Abstract
We have studied how membrane interactions of two synthetic cationic antimicrobial peptides with alternating α- and β-amino acid residues (“α/β-peptides”) impact toxicity to different prokaryotes. Electron microscopic examination of thin sections of Escherichia coli and of Bacillus subtilis exposed to these two α/β-peptides reveals different structural changes in the membranes of these bacteria. These two peptides also have very different effects on the morphology of liposomes composed of phosphatidylethanolamine and phosphatidylglycerol in a 2:1 molar ratio. Freeze fracture electron microscopy indicates that with this lipid mixture, α/β-peptide I induces the formation of a sponge phase. 31P NMR and X-ray diffraction are consistent with this conclusion. In contrast, with α/β-peptide II and this same lipid mixture, a lamellar phase is maintained, but with a drastically reduced d-spacing. α/β-Peptide II is more lytic to liposomes composed of these lipids than is I. These findings are consistent with the greater toxicity of α/β-peptide II, relative to α/β-peptide I, to E. coli, a bacterium having a high content of phosphatidylethanolamine. In contrast, both α/β-peptides display similar toxicity toward B. subtilis, in accord with the greater anionic lipid composition in its membrane. This work shows that variations in the selectivity of these peptidic antimicrobial peptides toward different strains of bacteria can be partly determined by the lipid composition of the bacterial cell membrane.
Introduction
There has been considerable interest in the application of antimicrobial peptides to combat infectious diseases (Boman [Citation2003]; Papo & Shai [Citation2003]; Powers & Hancock [Citation2003]; Wiese et al. [Citation2003]; Epand & Vogel [Citation1999]) as well as possible application of some of these peptides in cancer chemotherapy (Leuschner & Hansel [Citation2004]). It is well known that prokaryotic cells, as well as cancer cells, tend to have a higher density of anionic lipids on their outer surface than do normal eukaryotic cells, and it is widely believed that this difference in surface charge contributes to the greater sensitivity of bacteria to cationic antimicrobial agents relative to mammalian cells (Papo & Shai [Citation2003]). However, it is not well understood why a particular cationic host-defense peptide is more toxic to some micro-organisms than to others. In addition, the organisms that display the greatest sensitivity vary from peptide to peptide. In this work we present evidence that the different lipid compositions among different bacterial membranes contributes to the bacterial species specificity of the cationic antimicrobial agents we studied.
Many antimicrobial peptides are ribosomally synthesized, and they are found in organisms throughout the phylogenetic kingdoms. Recently, the repertoire of antimicrobial peptides has been expanded through the use of unnatural building blocks, including non-proteinogenic α-amino acids (Patch & Barron [Citation2003]; Oren & Shai [Citation1997]; Shai & Oren [Citation1996]; Steiner et al. [Citation1981]; Zasloff [Citation1987]), β-amino acids (Hamuro et al. [Citation1999]; Porter et al. [Citation2000]; Liu & DeGrado [Citation2001]; Arvidsson et al. [Citation2001]; Porter et al. [Citation2002]) and other types of subunits (Liu et al. [Citation2004]).
A new type of antimicrobial peptide that contains both α- and β-amino acid residues (“α/β-peptides”) has recently been introduced (Schmitt et al. [Citation2004]); examples include α/β-peptides I and II (see ).
Figure 1. (A) Structures of α/β-peptides I and II. (B) Schematic views of α/β-peptides I and II in idealized 14/15-helical conformations, viewed along the helix axis (these images are analogous to “helical wheel” diagrams for α-peptides). These perspectives show that when the α/β-peptides are helical, I is expected to be globally amphiphilic while II is not.
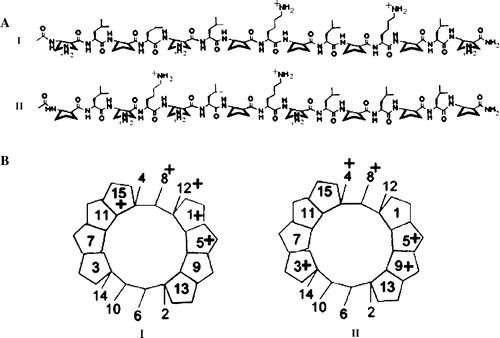
It has been shown that in solution some of these 15-residue peptides appear to adopt a helical conformation containing i,i + 4 C = O–H-N backbone hydrogen bonds, which occur in alternating 14- and 15-membered rings (“14/15-helix”) (Schmitt et al. [Citation2004]). Here we explore the interactions of α/β-peptides I and II with synthetic vesicles and bacterial cells. α/β-Peptide I is designed to have an amphiphilic character in the 14/15-helical conformation, that is, 14/15-helix formation should lead to a global segregation of lipophilic and hydrophilic (cationic) side chains. In contrast, α/β-peptide II should not be amphiphilic in the 14/15-helical conformation. The dramatic difference in reverse-phase HPLC elution of sequence isomers I and II (see ) provides evidence that these design goals have been achieved: I, which should display a large, continuous lipophilic surface in the 14/15-helical conformation, is much more strongly retained on the nonpolar stationary phase than is II, which should not be able to display a large, continuous lipophilic surface in the 14/15-helical conformation. Regardless of the exact conformation of these two peptides, the HPLC results clearly indicate that α/β-peptide I binds more readily to hydrophobic surfaces and should therefore have greater partitioning into membranes. Surprisingly, however, α/β-peptide II has more potent antimicrobial activity than I does against the Gram negative bacterium Escherichia coli (Schmitt et al. [Citation2004]). Analogous comparisons among other peptidic agents yield a very different trend: Conventional peptides with sequences that can form globally amphiphilic α-helices show moderately greater antimicrobial activity relative to isomers that cannot form globally amphiphilic α-helices (Tossi et al. [Citation2000]), and β-peptides with sequences that can form globally amphiphilic helices show even larger activity enhancements relative to isomers that cannot form globally amphiphilic helices (Porter et al. [Citation2002]; Raguse et al. [Citation2002]). Interestingly, although α/β-peptides I and II have very different minimal inhibitory concentrations against E. coli of >100 and 6.3 µg/ml, respectively; against Bacillus subtilis both peptides have the same minimal inhibitory concentrations of 6.3 µg/ml (Schmitt et al. [Citation2004]).
We have tried to identify the origin of this difference in biological activity by examining the effects of I and II on membranes with varying lipid compositions. Our results suggest that the relative toxicity of α/β-peptides I and II against different cell types can be understood in large part by considering the nature of the lipids on the bacterial cell surface.
Materials and methods
Materials
Phospholipids were purchased from Avanti Polar Lipids (Alabaster, AL). Dansyl Polymyxin B (DansylPX), Lipopolysaccharide from E. coli (LPS) and Lipoteichoic acid (LTA) were purchased from the Sigma Chemical Co.
Preparation of α/β-peptide I and II
The synthesis and purification of these compounds have been described (Schmitt et al. [Citation2004]). In the earlier report (Schmitt et al. [Citation2004]) three α/β-peptides were compared. What we designate as α/β-peptide I and II in the present paper refers to α/β-peptides 2 and 3 of the earlier work. The HPLC patterns of α/β-peptide I and II are shown in .
Figure 2. Comparison of reverse phase high pressure liquid chromatography (RP-HPLC) retention profiles of α/β-peptides I and II. Conditions: C8-silica analytical column, linear gradient of acetonitrile in water (with 0.1% TFA) from 20 to 60% over 40 min (the gradient starts upon elution of the solvent front, which occurs approximately 5 min after injection). Ordinate shows absorbance at 220 nm.
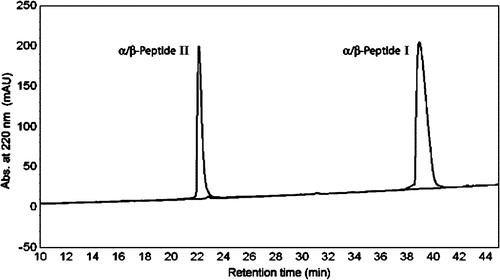
Preparation of phospholipid vesicles
Lipid films were made by dissolving appropriate amounts of lipid in a mixture of chloroform/methanol, 2/1 (v/v), followed by solvent evaporation under nitrogen to deposit the lipid as a film on the wall of a test tube. Final traces of solvent were removed in a vacuum chamber attached to a liquid nitrogen trap, for 2–3 h. Dried films were kept under Argon gas at −30°C if not used immediately. Films were hydrated with buffer and vortexed extensively at room temperature. The lipid suspension was then subjected to five cycles of freezing and thawing and the homogeneous lipid suspensions were then further processed by 10 passes through two stacked 0.1 µm polycarbonate filters (Nucleopore Filtration Products, Pleasanton, CA) in a high pressure barrel extruder (Lipex Biomembranes, Vancouver, BC), at room temperature to extrude large unilamellar vesicles (LUVs). The size of the LUVs were monitored with quasi-elastic light scattering and found to have a diameter of 90±10 Å. The vesicles were kept on ice and used within a few hours of preparation. Lipid phosphorus was determined by the method of Ames (Ames [Citation1966]).
Leakage studies with 8-aminonaphthalene-1,3,6-trisulfonic acid (ANTS)/p-xylene-bis-pyridinium bromide (DPX)
Leakage of aqueous contents from liposomes was determined using the ANTS-DPX assay (Ellens et al. [Citation1985]). Lipid films were hydrated with 12.5 mM ANTS, 45 mM DPX, 68 mM NaCl, 10 mM HEPES, at pH 7.4. The osmolarity of this solution was adjusted to be equal to that of the buffer as measured with a cryoosmometer (Advanced Model 3MOplus Micro-Osmometer, Advanced Instruments Inc., Norwood, MA). LUVs of 0.1 µm diameter were prepared by extrusion as described above. After passage through a 2.5×20 cm column of Sephadex G-75, the void volume fractions were collected, and the phospholipid concentration was determined by phosphate analysis. The fluorescence measurements were performed in 2 ml of the buffer, 10 mM HEPES, 140 mM NaCl, 1 mM EDTA, at pH 7.4 in a quartz cuvette equilibrated at 37°C with stirring. Aliquots of LUVs were added to the cuvette to a final lipid concentration of 50 µM, and the fluorescence was recorded as a function of time with an SLM Aminco Bowman Series II spectrofluorimeter, using an excitation wavelength of 360 nm and an emission wavelength of 530 nm with 8 nm bandwidth slits. A 490 nm cutoff filter was placed in the emission path. Leakage was initiated by addition of the α/β-peptide in buffer solution. We have used fresh solutions of peptide to avoid aggregation due to storage and freezing. The value for 100% leakage was obtained by adding 20 µl of a 20% Lubrol solution to the cuvette. Measurements were performed in duplicate.
31P NMR measurements
Lipid films for NMR were prepared by incorporating 10 mol% of α/β-peptide I or II, dissolved in methanol, into lipid mixtures in choroform:methanol (2:1) and brought to dryness with nitrogen gas and under vacuum for 2 h. The films were hydrated with 20 mM PIPES, 1 mM EDTA, 150 mM NaCl, pH 7.40 buffer (PIPES buffer). The 31P NMR spectra, from suspensions of about 25 mg of dioleoyl phosphatidylethanolamine: dioleoyl phosphatidylglycerol (DOPE: DOPG [2:1]) with or without 10 mol% of α/β-peptide in 200 µl buffer, were obtained using a Bruker DRX-500 spectrometer operating at 202.45 MHz in a 5 mm broad band probe over a 48.6 kHz sweep width in 16×1024 data points. A 90° pulse width of 9.4 µs was used. The sample was contained in a 5 mm diameter Shigemi NMR tube (Shigemi Co., Tokyo, Japan). Composite pulse decoupling was used to remove any proton coupling. Spectra were referenced to phosphoric acid assigned a chemical shift of 0 ppm. Generally, 800 free induction decays were processed using an exponential line broadening of 100 Hz prior to Fourier transformation. Probe temperature was maintained to ±0.2°C by a Bruker BVT 3000 variable temperature unit. Temperatures were monitored with a calibrated thermocouple probe placed in the cavity of the NMR magnet.
X-ray diffraction experiments
A Rigaku-Denki (Model RU-200B) rotating anode was used to obtain nickel-filtered Cu Kα radiation of λ = 1.54 Å. X-rays were focused using a Frank's type camera and recorded with a TEC (Model 205) position sensitive proportional counter. Calibration was done with freshly recrystallized samples of nonadecane, taking spacings for this standard as 26.2 Å. Unoriented samples of the same composition as used for 31P NMR were measured in 1.5 mm outer-diameter glass capillaries. The sample temperature was controlled to ±0.5°C with a thermoelectric device. The samples were prepared by incorporating 10 mol% of α/β-peptide I or II from methanol into the lipid mixture (DOPE: DOPG, 2:1) dissolved in chloroform:methanol, drying the mixture under nitrogen gas and then placed under vacuum for 2 h to produce a lipid film containing a total of 20 mg lipid. The film was hydrated with PIPES buffer and loaded into the capillaries.
Freeze fracture electron microscopy
Samples for freeze fracture electron microscopy were prepared as described for X-ray diffraction measurements. A small volume (∼0.1 µl) of a liposomal preparation was sandwiched between two pieces of thin copper foil and rapidly frozen in liquid propane maintained at 77K. Freeze fracture replicas were prepared as described earlier (Sen et al. [Citation1988]). The replicas were transferred onto electron microscopy grids and cleaned by repeated washing with a mixture of chloroform and methanol 1:1 v/v. The replicas were examined in a Hitachi H600 electron microscope.
Binding to LPS and LTA
Binding to E. coli LPS was carried out according to Fidai et al. ([Citation1997]). Briefly, LPS was first titrated with dansyl polymyxin B (DansylPX) to saturation, in 140 mM NaCl, 10 mM HEPES buffer, pH 7.4, by following the change in fluorescence at 496 nm, when excited at 340 nm. Competition binding by displacement was carried out by adding DansylPX at a concentration that gives 90% saturation of binding to LPS and then adding α/β-peptide sequentially in small aliquots. The decrease in fluorescence was recorded until the subsequent changes in emission intensity became small. A decrease of the initial fluorescence emission from the DansylPX to half of the original value corresponds to displacement of the probe from the outer monolayer of the liposome. A similar assay in modified form of the above was used for binding of LTA (Moore et al. [Citation1986]).
Bacterial morphology using transmission electron microscopy (TEM) with thin sections
E. coli cells, K12 JM109 (from New England BioLabs, MA) or B. subtilis cells, BR151 (from the Bacillus Genetic Stock Center, Columbus, OH) were grown in sterile Luria broth (Miller's modification, from Sigma), incubating overnight at 37°C in a dry shaker. An aliquot of the turbid cell suspension was further grown in Luria broth and samples were taken at intervals to measure the absorbance at 630 nm, until an absorbance of 0.4 (logarithmic growth phase) was reached. Cells were centrifuged at 1000 g for 5 min and suspended in phosphate buffered saline (PBS). They were washed twice with PBS and then 1 ml aliquots were taken into a series of centrifuge tubes and treated with 0, 18 or 72 µg α/β-peptide I or II and incubated for 5 or 45 min at 37°C. They were centrifuged at 1000 g, washed twice with PBS, and then 1 ml 2.5% glutaraldehyde in 0.1 M sodium cacodilate buffer, pH 7.4 was added to fix the cells. After centrifugation at 1000 g for 5 min, they were kept at 4°C overnight. The fixed cells were then washed with cacodilate buffer and post fixed with 1.25% potassium ferrocyanide mixed with 1% buffered OsO4 for 1 h at room temperature. Dehydration with a series of ethanol concentrations from 30–100% was followed by two changes of propylene oxide and gradually embedding the samples in Spurr's Epoxy Resin (Canemco-Marivac Inc., St Laurent, Quebec) going from 50–100% in propylene oxide. After baking overnight in an oven, ultrathin sections were cut approximately 90 nm thick, mounted on 200 mesh Cu/Pd grids and post stained with saturated uranyl acetate (70% ethanol) and Reynolds lead citrate. Sections were examined and photographed in a JEOL (Tokyo, Japan) JEM-200EX Scanning-Transmission Electron Microscope at an acceleration voltage of 80 kV. The images were photographed at 25,000× magnification with a digital camera.
Results
Liposomal leakage of aqueous contents promoted by α/β-peptides
The ANTS/DPX assay that measures the relief of quenching of ANTS by DPX as a consequence of dilution of the liposome contents into the external media was used to measure leakage from LUVs. PE and PG are abundant lipids in the cell membrane of most bacteria. E. coli membranes are particularly rich in PE, while those of B. subtilis have a higher mole fraction of PG. In contrast, mammalian cells have little anionic lipid exposed on the extracellular side of the cell membrane. We have therefore measured the actions of the two α/β-peptides using liposomes of different lipid compositions to mimic the differences found in lipid composition among different cell types. We have simplified the lipid composition of our liposomes by using mixtures with no more than three lipids in different proportions, corresponding to the major lipids of the cells in question. The major lipids of the inner membrane of E. coli are PE (80%) and PG (15%). Using LUVs composed of DOPE: DOPG (2:1) we find that α/β-peptide I causes very little leakage while α/β-peptide II is quite potent at inducing leakage from these vesicles, causing rapid and almost complete release of vesicle content (see A).
Figure 3. Leakage of aqueous contents measured by the ANTS/DPX assay at 37°C of 50 µM LUVs. The concentration of peptide used (2.5–10 µM) is shown on the Figure. (A) LUVs of DOPE: DOPG (2:1) with α/β-peptide I (left panel) or α/β-peptide II (right panel). (B) LUVs of DOPC: DOPG (2:1) with α/β-peptide I (left panel) or α/β-peptide II (right panel). (C) LUVs of DOPG: DOPE: DOPC (70:12:18) with α/β-peptide I (left panel) or α/β-peptide II (right panel). (D) LUVs of DOPC with α/β-peptide I (left panel) or α/β-peptide II (right panel).
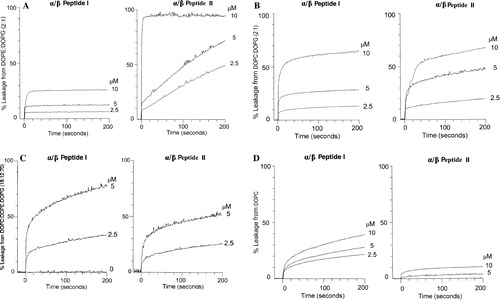
This difference in the potency by which the peptides induce leakage is dependent on the presence of PE. When DOPE in these liposomes is replaced with dioleoyl phosphatidylcholine (DOPC), both α/β-peptides induce a comparable amount of leakage (see B). The higher permeabilizing capacity of α/β-peptide II in liposomes composed of PE and PG is very significant since the E. coli membrane consists mostly of PE, and the PE headgroup is present in the membranes of Gram positive bacteria as well. In the case of the Gram positive bacterium B. subtilis the membrane has 12% PE and 70% PG. In addition, this bacterial membrane also contains 4% of cardiolipin (Clejan et al. [Citation1986]). We have mimicked this composition using a simplified mixture of DOPG: DOPE: DOPC (70:12:18) and shown that with a very high negative charge content and low PE, the α/β-peptides I and II exhibit similar lytic potencies (C).
The α/β-peptide I was more potent in inducing leakage in pure DOPC vesicles, against which II had little lytic activity (D). This is in accord with the higher degree of hemolysis observed for I relative to II (Schmitt et al. [Citation2004]), since the outer leaflet of human erythrocyte membranes is rich in PC.
Lipid phase behavior monitored by 31P NMR
31P NMR in the presence and absence of α/β-peptide I or II was conducted as a function of temperature (see ).
Figure 4. 31P NMR as a function of temperature with DOPE: DOPG (2:1) as control (left column) and in the presence of α/β-peptide I (middle column) or α/β-peptide II (right column). Each sample contained DOPE: DOPG (2:1) with or without 10 mol% of α/β-peptide in 200 µl Pipes buffer pH 7.4.
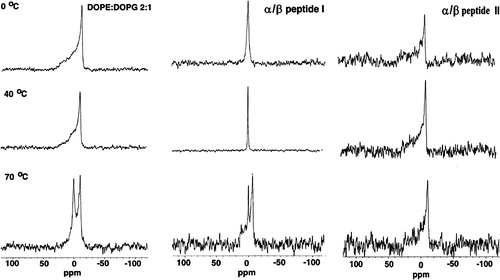
The 31P NMR spectra were obtained from suspensions of DOPE: DOPG (2:1) with or without 10 mol% of α/β-peptide in 200 µl buffer. In the absence of peptide about 25 mg of lipid was used, while for the samples with peptide, a lower amount of lipid was used because of the limited amount of peptide available. This resulted in a somewhat lower signal to noise ratio for the peptide-containing samples. α/β-Peptide I appears to be more perturbing than is II, because I induces the formation of an isotropic peak in DOPE: DOPG (2:1) at room temperature, while the bilayer appears to remain in a lamellar state with II even at elevated temperatures. Only at 70°C do the spectra of lipid with peptide I regain some bilayer characteristics. This may be a consequence of the peptide dissociating from the lipid at high temperature, but we have not investigated this aspect further. This pattern of behavior is interesting because II is much more effective than I at promoting vesicle leakage for this lipid composition (see A). This apparent anomaly is resolved by the X-ray diffraction and freeze fracture microscopy studies described below. An isotropic 31P NMR signal is not indicative of a specific structure since many lipid morphologies can give rise to complete motional averaging of the chemical shift anisotropy.
Small angle X-ray diffraction
We present the X-ray scattering profiles of samples of lipid with and without peptide measured at 25°C (see ). A pure lipid suspension of DOPE: DOPG (2:1) in 20 mM PIPES, 1 mM EDTA, 150 mM NaCl, pH 7.40 buffer gave two broad reflections corresponding to lamellar spacings of 75±2 Å. Addition of 10 mol% of α/β-peptide II resulted in a sharpening of the reflections, allowing for a more precise determination of the d-spacing. Again two orders of lamellar reflections were observed, but with a marked reduction in d-spacing (relative to the absence of II) to 58.0±0.1 Å. In contrast, when 10 mol% of α/β-peptide I was added only continuous scattering was observed.
Figure 5. One-dimensional diffraction patterns of DOPE: DOPG (2:1) measured at 25°C (No Peptide) and incorporating 10 mole% of either α/β-peptide I (middle panel) or α/β-peptide II (bottom panel), plotted against scattering vector q. Depression in the center of the pattern is from the beam stop. Lipid alone shows two orders of lamellar diffraction. Addition of peptide I results in loss of the diffraction peaks, while peptide II causes a broadening and sharpening of the diffraction pattern corresponding to a small spacing.
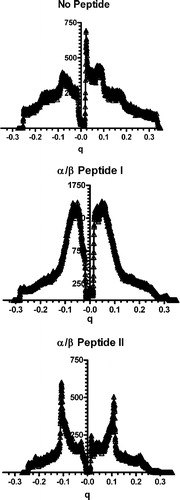
Lipid morphology by freeze fracture electron microscopy
There is a marked difference in morphology between the samples of DOPE: DOPG (2:1) in the presence of α/β-peptide I or II. Addition of α/β-peptide I leads to a corrugated morphology with small domains appearing as a disorganized cubic or sponge phase (see A). The domains observed here are similar to those previously described as “sponge” phases (Gustafsson et al. [Citation1999]; Maldonado et al. [Citation1996]; Noordam et al. [Citation1980]). In contrast, in the presence of α/β-peptide II the mixture forms tightly packed multilamellar vesicles (see B).
Binding of α/β-peptides I and II to lipopolysaccharide (LPS) and to lipoteichoic acid (LTA)
LPS is the major component of the outer membrane of Gram negative bacteria. LTA is the major component of Gram positive bacteria. We find that both I and II bind almost equally well to these negatively charged bacterial membrane components (see ).
Figure 7. Binding to LPS (top) or LTA (bottom) as a function of concentration of either α/β-peptide I or α/β-peptide II, at 25°C was determined by displacement of dansyl polymyxin B. The fluorescence intensity obtained at 495 nm (excitation at 430 nm) is expressed relative to that of LPS-dansyl polymyxin complex in absence of α,β-peptide.
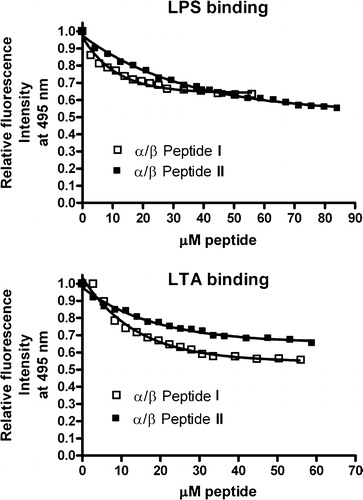
Therefore, the explanation for the difference in activities between these two α/β-peptides cannot arise from a preferential binding to LPS or LTA. However, the affinities of I and II for both LPS and LTA does indicate that both of these α/β-peptides are drawn to the external membranes of Gram negative and Gram positive bacteria.
α/β-Peptides damage E. coli morphology
Both α/β-peptides cause damage to E. coli as well as the other bacteria used and cause changes in the bacterial membranes. However, these membrane transformations are different with regard to the speed at which they are induced by each α/β-peptide and in the amount of α/β-peptide required to produce them. Both α/β-peptides cause marked changes in the E. coli membrane, as indicated by electron microscopy (see ).
Figure 8. Transmission electron microscopy of thin sections of fixed E. coli bacteria in the presence of α/β-peptide I and α/β-peptide II, at 25,000× magnification. Bars correspond to 200 nm. (A) Left, untreated E.coli bacteria, after 5 min incubation at 37°C. Middle, bacteria treated with low concentrations (18 µg/ml) of α/β-peptide II after 5 min incubation. Membrane alteration with loss of contents is already visible. Right, higher concentrations (72 µg/ml) of α/β-peptide II after 5 min incubation at 37°C; dramatic changes in morphology are present, i.e., blebbing, loss of internal contents, membrane joining, etc. (B) Two images each at 45 min incubation, with either α/β-peptide I (left) or α/β-peptide II (right) (72 µg/ml). These images show the progression of the membrane damage observed in A. Arrows mark regions where α/β-peptide I causes separation of inner and outer membranes (left side) or where α/β-peptide II results in adherence of the two membranes (right side). Regions highlighted by arrows are also shown in C in an expanded form. (C) Expanded view (∼10×) of sections of above four images at 45 min incubation shown in 8B.
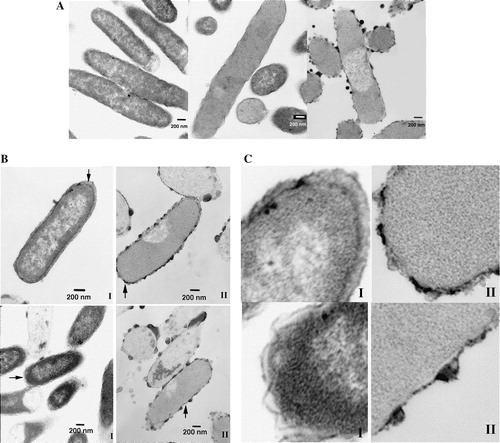
There is, however, a striking difference in the action of the two peptides, as the potency of II in inducing these damaging effects is much greater than that of I. After 45 min of incubation in the presence of I, the inner and outer membranes are well differentiated and the space between them appears more expanded than normal, but the bacteria have generally retained their integrity (see B and 8C). In contrast, during the same time period II induces extensive blebbing and massive loss of internal contents. In addition, the inner and outer bacterial membranes are difficult to resolve as two distinct membranes after treatment with II (C). With II, the onset of blebbing and loss of internal contents is very rapid, as these effects are detected almost as soon as the α/β-peptide is added (A). The protocol used in determining the minimal inhibitory concentration (MIC) (18), differs greatly from the one used for the EM studies described here, and consequently the amount of α/β-peptide required for lethality is likely to differ between these two experiments. Changes can be observed at relatively low concentrations of II and short incubation times but images resulting from exposure to higher concentrations show even more dramatic changes. For example, with 18 µg/ml of II, even after 5 min of incubation, drastic changes can already be visualized by EM, including loss of internal contents through a damaged membrane. With 72 µg/ml of II, after 5 min of incubation, a large number of bacteria had lost their membrane integrity, presenting extensive blebbing (A). On the other hand, even after 45 min of incubation with 72 µg/ml of α/β-peptide I, most of the bacteria remain intact, although some cells begin to show membrane damage (B).
α/β-Peptides are toxic to B. subtilis without gross change in membrane morphology
Both elongated and round bacterial morphology is found in our specimens. The sensitivity of B. subtlis to shape change is known (Burdett [Citation1979]). After 5 min of incubation of this bacterium with either peptide I or II there is little morphological change compared with the control or the sample at zero time (not shown). After 45 min of incubation both peptides I and II cause similar loss of cell viability in accord with their similar MIC, with the membranes partially disrupted leading to loss of internal contents (). For this bacterium the peptides do not cause much blebbing or protrusions, as was found with E. coli.
Figure 9. Transmission electron microscopy of thin sections of fixed B. subtilis incubated for 45 min at 37°C. Magnification 25,000×. Bars correspond to 200 nm. Left, without any additions. Middle, incubated in the presence of 72 µg/ml of peptide I. Right, incubated in the presence of 72 µg/ml of peptide II. While live bacteria predominate in the control, non-viable ones predominate in the presence of either peptide. The bacterial membrane is compromised in the same way by the presence of peptide, where leakage of internal contents is visible. Arrows mark regions of membrane rupture.
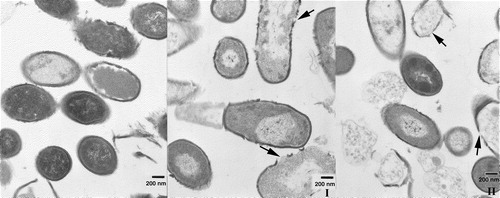
Discussion
The lipid composition of the E. coli inner membrane includes about 80% PE and 15% PG (Kol et al. [Citation2003]). Previous results (Schmitt et al. [Citation2004]) show that α/β-peptide II is more toxic toward E. coli than is I, even though α/β-peptide II is less able to bind to hydrophobic surfaces (). This order of toxicity, however, is in accord with our findings that α/β-peptide II is more lytic to vesicles containing a high mol fraction of PE (A). This selectivity for α/β-peptide II is unique to vesicles that contain a high mol fraction of PE, among the lipid compositions we have examined (). E. coli is a Gram negative bacteria and has an outer membrane rich in LPS. It is necessary for the peptides to enter the periplasmic space in order to damage the bacterial plasma membrane. Both α/β-peptides I and II have similar affinities for LPS (), which makes it unlikely that the greater sensitivity of E. coli to II relative to I results from α/β-peptide interactions with the outer membrane. In addition, the EM evidence indicates that both peptides can damage the cell membrane, indicating that their access to the periplasmic space is not completely blocked. Lastly, α/β-peptide II more toxic to E. coli than it is to several other Gram positive bacteria. It is unlikely that II could have this high potency if it did not enter the periplasmic space and also unlikely that α/β-peptide I, of similar size and charge, would have very different rates of entry through the large pores of the outer membrane. Nevertheless, interaction of the α/β-peptides with the outer membrane may be responsible for some of the morphological changes we observe with E. coli (). It has been proposed that the aggregation of polycations contributes to their crossing of the outer membrane of Gram negative bacteria (Chapple et al. [Citation2004]), and this outer membrane interaction could underlie the formation of blebs at the surface of bacteria when exposed to some antimicrobial peptides (Oren et al. [Citation1999]).
Although α/β-peptide II is more toxic to bacteria having a high membrane PE content and is more effective at inducing leakage in PE-containing vesicles, it does not perturb the 31P NMR bilayer powder pattern of DOPE: DOPG (2:1) (). In fact, II suppresses the isotropic component of the spectra seen with the lipid alone at 70°C (). In contrast, I, which is less toxic to E. coli and induces less leakage from PE-containing vesicles, exerts a more profound effect on DOPE: DOPG (2:1), according to 31P NMR powder patterns, leading to the formation of an isotropic phase. This apparent anomaly can be understood in conjunction with the X-ray diffraction and electron microscopy results as described below.
Freeze fracture electron microscopy of DOPE: DOPG (2:1) mixtures in the presence of α/β-peptide I shows undulations and the formation of small particles (A). In general, these particles do not have long range order. This morphology may correspond to what others have described as a “sponge phase” (Gustafsson et al. [Citation1999]; Maldonado et al. [Citation1996]). Other indications consistent with the suggestion that DOPE: DOPG (2:1) in the presence of I forms a sponge phase include the observations that it gives an isotropic 31P NMR powder pattern () and that this peptide–lipid mixture does not exhibit any sharp peaks in X-ray diffraction (), indicating that it has no significant long range order (Noordam et al. [Citation1980]).
However, the strongest evidence for the presence of this phase comes from the freeze fracture electron microscopy (A). The very high molecular weight aggregate of a sponge phase would not form rapidly from individual unilamellar liposomes because the rate would be limited by the rate of vesicle-vesicle contact. The α/β-peptide I therefore would not give rise to rapid leakage of internal contents (A). Thus, although α/β-peptide I can induce more extensive morphological change in liposomes rich in PE, this change in phase requires vesicle–vesicle contact and does not occur rapidly with LUVs or with bacteria. Hence bacteria rich in PE, such as E. coli are not particularly sensitive to the toxic action of α/β-peptide I. Similarly in B. subtilis, a bacterium that is particularly rich in PG, a lipid with no tendency to form inverted phases (cardiolipin concentration is relatively low and it forms inverted phases only in the presence of divalent cations), lipid polymorphism per se does not contribute to the toxicity of α/β-peptide I.
Cubic phases have many similarities to sponge phases, and one would expect that physical properties that promote one of these phases would also favor the other. We therefore suggest that α/β-peptide I alters the physical properties of the membrane in the same manner as do peptides that promote cubic phase formation. There are several examples of natural antimicrobial peptides, including alamethicin (Keller et al. [Citation1996]; Angelova et al. [Citation2000]), gramicidin S (Staudegger et al. [Citation2000]) and pardaxin (Hallock et al. [Citation2002]), that have been found to promote the formation of cubic phases. Several of these peptides, including alamethicin and pardaxin as well as melittin (Colotto et al. [Citation1991]) and the influenza virus fusion peptide promote the formation of cubic lipid phases (Colotto & Epand [Citation1997]; Siegel & Epand [Citation2000]). All of these peptides can fold into globally amphiphilic helices, as is also the case with α/β-peptide I.
α/β-Peptide II does not promote the formation of non-bilayer phases, as measured by 31P NMR, X-ray diffraction or freeze fracture electron microscopy. However, α/β-peptide II drastically reduces the d-spacing of suspensions of DOPE: DOPG (2:1). In the absence of II, this lipid mixture has a large d-spacing with a broad lamellar reflection, as is commonly found for charged lipid bilayers. However, in the presence of α/β-peptide II, the d-spacing is reduced by almost 20 Å, to a value not very much larger than that of a pure PE lipid and the diffraction peak is sharper (see ). It seems likely that the folded topology of α/β-peptide II, in which charged side chains are distributed around the helix axis, allows it to form a bridge between juxtaposed lipid bilayers, reducing their electrostatic repulsion. An analogous situation occurs with the compaction of multilayers containing anionic lipids by myelin basic protein (Mateu et al. [Citation1973]), a protein that is rich in cationic residues. We thus suggest that peptide II causes compaction of bilayers because of the distribution of charged groups about the helix axis, unlike peptide I where the charges are on one face. It is likely that the reduction in d-spacing promoted by α/β-peptide II will result in toxicity toward bacteria such as E. coli that have a high content of PE in their membranes and have two adjacent membranes that can stack together. Since PC is a more hydrated lipid, it would be anticipated that these effects would be less pronounced in membranes in which PC replaced PE. For example, there is less leakage of DOPC: DOPG (2:1) mixtures compared with DOPE: DOPG (2:1) in the presence of α/β-peptide II (see ).
We also suggest that certain alternative mechanisms are less likely. Peptide-induced changes in intrinsic membrane monolayer curvature can destabilize bilayers. However, studies of the shifts in the bilayer to hexagonal phase transition temperature of dipalmitoleoyl phosphatidylethanolamine gave similar results for both α/β-peptides (not shown), indicating that the difference in the destabilization of bilayers by these peptides is not a consequence of peptide-induced changes in intrinsic membrane curvature. Another mechanism by which certain antimicrobial peptides cause leakage through membranes is by the formation of a toroidal pore. The formation of such a pore is accompanied by rapid rates of phospholipid transbilayer diffusion (Matsuzaki [Citation1998]). However, although the two α/β-peptides had different lytic activity, they both induced similar transbilayer diffusion rates in several lipid systems (data not shown).
The types of morphological changes that α/β-peptides I and II produce in vesicles that mimic the lipid composition of E. coli membranes resemble the changes that occur in the bacterial membrane itself in the presence of these two α/β-peptides. α/β-Peptide II causes compaction of anionic MLVs and in the case of E. coli this peptide reduces the spacing between inner and outer membranes of this Gram-negative bacteria (). α/β-Peptide I causes a ruffling of the membrane of E. coli and sites at which the membrane eventually ruptures (). The ruffling observed in the bacteria resembles the morphological changes induced by I in the liposomes (A).
The cell membrane of the Gram positive bacterium B. subtilis has an exceptionally high content of anionic PG headgroups, corresponding to approximately 70% of the polar lipid composition of the membrane, and this membrane also contains 4% cardiolipin (Clejan et al. [Citation1986]). α/β-Peptides I and II are very similar in their toxic effects toward B. subtilis (Schmitt et al. [Citation2004]). It seems likely that this similarity arises because both α/β-peptides bear a high positive charge, and they are therefore driven electrostatically to interact with the highly anionic membrane of B. subtilis. At lower PE contents of the membrane the difference in leakage potency of α/β-peptides I and II is small (C). Therefore, when charge interactions predominate, there is little difference in the potency between α/β-peptides I and II. Analysis of thin sections of B. subtilis that have been exposed to the peptides shows that both α/β-peptides I and II behave similarly, consistent with electrostatic interactions predominating.
In the absence of electrostatic interactions, it would be anticipated that partitioning of these peptides into zwitterionic lipids, such as PC, would be determined largely by hydrophobic interactions. The suggested greater segregation of hydrophobic groups in α/β-peptide I can explain the observation that this peptide interacts more strongly with pure PC membranes than does α/β-peptide II. This is consistent with the observed greater retention of α/β-peptide I in reverse phase HPLC columns (). This functional difference between isomers I and II is manifested in vesicle leakage data (I is more effective at inducing leakage from DOPC LUVs than is II, D). The greater ability of I relative to II to disrupt DOPC vesicles is likely correlated to the fact that I is more effective than II at lysing human red blood cells (Schmitt et al. [Citation2004]), which have predominantly zwitterionic lipids on their outer surface.
Our studies demonstrate a relationship between the selectivity of related antimicrobial peptides for two different strains of bacteria and the lipid composition of these bacterial membranes. In addition, we show that rearrangements caused by isomeric α/β-peptides I and II in lipid membranes can explain some of the effects of these agents on intact bacteria. The markedly different effects of the two peptides on lipids help to explain why the less hydrophobic peptide, α/β-peptides II, is more toxic to E. coli. These results should contribute to the rational design of agents that manifest selective toxicity targeted to specific bacterial species.
This work was supported by grants from the CIHR (MT-7654, to R.M.E.) and NSF (CHE-0140621, to S.H.G.). M.A.S. was supported in part by a Molecular Biophysics training grant from NIGMS (T32 GM08293). R.M.E. is recipient a CIHR Senior Investigator Award. We are grateful to Marnie Tedeske for providing her superb expertise and kind assistance with the EM micrographs.
References
- Ames BN. Assay of inorganic phosphate, total phosphate and phosphatases. Meth Enzymol 1966; 8: 115–118
- Angelova A, Ionov R, Koch MH, Rapp G. Interaction of the peptide antibiotic alamethicin with bilayer- and non-bilayer-forming lipids: Influence of increasing alamethicin concentration on the lipids supramolecular structures. Arch Biochem Biophys 2000; 378: 93–106
- Arvidsson PI, Frackenpohl J, Ryder NS, Liechty B, Petersen F, Zimmermann H, Camenisch GP, Woessner R, Seebach D. On the antimicrobial and hemolytic activities of amphiphilic beta-peptides. Chembiochem 2001; 2: 771–773
- Boman HG. Antibacterial peptides: Basic facts and emerging concepts. J Intern Med 2003; 254: 197–215
- Burdett ID. Electron microscope study of the rod-to-coccus shape change in a temperature-sensitive rod- mutant of Bacillus subtilis. J Bacteriol 1979; 137: 1395–1405
- Chapple DS, Hussain R, Joannou CL, Hancock RE, Odell E, Evans RW, Siligardi G. Structure and association of human lactoferrin peptides with Escherichia coli lipopolysaccharide. Antimicrob Agents Chemother 2004; 48: 2190–2198
- Clejan S, Krulwich TA, Mondrus KR, Seto-Young D. Membrane lipid composition of obligately and facultatively alkalophilic strains of Bacillus spp. J Bacteriol 1986; 168: 334–340
- Colotto A, Epand RM. Structural study of the relationship between the rate of membrane fusion and the ability of the fusion peptide of influenza virus to perturb bilayers. Biochemistry 1997; 36: 7644–7651
- Colotto A, Lohner K, Laggner P. Small-angle X-ray-diffraction studies on the effects of melittin on lipid bilayer assemblies. J Appl Crystallography 1991; 24: 847–851
- Ellens H, Bentz J, Szoka FC. H+- and Ca2 + -induced fusion and destabilization of liposomes. Biochemistry 1985; 24: 3099–3106
- Epand RM, Vogel HJ. Diversity of antimicrobial peptides and their mechanisms of action. Biochim Biophys Acta 1999; 1462: 11–28
- Fidai S, Farmer SW, Hancock RE. Interaction of cationic peptides with bacterial membranes. Meth Mol Biol 1997; 78: 187–204
- Gustafsson J, Nylander T, Almgren M, Ljusberg-Wahren H. Phase behavior and aggregate structure in aqueous mixtures of sodium cholate and glycerol monooleate. J Colloid Interface Sci 1999; 211: 326–335
- Hallock KJ, Lee DK, Omnaas J, Mosberg HI, Ramamoorthy A. Membrane composition determines pardaxin's mechanism of lipid bilayer disruption. Biophys J 2002; 83: 1004–1013
- Hamuro Y, Schneider JP, DeGrado WF. De novo design of antibacterial beta-peptides. J Am Chem Soc 1999; 121: 12200–12201
- Keller SL, Gruner SM, Gawrisch K. Small concentrations of alamethicin induce a cubic phase in bulk phosphatidylethanolamine mixtures. Biochim Biophys Acta 1996; 1278: 241–246
- Kol MA, van Laak AN, Rijkers DT, Killian JA, de Kroon AI, de Kruijff B. Phospholipid flop induced by transmembrane peptides in model membranes is modulated by lipid composition. Biochemistry 2003; 42: 231–237
- Leuschner C, Hansel W. Membrane disrupting lytic peptides for cancer treatments. Curr Pharm Des 2004; 10: 2299–2310
- Liu D, DeGrado WF. De Novo design, synthesis and characterization of antimicrobial-peptides. J Am Chem Soc 2001; 123: 7553–7559
- Liu DH, Choi S, Chen B, Doerksen RJ, Clements DJ, Winkler JD, Klein ML, DeGrado WF. Nontoxic membrane-active antimicrobial arylamide oligomers. Angewandte Chemie – Int Ed 2004; 43: 1158–1162
- Maldonado A, Urbach W, Ober R, Langevin D. Swelling behavior and local topology of an L(3) (sponge) phase. Phys Rev 1996; 54: 1774–1778
- Mateu L, Luzzati V, London Y, Gould RM, Vosseberg FG. X-ray diffraction and electron microscope study of the interactions of myelin components. The structure of a lamellar phase with a 150 to 180 A repeat distance containing basic proteins and acidic lipids. J Mol Biol 1973; 75: 697–709
- Matsuzaki K. Magainins as paradigm for the mode of action of pore forming polypeptides. Biochim Biophys Acta 1998; 1376: 391–400
- Moore RA, Bates NC, Hancock RE. Interaction of polycationic antibiotics with Pseudomonas aeruginosa lipopolysaccharide and lipid A studied by using dansyl-polymyxin. Antimicrob Agents Chemother 1986; 29: 496–500
- Noordam PC, van Echteld CJ, de Kruijff B, Verkleij AJ, de Gier J. Barrier characteristics of membrane model systems containing unsaturated phosphatidylethanolamines. Chem Phys Lipids 1980; 27: 221–232
- Oren Z, Lerman JC, Gudmundsson GH, Agerberth B, Shai Y. Structure and organization of the human antimicrobial peptide LL-37 in phospholipid membranes: Relevance to the molecular basis for its non-cell-selective activity. Biochem J. 1999; 341(3)501–513
- Oren Z, Shai Y. Selective lysis of bacteria but not mammalian cells by diastereomers of melittin: Structure-function study. Biochemistry 1997; 36: 1826–1835
- Papo N, Shai Y. Can we predict biological activity of antimicrobial peptides from their interactions with model phospholipid membranes?. Peptides 2003; 24: 1693–1703
- Patch JA, Barron AE. Helical peptoid mimics of magainin-2 amide. J Am Chem Soc 2003; 125: 12092–12093
- Porter EA, Wang X, Lee HS, Weisblum B, Gellman SH. Non-haemolytic beta-amino-acid oligomers. Nature 2000; 404: 565
- Porter EA, Weisblum B, Gellman SH. Mimicry of host-defense peptides by unnatural oligomers: Antimicrobial beta-peptides. J Am Chem Soc 2002; 124: 7324–7330
- Powers JP, Hancock RE. The relationship between peptide structure and antibacterial activity. Peptides 2003; 24: 1681–1691
- Raguse TL, Porter EA, Weisblum B, Gellman SH. Structure-activity studies of 14-helical antimicrobial beta-peptides: Probing the relationship between conformational stability and antimicrobial potency. J Am Chem Soc 2002; 124: 12774–12785
- Schmitt MA, Weisblum B, Gellman SH. Unexpected relationships between structure and function in alpha,beta-peptides: Antimicrobial foldamers with heterogeneous backbones. J Am Chem Soc 2004; 126: 6848–6849
- Sen A, Hui SW, Mosgroberanthony M, Holm BA, Egan EA. Localization of lipid exchange sites between bulk lung surfactants and surface monolayer – freeze-fracture study. J Colloid Interface Sci 1988; 126: 355–360
- Shai Y, Oren Z. Diastereoisomers of cytolysins, a novel class of potent antibacterial peptides. J Biol Chem 1996; 271: 7305–7308
- Siegel DP, Epand RM. Effect of influenza hemagglutinin fusion peptide on lamellar/inverted phase transitions in dipalmitoleoylphosphatidylethanolamine: implications for membrane fusion mechanisms. Biochim Biophys Acta 2000; 1468: 87–98
- Staudegger E, Prenner EJ, Kriechbaum M, Degovics G, Lewis RN, McElhaney RN, Lohner K. X-ray studies on the interaction of the antimicrobial peptide gramicidin S with microbial lipid extracts: Evidence for cubic phase formation. Biochim Biophys Acta 2000; 1468: 213–230
- Steiner H, Hultmark D, Engstrom A, Bennich H, Boman HG. Sequence and specificity of two antibacterial proteins involved in insect immunity. Nature 1981; 292: 246–248
- Tossi A, Sandri L, Giangaspero A. Amphipathic, alpha-helical antimicrobial peptides. Biopolymers 2000; 55: 4–30
- Wiese A, Gutsmann T, Seydel U. Towards antibacterial strategies: Studies on the mechanisms of interaction between antibacterial peptides and model membranes. J Endotoxin Res 2003; 9: 67–84
- Zasloff M. Magainins, a class of antimicrobial peptides from Xenopus skin: isolation, characterization of two active forms, and partial cDNA sequence of a precursor. Proc Natl Acad Sci USA 1987; 84: 5449–5453