recognition (Fukata et al., Citation2004; Ohno et al., Citation2006). Howie et al. (Citation2014) demonstrated that the zDHHC5 disordered C-terminal tail is critical for zDHHC5 to bind to and mediate the palmitoylation of PLM. This extended C-terminal tail contains a PDZ-binding motif predicted to bind other PDZ-containing domains, present in many proteins largely involved in neuronal regulation, and synapse formation and maintenance (Feng & Zhang, Citation2009; Hata et al., Citation1998; Kim & Sheng, Citation2004). Most of the mentioned zDHHC5 and zDHHC8 substrates contain PDZ domains (Howie et al., Citation2014; Thomas et al., Citation2012; Yuan et al., Citation2015), which in some instances explain the ability for zDHHC5 and zDHHC8 to recognize and palmitoylate substrates, like GRIP1b. However, if the presence of PDZ-binding motifs or PDZ-containing domains were the sole contributor to enzyme-substrate specificity, then all zDHHC PATs containing PDZ-binding motifs would likely be capable of palmitoylating all PDZ-containing substrates. However, two other PATs with PDZ-binding motifs, zDHHC3 and zDHHC7, are not capable of palmitoylating any of the aforementioned PDZ-containing, zDHHC5- or zDHHC8-specific substrates like GRIP1b, δ-catenin, and PLM. Interestingly, zDHHC3 and zDHHC7 localize to the Golgi (Ohno et al., Citation2006), compared to plasma membrane targeted zDHHC5 and zDHHC8, which palmitoylate substrates whose predominant final destination is the PM. Thus, subcellular localization of non-Golgi PATs likely contributes to substrate recognition to drive specific substrate localization for proper formation of PM microdomains.
ER-localized PAT zDHHC6 is also involved in local palmitoylation of ER substrates
Although it is most commonly thought that palmitoylation enhances protein affinity for specific microdomains at the plasma membrane, palmitoylome profiling has shown that ER membranes also contain active PATs to palmitoylate proteins directly at the ER. The major ER glycoprotein chaperone calnexin is palmitoylated by ER-localized zDHHC6 in HeLa cells, which targets calnexin to the perinuclear rough ER to stabilize it for substrate capture (Lakkaraju et al., Citation2012). The proper ER localization of calnexin is highly dependent on its palmitoylation, concordant with the necessity for calnexin’s palmitoyl transferase zDHHC6 to be localized to the ER to locally palmitoylate and sort calnexin to its specific ER compartments. ER-localized zDHHC6 was also shown to drive the palmitoylation and subsequent peripheral-ER localization of the E3 ubiquitin ligase, gp78, critically involved in ER-associated degradation pathways (Fairbank et al., Citation2012). Lastly, Fredericks et al. showed that palmitoylation of the inositol 1,4,5-triphosphate receptor (IP3R) by zDHHC6 is critical for IP3R expression and localization at the ER membrane of immune cells, important for calcium release from the ER. IP3R is palmitoylated in a manner dependent on zDHHC6 interacting with another ER-localized selenium-containing protein, selenoprotein K. Both zDHHC6 and selenoprotein K associate through Src-homology 3 (SH3) binding domains (Fredericks et al., Citation2014).
Interestingly, overexpression of zDHHC6 in immune cells did not affect the palmitoylation of other palmitoylated ER substrates, such as the Wnt co-receptor lipoprotein receptor-related protein 6 (LRP6) (Lakkaraju et al., Citation2012). This suggests that zDHHC6 does not mediate the palmitoylation of all ER palmitoylated substrates, and that there must be other active zDHHCs in the ER, such as zDHHC4, as suggested by Gorleku et al (Citation2011). LRP6 must exit the ER, a process mediated by palmitoylation, and travel through the Golgi before reaching the plasma membrane, where it is able to mediate Wnt signaling (Abrami et al., Citation2008; Perrody et al., Citation2016). Given that the functional importance of LRP6 palmitoylation is to drive its sorting away from the ER towards the plasma membrane for further signaling, one could rationalize the recruitment of a different PAT than zDHHC6 to mediate LRP6 palmitoylation, as zDHHC6 has shown to specifically palmitoylate ER substrates that remain in the ER. Additionally, whether palmitoylation of LRP6 actually occurs in the ER, and whether the zDHHC that palmitoylates it is localized to the ER, remain unknown. Abrami et al. (Citation2008) reported that palmitoylation of LRP6 may induce a tilt of the transmembrane domain, in order to allow the protein to conform to the thin lipid membrane of the ER; whether this differential conformation changes PAT specificity remains unknown given that no LRP6-specific PAT has yet been identified.
ER PATs contain localization motifs that contribute to their ER targeting and substrate specificity
Similar to PM PATs, how ER PATs reach the ER is not completely established. ER-localized zDHHC6 contains a dilysine-based ER localization motif located in its C-terminus, conforming to the KKXX ER sorting motif (Gorleku et al., Citation2011). The addition of this dilysine based signal onto the C-terminus of zDHHC3, a Golgi-localized PAT, was sufficient to relocalize zDHHC3 to ER membranes. Interestingly, ER-localized zDHHC3 mutants retained their capacity to palmitoylate known substrates of zDHHC3, SNAP25, and cysteine string protein (CSP). However, a mutant CSP that mislocalizes to the ER is only palmitoylated by an ER-localized zDHHC3 mutant, and cannot be palmitoylated by wild-type, Golgi-localized zDHHC3 (Gorleku et al., Citation2011). Because the CSP mutant is no longer able to localize to the Golgi and instead is relocalized to the ER, Golgi-localized zDHHC3 is also not able to mediate CSP mutant palmitoylation (Gorleku et al., Citation2011). However, it is only when zDHHC3 is forced to an ER membrane that it is able to enhance ER-localized CSP mutant palmitoylation (Gorleku et al., Citation2011), consistent with local palmitoylation.
PAT localization in health and disease
Alterations in protein palmitoylation and aberrant PAT activity have been associated with a wide range of human diseases, including neurological, cardiovascular and infectious diseases. Loss-of-function variants in the Golgi-localized ZDHHC9 are linked to X-linked intellectual disability (XLID) (Han et al., Citation2017; Masurel-Paulet et al., Citation2014; Mitchell et al., Citation2014; Raymond et al., Citation2007; Tzschach et al., Citation2015), sometimes with an increased incidence of epilepsy (Baker et al., Citation2015). zDHHC9 regulates of dendritic growth and inhibitory synapse formation through the palmitoylation of the two GTPases, Ras and TC10, with significant implications for maintaining excitatory/inhibitory (E/I) balance in the brain (Shimell et al., Citation2019). Zdhhc9-knockout mice have altered E/I balance and exhibit seizure-like activity, which could contribute to XLID etiology. (Shimell et al., Citation2019). Genome-wide association studies have found deletions in the region of chromosome 11 that encodes ZDHHC5 in patients with bipolar disorder and schizophrenia (Fallin et al., Citation2004; Schizophrenia Working Group of the Psychiatric Genomics Consortium, Citation2014). A de novo missense mutation in ZDHHC5 has also been reported in schizophrenic patients (Fromer et al., Citation2014). However, which schizophrenic- and bipolar disorder-associated substrates and pathways are altered as a result of alterations in zDHHC5 currently remains unexplored. A number of polymorphisms within the ZDHHC8 gene, specifically those associated with the 22q11.2 chromosomal microdeletion, are also highly linked to schizophrenia (Mukai et al., Citation2004, Citation2008), but its substrate dependence remains incompletely understood. In a 22q11.2 microdeletion mouse model, which lacks functional Zdhhc8, Mukai et al observed decreased postsynaptic PSD95 puncta, as well as decreased presynaptic VGLUT1 puncta, leading to lower spine density and glutamatergic synapses, which could be rescued by re-introduction of enzymatically active zDHHC8 protein (Mukai et al., Citation2008). As expected by the decreased PSD95 puncta in this mouse model, PSD95, as well as other substrates such as Cdc42, Rho GTPases and Rac1, were found to be substrates of zDHHC8 (Mukai et al., Citation2008, Citation2015). Another proteomic study also observed reduced palmitoylation of the vesicular glutamate transporter 1 (VGLUT1), the small GTPase Ras, and myelin basic protein (MBP) in postmortem dorsolateral prefrontal cortices of schizophrenic patients, suggesting that the loss of zDHHC8-mediated palmitoylation of these substrates may contribute to the underlying mechanisms of schizophrenia (Pinner et al., Citation2016). Given the data highlighted in this review, one could predict that human-variant-driven loss of zDHHC8 expression or mistargeting of zDHHC8 at spines would result in loss of proper targeting of PSD95, Cdc42 and PICK1 at dendritic spine membranes, with severe consequences for the formation of synaptic connections and neuronal connectivity, potentially contributing to schizophrenia etiology. Given the critical role of zDHHC5 and zDHHC8 in mediating the palmitoylation of synaptic substrates involved in important processes like synaptic trafficking, transmission and plasticity, it will be important to further investigate how broad dysfunction of these synaptic PATs alter substrates’ capacities to localize to and function at synaptic membranes, and how these enzyme-substrate defects contribute to underlying mechanisms of neuropsychiatric diseases. In light of the role of zDHHC5-mediated palmitoylation of the cardiac phosphoprotein PLM (Howie et al., Citation2014), it would be unsurprising that mutations in ZDHHC5 may also alter cardiac function through PLM’s ability to modulate the cardiac sodium pump or through currently unidentified zDHHC5 cardiac substrates whose plasma-membrane localization is indispensable for function. In the future, investigating disease etiology from the perspective of alterations in PAT expression, localization, and function may provide insights into a much wider set of dysregulated substrates that may converge on one pathophysiological pathway.
Conclusions, current knowledge and outstanding questions
Excluding Golgi-mediated palmitoylation in which a substrate is palmitoylated at the Golgi by Golgi-localized PATs before being trafficked to the plasma membrane, many substrates are locally palmitoylated and targeted to non-Golgi membranes by active PATs residing at PM and ER membranes (non-Golgi PATs). Thus, the precise regulation of substrate localization and function at these PM and ER domains is often highly dependent on the proper PM and ER localization of their respective PATs. Despite improvements in our understanding of PAT enzyme-substrate specificity in recent years, consensus sequences that distinguish which PATs palmitoylate which substrates remain elusive. In this review, we highlight evidence showing that non-Golgi-PAT-substrate specificity is not always contingent on interaction motifs; interaction motifs contribute to a PAT being able to bind and recognize its substrate, but a previously unappreciated factor such as local palmitoylation also appears to be an important determinant of specificity. In fact, we rationalize that for the PAT and its substrate to be able to bind (through an interaction motif), they would need to be in the same subcellular compartment to engage in palmitoylation. In some cases, it may be that both local palmitoylation and an interaction motif are involved in ensuring proper palmitoylation of a substrate by a non-Golgi PAT. Although interaction motifs like PDZ domains clearly play an important role for PAT-substrate recognition, such as zDHHC5 for GRIP1b, we also reviewed examples where non-PDZ-containing substrates were specifically palmitoylated by PATs containing PDZ-binding motifs, such as zDHHC5 and ankyrin-G, suggesting that interaction motifs may not always drive PAT-substrate specificity. Instead, it is worth considering the role of local palmitoylation for substrates like ankyrin-G, where palmitoylation by lateral-membrane-localized zDHHC5 and zDHHC8 are indispensable for ankyrin-G targeting to lateral membranes of epithelial cells. No other PATs are able to mediate the palmitoylation and localization of ankyrin-G in epithelial cells, highlighting that proximity between PATs and substrates within membrane domains may be an important driver of specificity. A better understanding of the role of local palmitoylation for substrate specificity, targeting, and function, as well as its contributions alongside with or independent of interaction motifs, may provide molecular determinants to more effectively map PAT-substrate profiles. An outstanding question that stems from the local palmitoylation hypothesis is how soluble substrates, like ankyrin-G, which do not go through the Golgi for palmitoylation and only rely on PM palmitoylation to reach the membrane, achieve proximity near their respective non-Golgi, PM-associated PATs prior to palmitoylation. Whether these substrates are locally translated or somehow transported near their PAT post-translationally is a question that remains to be answered.
Acknowledgments
We thank Dr. Shaun Sanders and Dr. Dale Martin for their time and their constructive feedback in reviewing this article. We also thank Dr. Kathleen Ignatoski, Amanda France, Dr. Andrew D. Nelson, and Alexandra Bouza for their constructive comments.
Disclosure statement
The authors report no conflicts of interest. The authors alone are responsible for the content and writing of this article.
Additional information
Funding
References
- Abrami L, Kunz B, Iacovache I, van der Goot FG. 2008. Palmitoylation and ubiquitination regulate exit of the Wnt signaling protein LRP6 from the endoplasmic reticulum. Proc Natl Acad Sci USA 105:5384–5389.
- Babu P, Deschenes RJ, Robinson LC. 2004. Akr1p-dependent palmitoylation of Yck2p yeast casein kinase 1 is necessary and sufficient for plasma membrane targeting. J Biol Chem 279:27138–27147.
- Baker K, Astle DE, Scerif G, Barnes J, Smith J, Moffat G, et al. 2015. Epilepsy, cognitive deficits and neuroanatomy in males with ZDHHC9 mutations. Ann Clin Transl Neurol 2:559–569.
- Bartels DJ, Mitchell DA, Dong X, Deschenes RJ. 1999. Erf2, a novel gene product that affects the localization and palmitoylation of Ras2 in Saccharomyces cerevisiae. Mol Cell Biol 19:6775–6787.
- Blanc M, David F, Abrami L, Migliozzi D, Armand F, Bürgi J, et al. 2015. SwissPalm: protein palmitoylation database. F1000Res 4:261.
- Blaskovic S, Blanc M, van der Goot FG. 2013. What does S-palmitoylation do to membrane proteins? Febs J 280:2766–2774.
- Brigidi GS, Santyr B, Shimell J, Jovellar B, Bamji SX. 2015. Activity-regulated trafficking of the palmitoyl-acyl transferase DHHC5. Nat Commun 6:8200.
- Brigidi GS, Sun Y, Beccano-Kelly D, Pitman K, Mobasser M, Borgland SL, et al. 2014. Palmitoylation of delta-catenin by DHHC5 mediates activity-induced synapse plasticity. Nat Neurosci 17:522–532.
- Chen X, Nelson CD, Li X, Winters CA, Azzam R, Sousa AA, et al. 2011. PSD-95 is required to sustain the molecular organization of the postsynaptic density. J Neurosci 31:6329–6338.
- El-Husseini AE-D, Schnell E, Dakoji S, Sweeney N, Zhou Q, Prange O, et al. 2002. Synaptic strength regulated by palmitate cycling on PSD-95. Cell 108:849–863.
- Ernst AM, Syed SA, Zaki O, Bottanelli F, Zheng H, Hacke M, et al. 2018. S-palmitoylation sorts membrane cargo for anterograde transport in the Golgi. Dev Cell 47:479–493 e7.
- Ernst AM, Toomre D, Bogan JS. 2019. Acylation – a new means to control traffic through the Golgi. Front Cell Dev Biol 7:109.
- Fairbank M, Huang K, El-Husseini A, Nabi IR. 2012. RING finger palmitoylation of the endoplasmic reticulum Gp78 E3 ubiquitin ligase. FEBS Lett 586:2488–2493.
- Fallin MD, Lasseter VK, Wolyniec PS, McGrath JA, Nestadt G, Valle D, et al. 2004. Genomewide linkage scan for bipolar-disorder susceptibility loci among Ashkenazi Jewish families. Am J Hum Genet 75:204–219.
- Feng W, Zhang M. 2009. Organization and dynamics of PDZ-domain-related supramodules in the postsynaptic density. Nat Rev Neurosci 10:87–99.
- Fernandez-Hernando C, et al. 2006. Identification of Golgi-localized acyl transferases that palmitoylate and regulate endothelial nitric oxide synthase. J Cell Biol 174:369–377.
- Fredericks GJ, Hoffmann FW, Rose AH, Osterheld HJ, Hess FM, Mercier F, et al. 2014. Stable expression and function of the inositol 1,4,5-triphosphate receptor requires palmitoylation by a DHHC6/selenoprotein K complex. Proc Natl Acad Sci USA 111:16478–16483.
- Fromer M, Pocklington AJ, Kavanagh DH, Williams HJ, Dwyer S, Gormley P, et al. 2014. De novo mutations in schizophrenia implicate synaptic networks. Nature 506:179–184.
- Fukata M, Fukata Y, Adesnik H, Nicoll RA, Bredt DS. 2004. Identification of PSD-95 palmitoylating enzymes. Neuron 44:987–996.
- Fukata Y, Dimitrov A, Boncompain G, Vielemeyer O, Perez F, Fukata M, et al. 2013. Local palmitoylation cycles define activity-regulated postsynaptic subdomains. J Cell Biol 202:145–161.
- Fukata Y, Fukata M. 2010. Protein palmitoylation in neuronal development and synaptic plasticity. Nat Rev Neurosci 11:161–175.
- Globa AK, Bamji SX. 2017. Protein palmitoylation in the development and plasticity of neuronal connections. Curr Opin Neurobiol 45:210–220.
- Gold MG, Stengel F, Nygren PJ, Weisbrod CR, Bruce JE, Robinson CV, et al. 2011. Architecture and dynamics of an A-kinase anchoring protein 79 (AKAP79) signaling complex. Proc Natl Acad Sci USA 108:6426–6431.
- Gorleku OA, Barns A-M, Prescott GR, Greaves J, Chamberlain LH. 2011. Endoplasmic reticulum localization of DHHC palmitoyltransferases mediated by lysine-based sorting signals. J Biol Chem 286:39573–39584.
- Greaves J, Carmichael JA, Chamberlain LH. 2011. The palmitoyl transferase DHHC2 targets a dynamic membrane cycling pathway: regulation by a C-terminal domain. Mol Biol Cell 22:1887–1895.
- Greaves J, Chamberlain LH. 2007. Palmitoylation-dependent protein sorting. J Cell Biol 176:249–254.
- Greaves J, Chamberlain LH. 2014. New links between S-acylation and cancer. J Pathol 233:4–6.
- Greaves J, Gorleku OA, Salaun C, Chamberlain LH. 2010. Palmitoylation of the SNAP25 protein family: specificity and regulation by DHHC palmitoyl transferases. J Biol Chem 285:24629–24638.
- Greaves J, Salaun C, Fukata Y, Fukata M, Chamberlain LH. 2008. Palmitoylation and membrane interactions of the neuroprotective chaperone cysteine-string protein. J Biol Chem 283:25014–25026.
- Guan X, Fierke CA. 2011. Understanding protein palmitoylation: biological significance and enzymology. Sci China Chem 54:1888–1897.
- Han JY, Lee IG, Shin S, Kim M, Jang JH, Park J, et al. 2017. The first patient with sporadic X-linked intellectual disability with de novo ZDHHC9 mutation identified by targeted next-generation sequencing. Eur J Med Genet 60:499–503.
- Hata Y, Nakanishi H, Takai Y. 1998. Synaptic PDZ domain-containing proteins. Neurosci Res 32:1–7.
- Hayashi T, Rumbaugh G, Huganir RL. 2005. Differential regulation of AMPA receptor subunit trafficking by palmitoylation of two distinct sites. Neuron 47:709–723.
- He M, Abdi KM, Bennett V. 2014. Ankyrin-G palmitoylation and betaII-spectrin binding to phosphoinositide lipids drive lateral membrane assembly. J Cell Biol 206:273–288.
- He M, Jenkins P, Bennett V. 2012. Cysteine 70 of ankyrin-G is S-palmitoylated and is required for function of ankyrin-G in membrane domain assembly. J Biol Chem 287:43995–44005.
- Holland SM, Thomas GM. 2017. Roles of palmitoylation in axon growth, degeneration and regeneration. J Neurosci Res 95:1528–1539.
- Hou H, John Peter AT, Meiringer C, Subramanian K, Ungermann C. 2009. Analysis of DHHC acyltransferases implies overlapping substrate specificity and a two-step reaction mechanism. Traffic 10:1061–1073.
- Hou H, Subramanian K, LaGrassa TJ, Markgraf D, Dietrich LEP, Urban J, et al. 2005. The DHHC protein Pfa3 affects vacuole-associated palmitoylation of the fusion factor Vac8. Proc Natl Acad Sci USA 102:17366–17371.
- Howie J, Reilly L, Fraser NJ, Vlachaki Walker JM, Wypijewski KJ, Ashford MLJ, et al. 2014. Substrate recognition by the cell surface palmitoyl transferase DHHC5. Proc Natl Acad Sci USA 111:17534–17539.,
- Huang K, Sanders S, Singaraja R, Orban P, Cijsouw T, Arstikaitis P, et al. 2009. Neuronal palmitoyl acyl transferases exhibit distinct substrate specificity. Faseb J 23:2605–2615.
- Iwanaga T, Tsutsumi R, Noritake J, Fukata Y, Fukata M. 2009. Dynamic protein palmitoylation in cellular signaling. Prog Lipid Res 48:117–127.
- Jenkins PM, He M, Bennett V. 2015. Dynamic spectrin/ankyrin-G microdomains promote lateral membrane assembly by opposing endocytosis. Sci Adv 1:e1500301.
- Jeyifous O, Lin EI, Chen X, Antinone SE, Mastro R, Drisdel R, et al. 2016. Palmitoylation regulates glutamate receptor distributions in postsynaptic densities through control of PSD95 conformation and orientation. Proc Natl Acad Sci USA 113:E8482–E8491.,
- Jurado S, Biou V, Malenka RC. 2010. A calcineurin/AKAP complex is required for NMDA receptor-dependent long-term depression. Nat Neurosci 13:1053–1055.
- Kanaani J, El-Husseini AE-D, Aguilera-Moreno A, Diacovo JM, Bredt DS, Baekkeskov S, et al. 2002. A combination of three distinct trafficking signals mediates axonal targeting and presynaptic clustering of GAD65. J Cell Biol 158:1229–1238.
- Kanaani J, Patterson G, Schaufele F, Lippincott-Schwartz J, Baekkeskov S. 2008. A palmitoylation cycle dynamically regulates partitioning of the GABA-synthesizing enzyme GAD65 between ER-Golgi and post-Golgi membranes. J Cell Sci 121:437–449.
- Keith DJ, Sanderson JL, Gibson ES, Woolfrey KM, Robertson HR, Olszewski K, et al. 2012. Palmitoylation of A-kinase anchoring protein 79/150 regulates dendritic endosomal targeting and synaptic plasticity mechanisms. J Neurosci 32:7119–7136.
- Kim E, Sheng M. 2004. PDZ domain proteins of synapses. Nat Rev Neurosci 5:771–781.
- Lakkaraju AKK, Abrami L, Lemmin T, Blaskovic S, Kunz B, Kihara A, et al. 2012. Palmitoylated calnexin is a key component of the ribosome-translocon complex. Embo J 31:1823–1835.
- Lam KKY, Davey M, Sun B, Roth AF, Davis NG, Conibear E, et al. 2006. Palmitoylation by the DHHC protein Pfa4 regulates the ER exit of Chs3. J Cell Biol 174:19–25.
- Lemonidis K, Gorleku OA, Sanchez-Perez MC, Grefen C, Chamberlain LH. 2014. The Golgi S-acylation machinery comprises zDHHC enzymes with major differences in substrate affinity and S-acylation activity. Mol Biol Cell 25:3870–3883.
- Lemonidis K, Salaun C, Kouskou M, Diez-Ardanuy C, Chamberlain LH, Greaves J, et al. 2017. Substrate selectivity in the zDHHC family of S-acyltransferases. Biochem Soc Trans 45:751–758.
- Lemonidis K, Sanchez-Perez MC, Chamberlain LH. 2015. Identification of a novel sequence motif recognized by the ankyrin repeat domain of zDHHC17/13 S-acyltransferases. J Biol Chem 290:21939–21950.
- Lievens PM-J, Kuznetsova T, Kochlamazashvili G, Cesca F, Gorinski N, Galil DA, et al. 2016. ZDHHC3 tyrosine phosphorylation regulates neural cell adhesion molecule palmitoylation. Mol Cell Biol 36:2208–2225.
- Lin M-J, Fine M, Lu J-Y, Hofmann SL, Frazier G, Hilgemann DW, et al. 2013. Massive palmitoylation-dependent endocytosis during reoxygenation of anoxic cardiac muscle. Elife 2:e01295.
- Linder ME, Deschenes RJ. 2007. Palmitoylation: policing protein stability and traffic. Nat Rev Mol Cell Biol 8:74–84.
- Lobo S, Greentree WK, Linder ME, Deschenes RJ. 2002. Identification of a Ras palmitoyltransferase in Saccharomyces cerevisiae. J Biol Chem 277:41268–41273.
- Lundby A, Andersen MN, Steffensen AB, Horn H, Kelstrup CD, Francavilla C, et al. 2013. In vivo phosphoproteomics analysis reveals the cardiac targets of beta-adrenergic receptor signaling. Sci Signal 6:rs11–rs11.
- Masurel-Paulet A, Kalscheuer VM, Lebrun N, Hu H, Levy F, Thauvin-Robinet C, et al. 2014. Expanding the clinical phenotype of patients with a ZDHHC9 mutation. Am J Med Genet A 164:789–795.
- Mitchell DA, Hamel LD, Reddy KD, Farh L, Rettew LM, Sanchez PR, et al. 2014. Mutations in the X-linked intellectual disability gene, zDHHC9, alter autopalmitoylation activity by distinct mechanisms. J Biol Chem 289:18582–18592.
- Mitchell DA, Mitchell G, Ling Y, Budde C, Deschenes RJ. 2010. Mutational analysis of Saccharomyces cerevisiae Erf2 reveals a two-step reaction mechanism for protein palmitoylation by DHHC enzymes. J Biol Chem 285:38104–38114.
- Mitchell DA, Vasudevan A, Linder ME, Deschenes RJ. 2006. Protein palmitoylation by a family of DHHC protein S-acyltransferases. J Lipid Res 47:1118–1127.
- Moutin E, Nikonenko I, Stefanelli T, Wirth A, Ponimaskin E, De Roo M, et al. 2017. Palmitoylation of cdc42 promotes spine stabilization and rescues spine density deficit in a mouse model of 22q11.2 deletion syndrome. Cereb Cortex 27:3618–3629.
- Mukai J, Dhilla A, Drew LJ, Stark KL, Cao L, MacDermott AB, et al. 2008. Palmitoylation-dependent neurodevelopmental deficits in a mouse model of 22q11 microdeletion. Nat Neurosci 11:1302–1310.
- Mukai J, Liu H, Burt RA, Swor DE, Lai W-S, Karayiorgou M, et al. 2004. Evidence that the gene encoding ZDHHC8 contributes to the risk of schizophrenia. Nat Genet 36:725–731.
- Mukai J, Tamura M, Fénelon K, Rosen AM, Spellman TJ, Kang R, et al. 2015. Molecular substrates of altered axonal growth and brain connectivity in a mouse model of schizophrenia. Neuron 86:680–695.
- Nadolski MJ, Linder ME. 2007. Protein lipidation. Febs J 274:5202–5210.
- Nadolski MJ, Linder ME. 2009. Molecular recognition of the palmitoylation substrate Vac8 by its palmitoyltransferase Pfa3. J Biol Chem 284:17720–17730.
- Noritake J, Fukata Y, Iwanaga T, Hosomi N, Tsutsumi R, Matsuda N, et al. 2009. Mobile DHHC palmitoylating enzyme mediates activity-sensitive synaptic targeting of PSD-95. J Cell Biol 186:147–160.
- Ohno Y, Kashio A, Ogata R, Ishitomi A, Yamazaki Y, Kihara A, et al. 2012. Analysis of substrate specificity of human DHHC protein acyltransferases using a yeast expression system. Mol Biol Cell 23:4543–4551.
- Ohno Y, Kihara A, Sano T, Igarashi Y. 2006. Intracellular localization and tissue-specific distribution of human and yeast DHHC cysteine-rich domain-containing proteins. Biochim Biophys Acta 1761:474–483.
- Park M, Salgado JM, Ostroff L, Helton TD, Robinson CG, Harris KM, et al. 2006. Plasticity-induced growth of dendritic spines by exocytic trafficking from recycling endosomes. Neuron 52:817–830.
- Park M. 2004. Recycling endosomes supply AMPA receptors for LTP. Science 305:1972–1975.
- Perrody E, Abrami L, Feldman M, Kunz B, Urbé S, van der Goot FG, et al. 2016. Ubiquitin-dependent folding of the Wnt signaling coreceptor LRP6. Elife 5:e19083.
- Pinner AL, Tucholski J, Haroutunian V, McCullumsmith RE, Meador-Woodruff JH. 2016. Decreased protein S-palmitoylation in dorsolateral prefrontal cortex in schizophrenia. Schizophr Res 177:78–87.
- Plain F, Congreve SD, Yee RSZ, Kennedy J, Howie J, Kuo C-W, et al. 2017. An amphipathic alpha-helix directs palmitoylation of the large intracellular loop of the sodium/calcium exchanger. J Biol Chem 292:10745–10752.
- Politis EG, Roth AF, Davis NG. 2005. Transmembrane topology of the protein palmitoyl transferase Akr1. J Biol Chem 280:10156–10163.
- Putilina T, Wong P, Gentleman S. 1999. The DHHC domain: a new highly conserved cysteine-rich motif. Mol Cell Biochem 195:219–226.
- Rana MS, Lee CJ, Banerjee A. 2019. The molecular mechanism of DHHC protein acyltransferases. Biochem Soc Trans 47:157–167.
- Raymond FL, Tarpey PS, Edkins S, Tofts C, O’Meara S, Teague J, et al. 2007. Mutations in ZDHHC9, which encodes a palmitoyltransferase of NRAS and HRAS, cause X-linked mental retardation associated with a Marfanoid habitus. Am J Hum Genet 80:982–987.
- Rocks O, Gerauer M, Vartak N, Koch S, Huang Z-P, Pechlivanis M, et al. 2010. The palmitoylation machinery is a spatially organizing system for peripheral membrane proteins. Cell 141:458–471.
- Rocks O, Peyker A, Kahms M, Verveer PJ, Koerner C, Lumbierres M, et al. 2005. An acylation cycle regulates localization and activity of palmitoylated Ras isoforms. Science 307:1746–1752.
- Rodenburg RNP, Snijder J, van de Waterbeemd M, Schouten A, Granneman J, Heck AJR, et al. 2017. Stochastic palmitoylation of accessible cysteines in membrane proteins revealed by native mass spectrometry. Nat Commun 8:1280.
- Roth AF, Feng Y, Chen L, Davis NG. 2002. The yeast DHHC cysteine-rich domain protein Akr1p is a palmitoyl transferase. J Cell Biol 159:23–28.
- Roth AF, Wan J, Bailey AO, Sun B, Kuchar JA, Green WN, et al. 2006. Global analysis of protein palmitoylation in yeast. Cell 125:1003–1013.
- Rush DB, Leon RT, McCollum MH, Treu RW, Wei J. 2012. Palmitoylation and trafficking of GAD65 are impaired in a cellular model of Huntington's disease. Biochem J 442:39–48.
- Salaun C, Greaves J, Chamberlain LH. 2010. The intracellular dynamic of protein palmitoylation. J Cell Biol 191:1229–1238.
- Salaun C, Ritchie L, Greaves J, Bushell TJ, Chamberlain LH. 2017. The C-terminal domain of zDHHC2 contains distinct sorting signals that regulate intracellular localisation in neurons and neuroendocrine cells. Mol Cell Neurosci 85:235–246.
- Sanders SS, Martin DDO, Butland SL, Lavallée-Adam M, Calzolari D, Kay C, et al. 2015. Curation of the mammalian palmitoylome indicates a pivotal role for palmitoylation in diseases and disorders of the nervous system and cancers. PLoS Comput Biol 11:e1004405.
- Sanderson JL, Dell'Acqua ML. 2011. AKAP signaling complexes in regulation of excitatory synaptic plasticity. Neuroscientist 17:321–336.
- Sanderson JL, Gorski JA, Gibson ES, Lam P, Freund RK, Chick WS, et al. 2012. AKAP150-anchored calcineurin regulates synaptic plasticity by limiting synaptic incorporation of Ca2+-permeable AMPA receptors. J Neurosci 32:15036–15052.
- Schizophrenia Working Group of the Psychiatric Genomics Consortium. 2014. Biological insights from 108 schizophrenia-associated genetic loci. Nature 511:421–427.
- Setou M, Seog D-H, Tanaka Y, Kanai Y, Takei Y, Kawagishi M, et al. 2002. Glutamate-receptor-interacting protein GRIP1 directly steers kinesin to dendrites. Nature 417:83–87.
- Shimell JJ, Shah BS, Cain SM, Thouta S, Kuhlmann N, Tatarnikov I, et al. 2019. The X-linked intellectual disability gene Zdhhc9 is essential for dendrite outgrowth and inhibitory synapse formation. Cell Rep 29:2422–2437 e8.
- Shmueli A, Segal M, Sapir T, Tsutsumi R, Noritake J, Bar A, et al. 2010. Ndel1 palmitoylation: a new mean to regulate cytoplasmic dynein activity. Embo J 29:107–119.
- Siddoway B, Hou H, Yang H, Petralia R, Xia H. 2014. Synaptic activity bidirectionally regulates a novel sequence-specific S-Q phosphoproteome in neurons. J Neurochem 128:841–851.
- Smith KE, Gibson ES, Dell'Acqua ML. 2006. cAMP-dependent protein kinase postsynaptic localization regulated by NMDA receptor activation through translocation of an A-kinase anchoring protein scaffold protein. J Neurosci 26:2391–2402.
- Smotrys JE, Schoenfish MJ, Stutz MA, Linder ME. 2005. The vacuolar DHHC-CRD protein Pfa3p is a protein acyltransferase for Vac8p. J Cell Biol 170:1091–1099.
- Sobocinska J, Roszczenko-Jasińska P, Ciesielska A, Kwiatkowska K. 2017. Protein palmitoylation and its role in bacterial and viral infections. Front Immunol 8:2003.
- Sutton LM, Sanders SS, Butland SL, Singaraja RR, Franciosi S, Southwell AL, et al. 2013. Hip14l-deficient mice develop neuropathological and behavioural features of Huntington disease. Hum Mol Genet 22:452–465.
- Swarthout JT, Lobo S, Farh L, Croke MR, Greentree WK, Deschenes RJ, et al. 2005. DHHC9 and GCP16 constitute a human protein fatty acyltransferase with specificity for H- and N-Ras. J Biol Chem 280:31141–31148.
- Tavalin SJ, Colledge M, Hell JW, Langeberg LK, Huganir RL, Scott JD, et al. 2002. Regulation of GluR1 by the A-kinase anchoring protein 79 (AKAP79) signaling complex shares properties with long-term depression. J Neurosci 22:3044–3051.
- Thomas GM, Hayashi T, Chiu S-L, Chen C-M, Huganir RL. 2012. Palmitoylation by DHHC5/8 targets GRIP1 to dendritic endosomes to regulate AMPA-R trafficking. Neuron 73:482–496.
- Thomas GM, Hayashi T, Huganir RL, Linden DJ. 2013. DHHC8-dependent PICK1 palmitoylation is required for induction of cerebellar long-term synaptic depression. J Neurosci 33:15401–15407.
- Tian L, McClafferty H, Jeffries O, Shipston MJ. 2010. Multiple palmitoyltransferases are required for palmitoylation-dependent regulation of large conductance calcium- and voltage-activated potassium channels. J Biol Chem 285:23954–23962.
- Traub LM, Bonifacino JS. 2013. Cargo recognition in clathrin-mediated endocytosis. Cold Spring Harb Perspect Biol 5:a016790–a016790.
- Tsutsumi R, Fukata Y, Noritake J, Iwanaga T, Perez F, Fukata M, et al. 2009. Identification of G protein alpha subunit-palmitoylating enzyme. Mol Cell Biol 29:435–447.
- Tulloch LB, Howie J, Wypijewski KJ, Wilson CR, Bernard WG, Shattock MJ, et al. 2011. The inhibitory effect of phospholemman on the sodium pump requires its palmitoylation. J Biol Chem 286:36020–36031.
- Tzschach A, Grasshoff U, Beck-Woedl S, Dufke C, Bauer C, Kehrer M, et al. 2015. Next-generation sequencing in X-linked intellectual disability. Eur J Hum Genet 23:1513–1518.
- Valdez-Taubas J, Pelham H. 2005. Swf1-dependent palmitoylation of the SNARE Tlg1 prevents its ubiquitination and degradation. Embo J 24:2524–2532.
- Woodin M, Wang PP, Aleman D, McDonald-McGinn D, Zackai E, Moss E, et al. 2001. Neuropsychological profile of children and adolescents with the 22q11.2 microdeletion. Genet Med 3:34–39.
- Woolfrey KM, Sanderson JL, Dell'Acqua ML. 2015. The palmitoyl acyltransferase DHHC2 regulates recycling endosome exocytosis and synaptic potentiation through palmitoylation of AKAP79/150. J Neurosci 35:442–456.
- Yuan L, Seong E, Beuscher JL, Arikkath J. 2015. Delta-catenin regulates spine architecture via cadherin and PDZ-dependent interactions. J Biol Chem 290:10947–10957.