Abstract
Measurements of contact-dependent fluorescence quenching and of fluorescence resonance energy transfer (FRET) within bilayers provide information concerning the spatial relationships between molecules on distance scales of a few nm or up a few tens of nm, respectively, and are therefore well suited to detect the presence and composition of membrane microdomains. As described in this review, techniques based on fluorescence quenching and FRET have been used to demonstrate the formation of nanoscale liquid-ordered domains in cholesterol-containing model membranes under physiological conditions, and to investigate the structural features of lipids and proteins that influence their partitioning between liquid-ordered and liquid-disordered domains. FRET-based methods have also been used to test for the presence of ‘raft’ microdomains in the plasma membranes of mammalian cells. We discuss the sometimes divergent findings of these studies, possible modifications to the ‘raft hypothesis’ suggested by studies using FRET and other techniques, and the further potential of FRET-based methods to test and to refine current models of the nature and organization of membrane microdomains.
Introduction
Fluorescence-based methods have played an important role in the elucidation of membrane structure, since they are sensitive, versatile and, importantly, well suited to probe both the dynamics of molecules and their organization on a variety of distance scales. Measurements of contact-dependent fluorescence quenching and of fluorescence resonance energy transfer (FRET) offer valuable means to investigate the spatial relationships between molecules on distances ranging from the sub-nanometre range to a few tens of nm, distance scales that are highly germane to monitor molecular interactions. In this review we will discuss how both types of measurements have been applied to investigate the existence and properties of microdomains in model and biological membranes, what such studies have revealed to date concerning the potential domain organization of membranes, and what these techniques can contribute to address the many questions that remain in this area. Complementary discussions of some of the issues touched upon in this review can be found in other articles in this issue, including those by Kabouridis and by Manes and Viola.
Basic characteristics of FRET and fluorescence-quenching measurements
In this review we will focus on two types of interactions that can occur between fluorescent molecules and nearby species within a membrane: contact-dependent quenching and resonance energy transfer. In the first, the fluorescent molecule in its excited state interacts through direct physical contact with a quencher species, usually a spin-labeled or brominated lipid, allowing the excited-state fluorophore to return to the ground state without emission of fluorescence. Fluorescence-quenching measurements thus yield information about the immediate environment of a bilayer-bound fluorescent molecule (1–2 ‘shells’ of lipid nearest neighbors). The efficiency of fluorescence quenching is measured experimentally as the extent of reduction in either the fluorescence intensity (quantum yield) or the fluorescence lifetime for the fluorescent species.
FRET, the transfer of energy from an excited-state donor fluorophore to a ground-state acceptor group, does not require direct contact between the donor and acceptor residues but varies in efficiency (ε) depending on the donor-acceptor distance according to the equation ε=(1/(1+(R/Ro)6)), where R is the donor-acceptor distance and Ro is the Förster radius. The value of Ro depends on both the relative orientation and the spectral properties of the donor and acceptor fluorophores. When the donor and the acceptor-labeled molecules undergo substantial and independent rotational motions, the orientation dependence is typically largely averaged (unless the transition dipoles for both species are highly constrained near-parallel to the membrane normal axis), and the value of Ro depends principally on the fluorophores’ spectral properties. Ro values are of the order of 4–6 nm for donor-acceptor pairs commonly used for hetero-FRET experiments (in which the donor and acceptor fluorophores are different species) and <5 nm for species used in homo-FRET experiments (in which the donor and acceptor fluorophores are the same species). By virtue of the strong distance dependence of FRET, energy-transfer measurements can detect nonrandomness in the distribution of acceptor- vis-à-vis donor-labeled molecules on length scales of the order of Ro, including formation of clusters in which the average distance between donor- and acceptor-labeled molecules is significantly less than 2Ro, or the presence of domains with dimensions larger than Ro in which donors are segregated from acceptors.
Several methods have been used to measure the efficiency of FRET (or any of a number of correlated variables, known as ‘FRET indices’) in studies of molecular distributions in membranes. The efficiency of hetero-FRET can be monitored by measuring donor fluorescence in the absence vs. the presence of the acceptor species, enhancement of the donor fluorescence after the acceptor species is selectively photobleached or fluorescence emitted by the acceptor species when the donor fluorophore is excited, known as ‘sensitized emission’. All such methods require a variety of corrections for spectral overlap, background and other factors (Berney & Danuser [Citation2003]). Homo-FRET has been monitored through energy transfer-dependent reductions in fluorescence anisotropy, determined using steady-state or time-resolved measurements (Varma & Mayor [Citation1998], Sharma et al. [Citation2004]). For this latter approach, extensive additional work must be carried out to identify specifically the component of anisotropy decay that arises from FRET.
For most (though not all) of the fluorescent probes commonly used in membrane research, the lifetime of the excited state is of the order of several nsec or less. As a result, fluorescence-quenching and FRET methods can detect even very short-lived interactions between different molecules, provided that at any given instant significant fractions of the fluorescent species examined are involved in such interactions, but can provide little information concerning the lifetimes of the interactions detected.
Fluorescence-quenching studies of domains in sterol-containing model membranes
Fluorescence-quenching studies are well suited to detect even small-scale inhomogeneities in bilayer lateral organization and have been used to investigate lipid-lipid, lipid-peptide and lipid-protein interactions in lipid bilayers. Early applications of this methodology included characterization of the lipid environments immediately adjacent to integral membrane proteins (East & Lee [Citation1982], London & Feigenson [Citation1981a]) and detection of solid-fluid phase separations in bilayers (Florine & Feigenson [Citation1987], Silvius [Citation1990]).
More recently, measurements of contact-dependent quenching have been used to test for inhomogeneities in lipid mixing in sterol-containing bilayers, using the approach illustrated schematically in . A series of lipid samples is prepared combining cholesterol with two (or potentially more) polar lipids, one of which is a fluorescence quencher, and a small amount (typically 0.1–1 mol%) of a fluorescent probe. The proportion of sterol is typically held constant among the different samples while the relative proportions of the quencher and non-quencher polar lipids are varied. If lipid mixing is homogeneous in all of the samples examined (A), the normalized fluorescence intensity (or lifetime) measured for the fluorescent species will fall in a smooth, monotonic manner as the mol fraction of the quencher lipid increases (B). If however even small segregated domains are present over a particular range of compositions sampled (C), within this range the quenching curve will deviate from that expected for homogeneous mixing, as illustrated in D. Since this approach uses samples containing relatively high molar proportions of the quencher species (typically ranging from 5–10 mol% up to 70 mol% or even higher), it is important to choose quencher lipids whose physical properties match as closely as possible those of the unlabeled lipids they are intended to model. To date, the requirement that substantial molar proportions of quencher be incorporated in the membranes examined has restricted the use of this method to model (lipid, lipid-peptide and potentially, reconstituted lipid-protein) systems.
Figure 1. Detection of inhomogeneities in lipid mixing by measuring contact-dependent fluorescence quenching. Bilayers are prepared from a mixture of sterol, an unlabeled phospho- or sphingolipid and (black circles) a spin-labeled or brominated quencher lipid, and incorporating a small amount of a fluorescent probe (grey circles). (A, B) If lipid mixing is random (panel A) throughout the range of compositions examined, a plot of the normalized fluorescence intensity vs. mol% quencher lipid is monophasic with a nearly exponential form (panel B). (C, D) By contrast, if for some compositions the lipid bilayer exhibits a markedly inhomogeneous distribution of lipids on a scale of a few nm or larger (panel C), the plot of normalized fluorescence vs. bilayer quencher content (panel D, solid curve) will deviate from the behavior expected for random mixing (dashed curve) over the range of compositions for which inhomogeneity is present. In the example shown the fluorescent species associates preferentially with domains enriched in the nonquenching major lipid species.
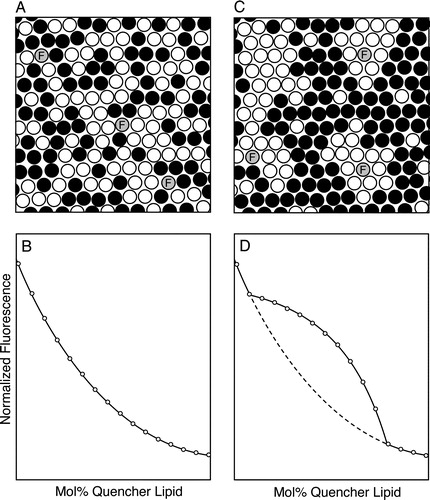
The approach just outlined was used to demonstrate that cholesterol can promote segregation of lipids in mixtures of dilauroyl and dipalmitoyl phosphatidylcholine (Silvius et al. [Citation1996]), and subsequently to demonstrate inhomogeneous lipid mixing at 37°C in bilayers combining physiological proportions of cholesterol, sphingolipids and a spin-labeled phosphatidylcholine (Ahmed et al. [Citation1997], De Almeida et al. [Citation2003]). The study of Ahmed et al. ([Citation1997]) provided the first concrete evidence that lipid mixtures with compositions resembling those of the plasma membrane outer leaflet could exhibit segregation of liquid-ordered (lo) and liquid-disordered (ld) domains at physiological temperatures. By contrast, using a similar approach it was found that cholesterol-containing lipid mixtures with compositions resembling those found in the cytoplasmic leaflet of the plasma membrane do not form segregated lipid domains at physiological temperatures (Wang & Silvius [Citation2001]).
London and colleagues used quenching methods to compare the abilities of different sterols to support formation of domains in sphingolipid/unsaturated phospholipid/sterol mixtures. They demonstrated that different sterols vary widely in their abilities to promote segregation of liquid-ordered lipid domains, and that some sterols can actually antagonize domain formation (Wang et al. [Citation2004], Xu et al. [Citation2001], Xu & London [Citation2000]). A fluorescence-quenching approach was also used to show that ceramide can displace cholesterol from lo domains (Megha & London [Citation2004], Wang et al. [Citation2004]).
London and Feigenson ([Citation1981b]) showed that by quantitatively analyzing the quenching curve for a fluorescent probe in a binary mixture of quencher and nonquencher lipids that form coexisting phases, the partition coefficient (Kp) describing the relative affinity of the probe for the two phases can be determined. Loura et al. ([Citation2001]) used a variation of this approach to examine the partitioning of fluorescent probes between coexisting ld and lo phases, in binary mixtures of dimyristoyl phosphatidylcholine and cholesterol. Such methods can be rigorously applied to ternary or higher-order systems only when extensive additional information is available concerning the systems’ phase diagrams. This information is only now becoming available but will facilitate accurate determination of the partition coefficients Kp(lo/ld) governing the distributions of fluorescent-labeled lipids, peptides or proteins between liquid-ordered and liquid-disordered lipid domains.
Silvius and colleagues (Wang et al. [Citation2000], [Citation2001], Wang & Silvius [Citation2000], [Citation2003]) have previously used a variation of the method of London and Feigenson ([Citation1981b]) to determine the relative affinities of different fluorescent lipids and lipid-modified peptides for lovs. ld domains in cholesterol-containing ternary lipid mixtures. The results obtained partially agreed with those obtained using a detergent-fractionation assay of the type frequently used to isolate ‘raft’ (detergent-resistant membrane [DRM]) fractions from membranes (London & Brown [Citation2000]) but also showed but that some bona fide constituents of these domains can be depleted from isolated DRM fractions. Koivusalo et al. ([Citation2004]) used this method to examine the partitioning of a variety of pyrene-labeled derivatives of phosphatidylcholine, sphingomyelin and galactocerebroside into lo domains in sphingomyelin/phosphatidylcholine/cholesterol bilayers.
London and colleagues (Fastenberg et al. [Citation2003], Shogomori et al. [Citation2005]) examined by fluorescence quenching the distribution of membrane-spanning α-helical peptides (a polyleucine-based sequence and the transmembrane sequence of the lymphocyte LAT protein) between ld and lo domains in cholesterol-containing lipid bilayers. They found that both types of bilayer-spanning sequences were strongly excluded from lo-domains, even when the LAT-derived peptide was S-acylated (palmitoylated) on two cysteine residues whose acylation is essential for raft association of LAT in the lymphocyte plasma membrane (Shogomori et al. [Citation2005]). Evidence from several approaches suggested that interactions between LAT and membrane lipids are insufficient to drive raft association of this protein, and that protein-protein interactions must also make important contributions.
FRET studies of fluid-fluid domain segregation in model membranes
Resonance energy transfer-based methods offer a potentially attractive complement to contact quenching-based techniques to study nanoscale domain formation in model and biological membranes, as FRET can provide information about molecular distributions on a distance scale (ca. 5–20 nm) that falls between those probed by simple fluorescence microscopy (>ca. 300 nm) and by contact-dependent quenching measurements (1–2 nm). This potential was first applied to lipid model systems to examine phase transitions in one- and two-component lipid bilayers (Leidy et al. [Citation2001], Pedersen et al. [Citation1996]).
Feigenson and Buboltz ([Citation2001]) used measurements of energy transfer for the donor-acceptor pair diO-C18:2/diI-C20:0 to test for the formation of domains in bilayers composed of dipalmitoyl phosphatidylcholine (DPPC), dilauroyl phosphatidylcholine (DLPC) and cholesterol. The authors reported FRET-based evidence for the formation of domains with dimensions of at least 5–10 nm in mixtures of these lipids for which domains were not observed by fluorescence microscopy. As expected, lateral inhomogeneity in lipid mixing was also detected by the same FRET approach in DPPC/DLPC/cholesterol mixtures that form domains large enough to be visualized by fluorescence microscopy. Similarly, using alternative FRET-based assays, Silvius ([Citation2003]) and De Almeida et al. ([Citation2005]) showed that bilayers combining sphingolipids (or long-chain saturated phospholipids), unsaturated phospholipids and cholesterol form segregated lo and ld domains at physiological temperatures and cholesterol contents, conditions under which such lipid mixtures do not form microscopically visible domains (Dietrich et al. [Citation2001], Veatch & Keller [Citation2003a], [Citation2003b]). Reassuringly, measurements of contact-dependent fluorescence quenching in similar lipid mixtures also provide evidence for laterally inhomogeneous lipid mixing under the same conditions (Ahmed et al. [Citation1997], Silvius et al. [Citation1996], Wang & Silvius [Citation2000], [Citation2003]). These observations, indicating that cholesterol-containing lipid mixtures can form segregated lo domains of nanoscopic dimensions, are of interest in the light of experimental evidence suggesting that rafts in biological membranes may exhibit dimensions on the order of tens of nanometers (Friedrichson & Kurzchalia [Citation1998], Pralle et al. [Citation2000], Sharma et al. [Citation2004], Varma & Mayor [Citation1998]).
Combining infrared-spectroscopic experiments with measurements of FRET between acyl chain-labeled fluorescent phospholipid derivatives, Redfern and Gericke ([Citation2004]; [Citation2005]) reported evidence that various physiological mono- and diphosphate derivatives of phosphatidylinositol tend to demix from phosphatidylcholine, even in fluid bilayers and at low mol fractions of the phosphoinositides. Interestingly, this tendency was manifested at physiological or higher but not at acidic pH. Given the great importance of phosphoinositides in intracellular signaling, further studies of their potential to cluster, particularly in lipid environments resembling those of the cytoplasmic leaflets of cellular membranes, will be of considerable interest.
FRET studies of domain organization in biological membranes
Basic considerations
To date FRET measurements have been used chiefly to examine the possible existence of microdomains within the plasma membrane, the membrane most accessible to manipulation and observation in intact cells. In order to determine whether an energy-transfer acceptor is nonrandomly distributed vis-à-vis a donor species on the cell surface, it is of course necessary to compare the experimental FRET signal to that expected if the acceptor distribution is truly random. The latter depends on the absolute (average) surface density of the acceptor species, the Förster radius Ro and the distance of closest possible approach of the donor and acceptor fluorophores (Dewey & Hammes [Citation1980], Wolber & Hudson [Citation1979]). Since for a given biological system these quantities are seldom all precisely known, assessments of possible molecular clustering or domain formation in biological membranes typically rest on analyses not of the absolute magnitude of the measured energy transfer, but rather of the qualitative manner in which the energy-transfer efficiency varies with the surface density of the acceptor species. For such analyses, the average FRET efficiency (or a related FRET index) and the average acceptor density (or acceptor fluorescence intensity) are measured for one or more selected regions on each of a number of cells and plotted to yield a graph that will hereafter be termed a ‘FRET efficiency profile’.
Theoretical FRET efficiency profiles are shown in A for cases where the distribution of acceptor molecules vis-à-vis donor molecules within the membrane is purely random and the acceptor and donor fluorophores can approach to different minimal distances Re (Dewey & Hammes [Citation1980], Wolber & Hudson [Citation1979]). The variation of FRET efficiency with acceptor density is nearly linear at low acceptor densities and extrapolates to a zero y-intercept. By contrast, in the extreme opposite case where the donor and acceptor species are entirely complexed or co-clustered even at low densities within the membrane, substantial energy transfer is measured even at very low acceptor densities. For simplicity of analysis, nonrandom distributions of fluorescent-labeled molecules within membranes are often modeled with a (constant) fraction of the donor and acceptor molecules clustered even at low surface densities and the remainder randomly distributed, giving a predicted FRET efficiency profile like the example shown in B.
Figure 2. FRET efficiency profiles for different possible distributions of fluorescent donor- and acceptor-labeled species in the membrane. The acceptor density is plotted as the average number of acceptor molecules per area element of size Ro2 (Ro=Förster radius). (A) Random distribution of donor and acceptor molecules within the membrane; the different curves are calculated for the indicated ratios of the distance of closest possible approach of donor and acceptor fluorophores (Re) to Ro, using equation [17] from Wolber and Hudson ([Citation1979]). (B) Thirty percent of the donor and acceptor molecules are co-clustered even at low concentrations in the membrane, while the remainder is randomly distributed in the membrane plane. The value of the y-intercept depends on the spacing of donor and acceptor species within clusters and does not provide an accurate estimate (though it may provide a lower bound) for the fraction of molecules clustered. The data scatter shown is representative of that generally observed in measurements of this type. (C) The donor and acceptor species are exclusively restricted to domains comprising 33% of the total membrane area. (D) The donor and acceptor species are 5-fold enriched in domains that comprise 20% of the total surface area of the membrane. In Panels C and D, the predicted FRET efficiency profiles (curves labeled ‘restricted’ and ‘enriched,’ respectively) are markedly different from the profiles predicted for a random distribution of donors and acceptors. However, unless both the x- and y-axis axis data can be accurately calibrated in terms of the absolute density of acceptor molecules in the membrane and the absolute FRET efficiency, respectively, the efficiency profiles can easily be fit within typical experimental error to the form expected for a random distribution (compare the ‘random’ curves to the ‘restricted (rescaled)’ or the ‘enriched (rescaled)’ curves in panels C and D, respectively).
![Figure 2. FRET efficiency profiles for different possible distributions of fluorescent donor- and acceptor-labeled species in the membrane. The acceptor density is plotted as the average number of acceptor molecules per area element of size Ro2 (Ro=Förster radius). (A) Random distribution of donor and acceptor molecules within the membrane; the different curves are calculated for the indicated ratios of the distance of closest possible approach of donor and acceptor fluorophores (Re) to Ro, using equation [17] from Wolber and Hudson ([Citation1979]). (B) Thirty percent of the donor and acceptor molecules are co-clustered even at low concentrations in the membrane, while the remainder is randomly distributed in the membrane plane. The value of the y-intercept depends on the spacing of donor and acceptor species within clusters and does not provide an accurate estimate (though it may provide a lower bound) for the fraction of molecules clustered. The data scatter shown is representative of that generally observed in measurements of this type. (C) The donor and acceptor species are exclusively restricted to domains comprising 33% of the total membrane area. (D) The donor and acceptor species are 5-fold enriched in domains that comprise 20% of the total surface area of the membrane. In Panels C and D, the predicted FRET efficiency profiles (curves labeled ‘restricted’ and ‘enriched,’ respectively) are markedly different from the profiles predicted for a random distribution of donors and acceptors. However, unless both the x- and y-axis axis data can be accurately calibrated in terms of the absolute density of acceptor molecules in the membrane and the absolute FRET efficiency, respectively, the efficiency profiles can easily be fit within typical experimental error to the form expected for a random distribution (compare the ‘random’ curves to the ‘restricted (rescaled)’ or the ‘enriched (rescaled)’ curves in panels C and D, respectively).](/cms/asset/3108ed47-8620-444e-adab-c2c3e946a7e2/imbc_a_147283_f0002_b.jpg)
Concentration-independent (complete or partial) clustering of membrane molecules of the idealized type just described can be readily deduced from experimental FRET efficiency profiles. However, other types of nonrandom distributions of acceptor vis-à-vis donor molecules can be more difficult to detect. FRET efficiency profiles predicted for two simple examples of such distributions are illustrated in C and 2D. In the first, donor and acceptor species are present exclusively in domains that comprise one-third of the total membrane area. In the second, donor and acceptor species both partition with a five-fold preference into domains that comprise 20% of the total membrane surface. In both of these examples the form of the FRET efficiency profile resembles that expected for a random donor-acceptor distribution, with a monophasic appearance and a zero y-intercept. When (as is typically the case) the absolute surface density of the acceptor species and/or the absolute efficiency of energy transfer cannot be precisely determined, and when the data moreover exhibit substantial y-axis scatter, such results could easily be erroneously interpreted as indicating a random distribution of acceptor vis-à-vis donor molecules within the membrane. With one exception (Glebov & Nichols [Citation2004]), most of the studies discussed below have utilized approaches more suitable to detect small domains or clusters, comprising a very small fraction of the total membrane area and within which energy-transfer donors and acceptors are highly concentrated, than to detect other types of inhomogeneities in membrane organization like those just noted.
FRET studies of microdomain organization in cell plasma membranes
An early FRET-based test for possible clustering of raft components in the cell plasma membrane was reported by Varma and Mayor ([Citation1998]), who measured the steady-state fluorescence anisotropy of a fluorescein-labeled folate, bound to a GPI-anchored (putatively raft-associated) vs. a transmembrane (putatively raft-excluded) form of the folate receptor on live CHO cells. For the transmembrane form the steady-state anisotropy decreased as the extent of surface labeling increased, in a manner consistent with a random distribution of molecules on the cell surface. By contrast, for the GPI-anchored form of the receptor the measured anisotropy was nearly constant, and lower in magnitude than that measured for the transmembrane-anchored receptor, over the range of surface densities examined. When cellular cholesterol was depleted, the anisotropy measured for fluorescent folate bound to the GPI-anchored form of the receptor became density-dependent, in a manner very similar to that observed for the transmembrane form. These findings were interpreted to suggest that the GPI-anchored form of the receptor exists in cholesterol-stabilized clusters within the plasma membrane. Based on the observation that the number of receptors per 1-µm2 pixel varied over a range of at least 200-fold, suggesting that an individual cluster could occupy an area as small as (1/200) µm2 or less, it was inferred that such clusters must be very small (dimensions of at most several tens of nm) (Varma & Mayor [Citation1998]).
Kenworthy and colleagues ([Citation2000]) examined energy transfer between Cy3- and Cy5-antibody-labeled antibodies or Fab fragments bound to GPI-anchored 5′-nucleotidase on the apical surface of fixed MDCK cells. Substantial density-dependent FRET was observed, which however varied in a manner consistent with that expected for a random distribution of acceptor- vis-à-vis donor-labeled molecules on the cell surface. From an error analysis of these results, the authors estimated that no more than ca. 20% of 5′-nucleotidase molecules on the cell surface could be present in clusters.
The divergent conclusions of the above studies (which as noted utilized different systems and methodologies) prompted further spectroscopic studies to resolve this discrepancy. De Angelis et al. ([Citation1998]), measuring proximity-dependent spectral interactions between molecules of GPI-anchored green fluorescent protein (GPI-GFP), obtained evidence that a small fraction of the GPI-GFP molecules exist in close proximity on the surface of living HeLa cells. A possible complication in the interpretation of this result is the potential of GFP and its variants to dimerize. While weak in solution, such dimerization can become significant when these proteins are anchored to a membrane (Glebov & Nichols [Citation2004], Zacharias et al. [Citation2002]), although this tendency appears weaker for GFP itself than for some of its color variants (Sharma et al. [Citation2004]). Kenworthy et al. ([Citation2004]) measured the efficiency of FRET between three different GPI-proteins and cholera toxin B-subunit (CTB) bound to ganglioside GM1 on the surface of several types of mammalian cells, both live and fixed. Strictly density-dependent energy transfer, suggesting an absence of clustering, was observed between Cy3- and Cy5-antibody-labeled molecules of the folate receptor, CD59 or 5′-nucleotidase, all GPI-anchored proteins and putative raft components, in nonpolarized HeLa, NRK and Fao cells. The same study also found no evidence for co-clustering of Cy3-antibody-labeled CD59 and Cy5-labeled CTB bound to ganglioside GM1 on these cells.
Nichols ([Citation2003]) used FRET measurements to examine the distributions on live COS-7 cells of GM1-bound CTB and of GPI-anchored GFP (GPI-GFP). Evidence was found for cholesterol-dependent clustering of GM1-bound CTB and for co-clustering of CTB with GPI-GFP, although GPI-GFP molecules showed a much weaker tendency to cluster with one another. A notable feature of this study was that it compared directly the efficiencies of energy transfer (at comparable acceptor densities) between CTB molecules, from CTB to transferrin receptor (Tf-R) molecules and between Tf-R molecules, finding markedly higher energy transfer between CTB molecules than for the other donor/acceptor combinations tested. These results suggested that GM1-bound CTB is enriched within membrane domains, tentatively identified with lipid rafts, from which Tf-R, a classical ‘non-raft’ marker, is excluded. In a subsequent study Glebov and Nichols ([Citation2004]), from measurements of energy transfer between GPI-anchored derivatives of dimerization-resistant CFP and YFP (mCFP, mYFP), concluded that at most a very small proportion (<10%) of these GPI-proteins are co-clustered in the plasma membranes of living Jurkat and COS-7 cells.
A possible model to reconcile most of the above results, as noted by Kenworthy et al. ([Citation2004]), is that a finite but small fraction of GPI-proteins and ganglioside GM1 may be clustered in mammalian cell membranes, and that different experimental approaches may differ in their ability to detect such minority populations of clustered molecules. This suggestion appears consistent with the findings of a recent study by Sharma et al. ([Citation2004]) who used fluorescence anisotropy measurements to monitor homo-FRET for GPI-anchored fluorescent proteins (GFP or mYFP) or the GPI-anchored folate receptor (labeled with a bound fluoresceinated folate) in several types of living mammalian cells. For all three types of GPI-anchored proteins the authors observed bi- or multiphasic fluorescence anisotropy decay curves, which included a rapid component identified by several criteria as arising from homo-FRET between labeled GPI-protein molecules. Interestingly, homo-FRET efficiency was independent of the surface density of the fluorescent GPI-protein examined but decreased as the level of expression of heterologous GPI-proteins increased. These and other findings suggested that GPI-proteins in the plasma membrane exist in part in ‘nanoclusters’ (estimated to comprise no more than 3-4 GPI-protein molecules) that can include different GPI-anchored species but do not obey a simple monomer-oligomer association equilibrium. Antibody-induced clustering of specific GPI-protein species led to their apparent depletion from nanoclusters, indicating that the latter are not entirely static. Formation of nanoclusters was cholesterol-dependent and influenced by membrane sphingolipid levels. From various data the authors estimated that the fraction of GPI-proteins present in clusters could be as low as 20% or as high as 40%, depending on the specific values used to estimate parameters not directly measurable. Like other workers, the authors found that measurements of hetero-FRET between GPI-anchored mCFP and mYFP did not provide clear evidence for co-clustering of these species on the cell surface. They suggested that combinatorial factors make homo-FRET measurements more sensitive to detect the presence of very small clusters (comprising only a few protein molecules) than are measurements of energy transfer between proteins labeled with different fluorophores.
A consensus picture of the organization of GPI-proteins on the cell surface may thus be emerging. A minority fraction of GPI-anchored proteins forms small clusters that comprise as few as 3–4 GPI-protein molecules and in which different GPI-protein species can be present. While the proportion of a given GPI-protein that is present in such clusters may obviously vary for different proteins, an average value from the results published to date may be of the order of 20%. The remaining, majority fraction of GPI-proteins could be distributed within the plasma membrane either in a purely random manner or, potentially (as noted earlier – see C,D), in an inhomogeneous manner that the FRET approaches most commonly employed are not well suited to detect. Glebov and Nichols ([Citation2004]) addressed this issue by comparing in live COS-7 and Jurkat cells the efficiency of energy transfer (at comparable acceptor densities) from GPI-anchored mCFP to either GPI-mYFP or an mYFP-labeled version of a transmembrane protein considered to be excluded from lipid rafts. Very similar FRET efficiency profiles were observed for the two donor-acceptor pairs, suggesting that the majority, unclustered populations of GPI-mCFP and –mYFP molecules on these cells are randomly distributed in the plasma membrane rather than enriched in possible raft (or other) domains.
To date the only systematic study reported using FRET to test for possible raft-related clustering of proteins at the inner face of the plasma membrane has been carried out by Zacharias et al. ([Citation2002]), who examined the efficiencies of energy transfer in living cells between several combinations of expressed lipid-anchored mCFP and mYFP protein constructs bound to this membrane surface. These workers reported that derivatives of mCFP and mYFP anchored to the plasma membrane inner leaflet via multiple saturated acyl chains showed evidence for clustering, which was abolished when the cells were treated with beta-methyl cyclodextrin to deplete cellular cholesterol. Evidence was also obtained for co-clustering of caveolin-CFP and acylated YFP, whereas geranylgeranylated derivatives of mCFP or mYFP did not appear to co-cluster with acylated mYFP or caveolin-CFP, respectively. Somewhat surprisingly, however, the authors reported evidence for co-clustering of geranylgeranylated forms of mCFP and mYFP under the same conditions, which in contrast to the clustering of acylated fluorescent proteins was not affected by cyclodextrin-mediated depletion of membrane cholesterol.
An(other) identity crisis for lipid rafts?
The question naturally arises how the FRET-based results discussed above can be integrated with findings from other methods concerning the organization of putatively raft-associated proteins in membranes, and into our evolving model of the possible nature of lipid microdomains. These considerations touch on three important current questions concerning the nature of lipid rafts. First, are rafts constitutively present at all in the plasma membrane? Second, do rafts exist in biological membranes as structures large enough to promote the interactions of diverse proteins in a functionally meaningful manner? Third, to what degree do protein-protein, as opposed to protein-lipid and lipid-lipid interactions, determine the formation and properties of the possibly diverse entities now referred to collectively as ‘rafts’?
The question whether lipid rafts exist as stable entities, particularly in resting cells, has been posed repeatedly in recent years (Glebov & Nichols [Citation2004], Kusumi et al. [Citation2004], Munro [Citation2003]). On this question the FRET findings reported to date must be considered equivocal. As discussed above, FRET evidence has been reported both for and against the hypothesis that GPI-proteins, ganglioside GM1 and multiply acylated inner-leaflet proteins in the plasma membrane, all regarded as classical raft markers by biochemical criteria, are clustered or highly concentrated in special microdomains. In interpreting these divergent reports, two points are useful to keep in mind. First, almost all existing FRET data suggest that for putative raft markers such as GPI-proteins, at best a minority of the total population of molecules is clustered, at least in resting cells. Second, as already noted most FRET studies to date have been designed and analyzed in a manner most suitable to detect tight clusters of labeled molecules. As discussed above, the experimental design applied by Glebov and Nichols to T-lymphocytes, comparing the efficiency of energy transfer from a putatively raft-associating donor species to putatively raft-associating vs. raft-excluded acceptor species, is better suited to test for other types of nonrandom distributions of raft components within cell membranes. It would be of great interest to apply this approach to other systems and membrane components. Further FRET studies could also be very useful to assess proposals that rafts may become stabilized and greatly expanded under certain conditions of cellular stimulation (Kusumi et al. [Citation2004], Subczynski & Kusumi [Citation2003]).
FRET studies to date have provided only limited information concerning the likely dimensions of lipid rafts, largely from the homo-FRET-based studies of Mayor and colleagues (Sharma et al. [Citation2004], Varma & Mayor [Citation1998]), who as already noted estimate that clusters of GPI-proteins in cell membranes may comprise on average as few as 3–4 GPI-protein molecules. The concept of a ‘nanoraft’ is supported by studies from the Kusumi laboratory, using single particle tracking analysis of GPI-linked CD59, that describe rafts in resting cells as small, unstable structures of only a few molecules with an average lifetime of less than 1 ms (Subczynski & Kusumi [Citation2003]). These estimates of size are roughly consistent with the dimensions (a few tens of nm) of clusters observed in immunogold-labeling EM studies of proteins involved in polyvalent IgE-initiated signaling in mast cell plasma membranes (Oliver et al. [Citation2004], Wilson et al. [Citation2004]) and of H-ras, K-ras and GPI-anchored GFP in BHK cells (Parton & Hancock [Citation2004], Prior et al. [Citation2001], [Citation2003]). Other methods have yielded similar estimates of the dimensions of raft domains in biological membranes (Friedrichson & Kurzchalia [Citation1998], Pralle et al. [Citation2000]), although one study has concluded that raft domains in myoblasts could be considerably larger (Schutz et al. [Citation2000]).
A final question that FRET-based findings can help to address concerning the possible domain organization of biological membranes is the composition of microdomains (including their possible heterogeneity) and the nature of the molecular interactions that generate and stabilize them. In this regard FRET, immunogold electron microscopy and single-molecule fluorescence microscopy offer potentially complementary approaches to correlate the spatial distributions of different components of cell membranes. In lymphocytes, FRET has failed to show evidence for coenrichment of different GPI-proteins in membrane domains in resting cells, or for accumulation of GPI-proteins or (CTB-bound) ganglioside GM1 in regions of the plasma membrane where T-cell receptor (TCR) molecules become activated through cell contact with anti-TCR-coated beads (Glebov & Nichols [Citation2004]). These findings agree well with those of single-molecule microscopy experiments (Bunnell et al. [Citation2002], Douglass & Vale [Citation2005]) that suggest that accumulation of downstream signaling molecules, including the raft-associating proteins LAT and Lck, at sites of TCR activation is driven by protein-protein interactions rather than by local accumulation or growth of lipid rafts. In mast cells, FRET results (Gidwani et al. [Citation2003]) have suggested that gangliosides GM1 and GD1b (bound to CTB and to anti-GD1b antibody, respectively) and the IgE receptor FcεRI are enriched in the vicinity of GPI-anchored Thy-1 molecules on the cell surface while the transferrin receptor (Tf-R) and CD48, both considered to be raft-excluded species, are not. In contrast, an immunogold EM study of the mast cell surface (Wilson et al. [Citation2004]) found that GM1 (complexed to CTB) and FcεRI codistributed only after the two species were (independently) clustered using antibodies, and that Thy-1 molecules, even when clustered with anti-Thy-1, did not co-cluster with either of these membrane components but did co-cluster with LAT, another putatively raft-associated protein.
The latter observations just discussed raise important questions as to whether ‘lipid rafts’ constitute domains in which a variety of molecular components with a common affinity for liquid-ordered lipid domains accumulate together, or whether subsets of molecules now considered as ‘raft’ components in fact form much more specific and diverse functional assemblies within the membrane. Formation of such assemblies could of course be driven by highly specific protein-protein interactions, as in the assembly of clusters of signaling and adaptor proteins observed upon activation of the T-cell receptor (Bunnell et al. [Citation2002], Douglass & Vale [Citation2005]), the galectin-1-dependent, cholesterol-independent clustering reported for activated H-ras (Prior et al. [Citation2003]) or the manifold protein-protein interactions reported to be mediated by the scaffolding domain of caveolin-1 (Liu et al. [Citation2002], Okamoto et al. [Citation1998]). However, more generic interactions, such as the interactions of galectins with glycoproteins and glycolipids that are postulated to generate or stabilize membrane domains (Braccia et al. [Citation2003], Brewer et al. [Citation2002], Delacour et al. [Citation2005], Partridge et al. [Citation2004], Sacchetini et al., [Citation2001]), could also play important roles in forming these structures.
Should the ‘classical’ concept of rafts be replaced by a picture of membrane microdomains as a many-faceted ensemble of more specific structures, whose generation requires critical contributions from protein-protein and protein-carbohydrate as well as protein-lipid and lipid-lipid interactions? Complex, combinatorial interactions of these types can of course easily be envisaged to give rise to specialized functional assemblies within liquid-ordered lipid domains. Microcompartmentation of this latter sort offers intrinsically faster and more efficient interactions between specific ‘raft-associated’ molecules than does random coenrichment of all ‘raft-associated’ molecules in very small (or larger, but highly abundant) domains within the plasma membrane. It also offers wider possibilities for regulation of domain assembly and stability, through modulation of any of a broad range of lipid-lipid, protein-lipid, protein-carbohydrate and protein-protein interactions, that may be in part responsible for discrepant data on the role of rafts (and caveolae) in cellular functions such as endocytosis and receptor signaling (Nabi & Le [Citation2003], Pike [Citation2004]). In this case, however, what special contributions can a liquid-ordered lipid environment bring to the function of such assemblies, and therefore to the function of the membrane as a whole? The answer may lie less in the ability of liquid-ordered domains to concentrate particular membrane components than in their ability to exclude others efficiently, as fluorescence-quenching and other studies of model membranes have demonstrated.
Models of rafts as families of more specific microdomains as discussed above are appealing, though by no means generally proven at present. Suitably designed FRET studies should be able to test such possibilities and to complement immunogold-labeling and single-molecule approaches in this respect, as FRET measurements can be carried out directly on living cells and rapidly provide data either for large or, in principle, very small populations of molecules. The distinctive potential of FRET measurements to provide population-based, real-time data concerning spatial relationships between membrane molecules may moreover prove invaluable to monitor changes in membrane domain organization during processes such as membrane trafficking or activation of cellular signaling at the plasma membrane. As FRET studies to date illustrate, new and highly sophisticated technologies (e.g., microscopy-based time-resolved anisotropy measurements) may be required to realize fully this potential. Ironically, one of the more challenging tasks for FRET as well as other approaches may be to determine whether a given functional domain is associated with liquid-ordered lipids. To this end entirely new classes of endogenous or exogenously incorporated membrane components may need to be identified as reliable and general markers for liquid-ordered regions of the membrane. Only when such issues are addressed may we may be properly able to define the place of ‘rafts’ in membrane function and organization.
This paper was first published online on prEview on 27 January 2006.
Research in the authors’ laboratories was supported by operating grants from the Canadian Institutes of Health Research (CIHR). I.R.N. is the recipient of a CIHR Investigator award.
References
- Ahmed SN, Brown DA, London E. On the origin of sphingolipid/cholesterol-rich detergent-insoluble cell membranes: physiological concentrations of cholesterol and sphingolipid induce formation of a detergent-insoluble, liquid-ordered lipid phase in model membranes. Biochemistry 1997; 36: 10944–10953
- Berney C, Danuser G. FRET or no FRET: a quantitative comparison. Biosphys J 2003; 84: 3992–4010
- Braccia A, Villani M, Immerdal L, Niels-Christiansen LL, Nystrom BT, Hansen GH, Danielsen EM. Microvillar membrane microdomains exist at physiological temperature. Role of galectin-4 as lipid raft stabilizer revealed by ‘superrafts’. J Biol Chem 2003; 278: 15679–15684
- Brewer CF, Miceli MC, Baum LG. Clusters, bundles, arrays and lattices: novel mechanisms for lectin-saccharide-mediated cellular interactions. Curr Opin Struct Biol 2002; 12: 616–623
- Bunnell SC, Hong DI, Kardon JR, Yamazaki T, McGlade CJ, Barr VA, Samelson LE. T cell receptor ligation induces the formation of dynamically regulated signaling assemblies. J Cell Biol 2002; 158: 1263–1275
- de Almeida RF, Fedorov A, Prieto M. Sphingomyelin/phosphatidylcholine/cholesterol phase diagram: boundaries and composition of lipid rafts. Biophys J 2003; 85: 2406–2416
- de Almeida RF, Loura LM, Fedorov A, Prieto M. Lipid rafts have different sizes depending on membrane composition: a time-resolved fluorescence resonance energy transfer study. J Mol Biol 2005; 346: 1109–1120
- De Angelis DA, Miesenbock G, Zemelman BV, Rothman JE. PRIM: proximity imaging of green fluorescent protein-tagged polypeptides. Proc Natl Acad Sci USA 1998; 95: 12312–12316
- Delacour, D, Gouyer, V, Zanetta, JP, Drobecq, H, Leteurtre, E, Grard, G, Moreau-Hannedouche, O, Maes, E, Pons, A, Andre, S, and others. 2005. Galectin-4 and sulfatides in apical membrane trafficking in enterocyte-like cells.J Cell Biol, 169:491–501.
- Dewey TG, Hammes GG. Calculation of fluorescence resonance energy transfer on surfaces. Biophys J 1980; 32: 1023–1035
- Dietrich C, Bagatolli LA, Volovyk ZN, Thompson NL, Levi M, Jacobson K, Gratton E. Lipid rafts reconstituted in model membranes. Biophys J 2001; 80: 1417–1428
- Douglass AD, Vale RD. Single-molecule microscopy reveals plasma membrane microdomains created by protein-protein networks that exclude or trap signaling molecules in T cells. Cell 2005; 121: 937–950
- East JM, Lee AG. Lipid selectivity of the calcium and magnesium ion dependent adenosinetriphosphatase, studied with fluorescence quenching by a brominated phospholipid. Biochemistry 1982; 21: 4144–4151
- Fastenberg ME, Shogomori H, Xu X, Brown DA, London E. Exclusion of a transmembrane-type peptide from ordered-lipid domains (rafts) detected by fluorescence quenching: extension of quenching analysis to account for the effects of domain size and domain boundaries. Biochemistry 2003; 42: 12376–12390
- Feigenson GW, Buboltz JT. Ternary phase diagram of dipalmitoyl-PC/dilauroyl-PC/cholesterol: nanoscopic domain formation driven by cholesterol. Biophys J 2001; 80: 2775–2788
- Florine KI, Feigenson GW. Influence of the calcium-induced gel phase on the behavior of small molecules in phosphatidylserine and phosphatidylserine-phosphatidylcholine multilamellar vesicles. Biochemistry 1987; 26: 1757–1768
- Friedrichson T, Kurzchalia TV. Microdomains of GPI-anchored proteins in living cells revealed by crosslinking. Nature 1998; 394: 802–805
- Gidwani A, Brown HA, Holowka D, Baird B. Disruption of lipid order by short-chain ceramides correlates with inhibition of phospholipase D and downstream signaling by FcepsilonRI. J Cell Sci 2003; 116: 3177–3187
- Glebov OO, Nichols BJ. Lipid raft proteins have a random distribution during localized activation of the T-cell receptor. Nat Cell Biol 2004; 6: 238–243
- Kenworthy AK, Nichols BJ, Remmert CL, Hendrix GM, Kumar M, Zimmerberg J, Lippincott-Schwartz J. Dynamics of putative raft-associated proteins at the cell surface. J Cell Biol 2004; 165: 735–746
- Kenworthy AK, Petranova N, Edidin M. High-resolution FRET microscopy of cholera toxin B-subunit and GPI-anchored proteins in cell plasma membranes. Molecular Biology of the Cell 2000; 11: 1645–1655
- Koivusalo M, Alvesalo J, Virtanen JA, Somerharju P. Partitioning of pyrene-labeled phospho- and sphingolipids between ordered and disordered bilayer domains. Biophys J 2004; 86: 923–935
- Kusumi A, Koyama-Honda I, Suzuki K. Molecular dynamics and interactions for creation of stimulation-induced stabilized rafts from small unstable steady-state rafts. Traffic 2004; 5: 213–230
- Leidy C, Wolkers WF, Jorgensen K, Mouritsen OG, Crowe JH. Lateral organization and domain formation in a two-component lipid membrane system. Biophys J 2001; 80: 1819–1828
- Liu P, Rudick M, Anderson RG. Multiple functions of caveolin-1. J Biol Chem 2002; 277: 41295–41298
- London E, Brown DA. Insolubility of lipids in Triton X-100: physical origin and relationship to sphingolipid/cholesterol membrane domains (rafts). Biochim Biophys Acta 2000; 1508: 182–195
- London E, Feigenson GW. Fluorescence quenching in model membranes. 2. Determination of local lipid environment of the calcium adenosinetriphosphatase from sarcoplasmic reticulum. Biochemistry 1981a; 20: 1939–1948
- London E, Feigenson GW. Fluorescence quenching in model membranes. 1. Characterization of quenching caused by a spin-labeled phospholipid. Biochemistry 1981b; 20: 1932–1938
- Loura LM, Fedorov A, Prieto M. Exclusion of a cholesterol analog from the cholesterol-rich phase in model membranes. Biochim Biophys Acta 2001; 1511: 236–243
- Megha, London E. Ceramide selectively displaces cholesterol from ordered lipid domains (rafts): implications for lipid raft structure and function. J Biol Chem 2004; 279: 9997–10004
- Munro S. Lipid rafts: elusive or illusive?. Cell 2003; 115: 377–88
- Nabi IR, Le PU. Caveolae/raft-dependent endocytosis. J Cell Biol 2003; 161: 673–677
- Nichols BJ. GM1-containing lipid rafts are depleted within clathrin-coated pits. Curr Biol 2003; 13: 686–690
- Okamoto T, Schlegel A, Scherer PE, Lisanti MP. Caveolins, a family of scaffolding proteins for organizing preassembled signaling complexes at the plasma membrane. J Biol Chem 1998; 273: 5419–5422
- Oliver, JM, Pfeiffer, JR, Surviladze, Z, Steinberg, SL, Leiderman, K, Sanders, ML, Wofsy, C, Zhang, J, Fan, H, Andrews, N, and others. 2004. Membrane receptor mapping: the membrane topography of Fc(epsilon)RI signaling. Subcell Biochem, 37:3–34.
- Parton RG, Hancock JF. Lipid rafts and plasma membrane microorganization: insights from Ras. Trends Cell Biol 2004; 14: 141–147
- Partridge EA, Le Roy C, Di Guglielmo GM, Pawling J, Cheung P, Granovsky M, Nabi IR, Wrana JL, Dennis JW. Regulation of cytokine receptors by Golgi N-glycan processing and endocytosis. Science 2004; 306: 120–124
- Pedersen S, Jorgensen K, Baekmark TR, Mouritsen OG. Indirect evidence for lipid-domain formation in the transition region of phospholipid bilayers by two-probe fluorescence energy transfer. Biophys J 1996; 71: 554–560
- Pike LJ. Lipid rafts: heterogeneity on the high seas. Biochem J 2004; 378: 281–292
- Pralle A, Keller P, Florin EL, Simons K, Horber JK. Sphingolipid-cholesterol rafts diffuse as small entities in the plasma membrane of mammalian cells. J Cell Biol 2000; 148: 997–1008
- Prior IA, Harding A, Yan J, Sluimer J, Parton RG, Hancock JF. GTP-dependent segregation of H-ras from lipid rafts is required for biological activity. Nat Cell Biol 2001; 3: 368–375
- Prior IA, Muncke C, Parton RG, Hancock JF. Direct visualization of Ras proteins in spatially distinct cell surface microdomains. J Cell Biol 2003; 160: 165–170
- Redfern DA, Gericke A. Domain formation in phosphatidylinositol monophosphate/phosphatidylcholine mixed vesicles. Biophys J 2004; 86: 2980–2992
- Redfern DA, Gericke A. pH-dependent domain formation in phosphatidylinositol polyphosphate/phosphatidylcholine mixed vesicles. J Lipid Res 2005; 46: 504–515
- Sacchettini JC, Baum LG, Brewer CF. Multivalent protein-carbohydrate interactions. A new paradigm for supermolecular assembly and signal transduction. Biochemistry 2001; 40: 3009–3015
- Schutt GJ, Kada G, Pastushenko VP, Schindler H. Properties of lipid microdomains in a mvscle cell membrane visualized by single molecule microscopy. EMBO J 2000; 19: 892–901
- Sharma P, Varma R, Sarasij RC, Ira, Gousset K, Krishnamoorthy G, Rao M, Mayor S. Nanoscale organization of multiple GPI-anchored proteins in living cell membranes. Cell 2004; 116: 577–589
- Shogomori H, Hammond AT, Ostermeyer-Fay AG, Barr DJ, Feigenson GW, London E, Brown DA. Palmitoylation and intracellular domain interactions both contribute to raft targeting of linker for activation of T cells. J Biol Chem 2005; 280: 18931–18942
- Silvius JR. Calcium-induced lipid phase separations and interactions of phosphatidylcholine/anionic phospholipid vesicles. Fluorescence studies using carbazole-labeled and brominated phospholipids. Biochemistry 1990; 29: 2930–2938
- Silvius JR. Fluorescence energy transfer reveals microdomain formation at physiological temperatures in lipid mixtures modeling the outer leaflet of the plasma membrane. Biophys J 2003; 85: 1034–1045
- Silvius JR, del Giudice D, Lafleur M. Cholesterol at different bilayer concentrations can promote or antagonize lateral segregation of phospholipids of differing acyl chain length. Biochemistry 1996; 35: 15198–15208
- Subczynski WK, Kusumi A. Dynamics of raft molecules in the cell and artificial membranes: approaches by pulse EPR spin labeling and single molecule optical microscopy. Biochim Biophys Acta 2003; 1610: 231–243
- Varma R, Mayor S. GPI-anchored proteins are organized in submicron domains at the cell surface. Nature 1998; 394: 798–801
- Veatch SL, Keller SL. A closer look at the canonical ‘Raft Mixture’ in model membrane studies. Biophys J 2003a; 84: 725–726
- Veatch SL, Keller SL. Separation of liquid phases in giant vesicles of ternary mixtures of phospholipids and cholesterol. Biophys J 2003b; 85: 3074–3083
- Wang J, Megha, London E. Relationship between sterol/steroid structure and participation in ordered lipid domains (lipid rafts): implications for lipid raft structure and function. Biochemistry 2004; 43: 1010–1018
- Wang TY, Leventis R, Silvius JR. Fluorescence-based evaluation of the partitioning of lipids and lipidated peptides into liquid-ordered lipid microdomains: a model for molecular partitioning into ‘lipid rafts’. Biophys J 2000; 79: 919–933
- Wang TY, Leventis R, Silvius JR. Partitioning of lipidated peptide sequences into liquid-ordered lipid domains in model and biological membranes. Biochemistry 2001; 40: 13031–13040
- Wang TY, Silvius JR. Different sphingolipids show differential partitioning into sphingolipid/cholesterol-rich domains in lipid bilayers. Biophys J 2000; 79: 1478–1489
- Wang TY, Silvius JR. Cholesterol does not induce segregation of liquid-ordered domains in bilayers modeling the inner leaflet of the plasma membrane. Biophys J 2001; 81: 2762–2773
- Wang TY, Silvius JR. Sphingolipid partitioning into ordered domains in cholesterol-free and cholesterol-containing lipid bilayers. Biophys J 2003; 84: 367–378
- Wilson BS, Steinberg SL, Liederman K, Pfeiffer JR, Surviladze Z, Zhang J, Samelson LE, Yang LH, Kotula PG, Oliver JM. Markers for detergent-resistant lipid rafts occupy distinct and dynamic domains in native membranes. Mol Biol Cell 2004; 15: 2580–2592
- Wolber PK, Hudson BS. An analytic solution to the Forster energy transfer problem in two dimensions. Biophys J 1979; 28: 197–210
- Xu X, Bittman R, Duportail G, Heissler D, Vilcheze C, London E. Effect of the structure of natural sterols and sphingolipids on the formation of ordered sphingolipid/sterol domains (rafts). Comparison of cholesterol to plant, fungal, and disease-associated sterols and comparison of sphingomyelin, cerebrosides, and ceramide. J Biol Chem 2001; 276: 33540–33546
- Xu X, London E. The effect of sterol structure on membrane lipid domains reveals how cholesterol can induce lipid domain formation. Biochemistry 2000; 39: 843–849
- Zacharias DA, Violin JD, Newton AC, Tsien RY. Partitioning of lipid-modified monomeric GFPs into membrane microdomains of live cells. Science 2002; 296: 913–916