Abstract
Infection of human erythrocytes by the malarial parasite, Plasmodium falciparum, results in complex membrane sorting and signaling events in the mature erythrocyte. These events appear to rely heavily on proteins resident in erythrocyte lipid rafts. Over the past five years, we and others have undertaken a comprehensive characterization of major proteins present in erythrocyte detergent-resistant membrane lipid rafts and determined which of these proteins traffic to the host-derived membrane that bounds the intraerythrocytic parasite. The data suggest that raft association is necessary but not sufficient for vacuolar recruitment, and that there is likely a mechanism of active uptake of a subset of erythrocyte detergent-resistant membrane proteins. Of the ten internalized proteins, few have been evaluated for a role in malarial entry. The β2-adrenergic receptor and heterotrimeric G protein Gs signaling pathway proteins regulate invasion. The implications of these differences are discussed. In addition, the latter finding indicates that erythrocytes possess important signaling pathways. These signaling cascades may have important influences on in vivo malarial infection, as well as on erythrocyte membrane flexibility and adhesiveness in sickle cell anemia. With respect to malarial infection, host signaling components alone are not sufficient to induce formation of the malarial vacuole. Parasite proteins are likely to have a major role in making the intraerythrocytic environment conducive for vacuole formation. Such interactions should be the focus of future efforts to understand malarial infection of erythrocytes since host- and parasite-targeted interventions are urgently needed to combat this terrible disease.
Malaria, blood stage infection and the involvement of lipid rafts
Malaria is a major world health problem Citation[1]. Plasmodium falciparum causes the most virulent form of human malaria. It kills over one million children annually, mostly in sub-Saharan Africa. In humans, the parasite infects the liver and mature red blood cells (). Intraerythrocytic infection is responsible for all of the symptoms and pathologies associated with malaria. Uncomplicated malaria is associated with cyclical fevers and chills. Fevers occur concurrently with the rupture of infected erythrocytes and release of parasite progeny. Chills are associated with intracellular parasite development in the erythrocyte. In infections of Plasmodium falciparum, the intracellular cycle lasts ∼48 hours, resulting in a fever once every two days. Severe malaria includes multiple pathological conditions such as lactic acidosis, cerebral malaria (resulting from adhesion of infected erythrocytes to the endothelium in the brain) and severe anemia (arising from clearance of both infected and uninfected erythrocytes, Citation[2]).
Figure 1. Asexual erythrocytic lifecycle of Plasmodium falciparum. Human malarial infection commences when an infected Anopheline mosquito bites a susceptible person. Transmitted sporozoites (not shown) spend the first 5–20 days replicating in the liver, without clinical symptoms. Liver-stage infection culminates with the production of thousands of merozoites that enter the bloodstream, where they rapidly adhere to and invade mature erythrocytes. The apical organelles (rhoptries shown) are involved in invasion and vacuole formation. Once inside, the intraerythrocytic lifecycle of Plasmodium falciparum is 48 hours long. Throughout this period, the growing parasite resides in a membranous sack, called the parasitophorous vacuolar membrane (PVM). Immediately following invasion, merozoites change into ring-shaped parasites during the first 24 hours of the lifecycle. From 24–36 hours post-invasion, trophozoite-stage parasites replicate their DNA and organelles and develop a tubovesicular transport network (TVN), which is used to move nutrients and wastes in and out of the cell. During the last 12 hours of the cycle, mature schizont-stage parasites segment into 16–32 daughter merozoites, rupture from the cell and subsequently infect additional erythrocytes. N = nucleus. This Figure is reproduced in color in Molecular Membrane Biology online.
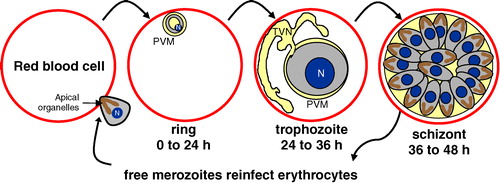
Parasite entry into erythrocytes is key to the establishment of blood stage infection. It is thus central to both acute and severe malaria. Entry is a complex and dynamic process Citation[3]. The invading merozoite-stage parasite contains specialized apical, secretory organelles called micronemes, rhoptries and dense granules (see ). With its apical end pointed at the erythrocyte, the merozoite interacts with the cell to form a parasite-host junction. Next, the erythrocyte bilayer invaginates, engulfing the parasite and forming the vacuolar membrane that surrounds the parasite throughout its development Citation[4]. There is cumulative evidence that merozoite entry involves parasite proteins that reside on the surface of the merozoite as well as its apical organelles and have the capacity to adhere to erythrocytes Citation[5–8]. Insights into host molecular determinants that regulate vacuole formation have come from recent studies on the identification and characterization of buoyant cholesterol-rich detergent-resistant membrane (DRM) complexes isolated from erythrocytes, suggestive of the presence of lipid rafts Citation[9–11] and raft-associated signaling pathways that are required during malarial infection Citation[12]. Moreover, some parasite ligands that reside in invasion-associated apical organelles adhere to erythrocytes and are also enriched in parasite DRM rafts that insert into the nascent vacuole, suggesting that they interact with host rafts there. This has led to a model that erythrocyte raft-associated signaling and parasite ligands act in conjunction to modulate host rafts, catalyzing endovacuolation of the erythrocytic membrane found in malarial infection.
Erythrocyte rafts: What and where are they?
The mature human erythrocyte is a terminally-differentiated cell. It lacks a nucleus, other intracellular organelles, de novo protein and lipid biosynthesis and endocytic membrane turnover Citation[13], Citation[14]. The organization of the red cell reflects its functions of delivering oxygen to tissues and surviving repeated passage through capillaries. This explains the presence of high concentrations of cytoplasmic hemoglobin, prominent solute and ion transport systems and a deformable sub-membrane cytoskeleton, whose functions in the erythrocyte have been elucidated by numerous, detailed studies over many years. More recent studies suggest that erythrocytes contain DRM rafts whose functions are only emerging. Rafts are thought to constitute floating ‘islands’ of proteins and lipids held together by the cholesterol-rich microenvironment Citation[15–18]. Many (if not most) raft proteins can be isolated as DRMs. Since DRMs are highly buoyant and cholesterol-rich, they can be separated from other detergent-resistant cytoskeletal components by density gradient centrifugation. Although it has been questioned whether DRMs are artifactual, multiple studies (summarized by Citation[19]) demonstrate that DRMs do contain aggregated rafts. In addition, non-raft proteins and lipids are significantly depleted from isolated DRMs that, like rafts, are enriched in cholesterol Citation[20]. Constituent components of cholesterol-dependent DRMs are thus likely to possess properties that reflect specialized requirements for association with the cholesterol-rich raft environment in cellular membranes.
Studies over the last five years have established that DRM raft complexes can be isolated from uninfected erythrocyte membranes. A first round of major and minor erythrocyte DRM raft components was delineated by antigenic and structural methods by several laboratories Citation[10], Citation[21–24]. The identified DRM proteins are involved in diverse cellular functions such as complement regulation (i.e., CD55, CD59) and water transport (i.e., aquaporin-1). They include major integral membrane proteins such as band 3, but not others such as glycophorin A. Examinations of individual proteins found in DRMs suggest their uniform distribution over the erythrocyte membrane at a ‘macro’ level. Direct biophysical evidence for the organization and function of raft resident proteins in ‘micro’ lateral heterogeneities in the erythrocyte bilayer is still lacking. However, many red cell DRM raft proteins are clearly enriched in the parasitophorous vacuolar membrane (PVM) formed by the malaria parasite as it enters the erythrocyte (). Non-DRM proteins of the host membrane are not internalized to the PVM. Further, only a subset of DRM raft proteins access the vacuole, and this has led to the suggestion that host raft protein uptake is not due to diffusion but is a consequence of one or more active signaling mechanisms Citation[11].
Figure 2. Selective uptake of a subset of erythrocyte DRM proteins into the PVM. Immunofluorescence assays demonstrated that some DRM proteins (i.e., flotillin-1) are strongly recruited to the vacuolar membrane, whereas other DRM proteins (i.e., stomatin/band 7) are excluded from the PVM. The integral membrane protein, band 3, which is a major constituent of erythrocyte DRMs, does not enter the PVM. Further, non-DRM proteins do not traffic to the PVM (i.e., CD47). Cumulatively, the data suggests that malarial invasion and vacuole formation involves an active mechanism to sort host raft proteins that enter the vacuolar membrane. The nuclei were visualized by Hoechst DNA staining. Diameter of erythrocytes ∼7 µm. Adapted from Murphy and Samuel Citation[11] with permission from Blood. This Figure is reproduced in color in Molecular Membrane Biology online.
![Figure 2. Selective uptake of a subset of erythrocyte DRM proteins into the PVM. Immunofluorescence assays demonstrated that some DRM proteins (i.e., flotillin-1) are strongly recruited to the vacuolar membrane, whereas other DRM proteins (i.e., stomatin/band 7) are excluded from the PVM. The integral membrane protein, band 3, which is a major constituent of erythrocyte DRMs, does not enter the PVM. Further, non-DRM proteins do not traffic to the PVM (i.e., CD47). Cumulatively, the data suggests that malarial invasion and vacuole formation involves an active mechanism to sort host raft proteins that enter the vacuolar membrane. The nuclei were visualized by Hoechst DNA staining. Diameter of erythrocytes ∼7 µm. Adapted from Murphy and Samuel Citation[11] with permission from Blood. This Figure is reproduced in color in Molecular Membrane Biology online.](/cms/asset/d4102eca-c1fb-4104-b13e-04036b0321ef/imbc_a_147327_f0002_b.jpg)
There have been difficulties defining what constitutes a resident DRM protein. This is largely due to the fact that erythrocyte DRM protein and lipid composition is highly dependent on the ratio of detergent to protein used in the extractions Citation[11], Citation[22], Citation[25]. Thus, distinct compositions have been described for erythrocyte DRM complexes extracted under different conditions Citation[10], Citation[11], Citation[21–23], Citation[26], although all DRMs are identified by high cholesterol content and buoyancy in a sucrose gradient Citation[19]. As suggested by a recent comparative study Citation[11], in general, high protein concentrations (7.5 mg protein/ml vs. 1.25 mg/ml) in the extractions, results in isolation of protein-rich complexes that include components of the erythrocyte skeleton. Further, depletion of cholesterol does not induce disruption of such complexes Citation[10], Citation[27]. In contrast, lowering the protein concentration results in isolation of protein-poor complexes that lack skeletal components. Detergent concentration also profoundly affects DRM composition. Low detergent concentration (i.e., 0.5% Triton X-100) increases the total bulk of protein recruited to the erythrocyte DRM (including skeletal proteins), whereas high concentration solubilizes membranes completely (i.e., 2% Triton X-100). At 1% Triton X-100, protein-poor, cholesterol-rich complexes are isolated free of cytoskeletal components. Since the higher protein mass extracted in 0.5% Triton X-100 showed the same cholesterol-to-protein ratio of that found by extraction at 1%, we hypothesized that membrane lipid interactions other than those mediated by cholesterol (i.e., non-raft interactions) are not disrupted by low (i.e., 0.5%) levels of Triton X-100 Citation[11].
Our studies on erythrocyte DRM rafts and malarial infection have focused on these protein-poor complexes for two reasons. First, erythrocyte DRM complexes have provided a rich vein for identifying at least 14 erythrocyte proteins recruited to the malarial vacuole (ten of which are summarized in Citation[11]). Previous studies often failed to detect major host proteins in the malarial vacuole. This makes sense when you realize that erythrocyte DRMs contain no more than a few percent of the total mass of red cell membrane proteins Citation[10], Citation[11]. Second, mild cholesterol depletion of erythrocytes blocks malarial infection and dissipates erythrocyte DRMs Citation[10]. This provides a functional link between conditions required for infection and those associated with DRM complex formation.
Thus, the working model for erythrocyte rafts and malarial infection is based on studies of protein-poor, lipid-rich DRMs of erythrocytes whose formation is critically dependent on cholesterol. At least 19 erythrocyte proteins associate with DRMs (; from Murphy and SamuelCitation[11]). P. falciparum entry results in uptake of at least 10 of these proteins (; from Murphy and SamuelCitation[11]). Of these, proteins that strongly partition into erythrocyte DRMs also appear to traffic to the PVM (i.e., flotillin-1/-2, minor proteins). In contrast, the bulk mass of erythrocyte DRM proteins (i.e., band 3 and stomatin/band 7) and all non-DRM proteins are excluded from the PVM. The model indicates that residence in a DRM is necessary but not sufficient for recruitment of a host protein to the PVM. Thus, there must be active mechanisms in place to sort and traffic select erythrocyte DRM raft proteins away from other DRM proteins and abundant non-DRM host proteins.
Figure 3. Model of erythrocyte DRM rafts and their enrichment in the malarial vacuolar membrane. The uninfected erythrocyte membrane contains a variety of generalized lipid domains (grey spheres) and raft microdomains (pink spheres) containing various proteins. Some proteins partition mostly into DRM raft domains (i.e., flotillins), while others are only minimally present there (i.e., band 3). During malaria infection, merozoite-stage parasites invade erythrocytes to reside in a membrane-bound parasitophorous vacuole. The PVM becomes selectively cholesterol-enriched, and ten of the known raft proteins are internalized to the PVM (flotillin-1 and -2, Gs, β2AR, AQP1, Duffy, CD55, CD58, CD59, scramblase). Major integral membrane proteins are not internalized to the PVM (i.e., glycophorins A and C, cytoskeleton-associated band 3, etc.). The lower left inset shows the perspective of the model, depicting a single infected erythrocyte with a magnified view of the plasma membrane and PVM. Since the PVM is formed by invagination of the plasma membrane, proteins that are cytoplasmically-oriented in uninfected cells remain so upon infection; protein structures exposed to the extracellular space face the vacuolar space upon infection. 4.1 indicates protein 4.1.
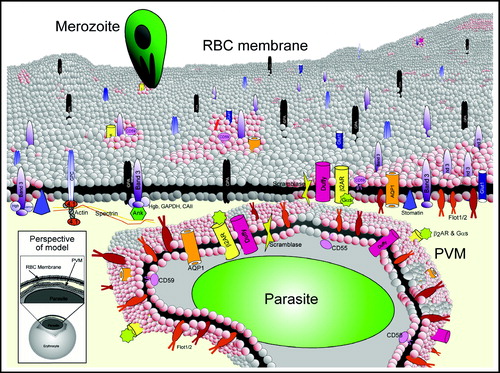
Table I. Summary of erythrocyte lipid raft proteins.
Functional consequences of internalization of host raft components in malarial infection
Malarial infection results in uptake of a subset of erythrocyte DRM proteins. This uptake is likely to have some consequence for malarial invasion, growth and/or egress from the infected erythrocyte. The importance of internalized proteins for malarial infection has been assessed for only a few of the fourteen internalized proteins. Three glycosylphosphatidylinositol (GPI)-anchored proteins (CD55, CD58 and CD59) are internalized host proteins associated with regulation of complement. Despite the importance of these proteins in vivo (for complement regulation), these GPI-anchored proteins appear to be dispensable for P. falciparum infection in vitroCitation[10]. This finding was demonstrated using blood from patients with paroxysmal nocturnal hemoglobinuria (PNH), which is a rare disorder resulting from a somatic mutation in the phosphatidylinositol glycan class A gene, leading to partial or complete absence of GPI-anchored proteins on the cell surface Citation[28]. Although ‘severe’ phenotype PNH erythrocytes contained higher total cholesterol than normal erythrocytes, this increase did not appear to stimulate recruitment of proteins into erythrocyte DRMs. Moreover, the absence of GPI-anchored proteins had no effect on malarial invasion or trafficking of DRM raft markers to the PVM Citation[10]. However, although a functional role for these GPI-anchored proteins cannot be established based on the in vitro studies to date, we cannot rule out a function for GPI-anchored protein uptake during in vivo infection. Recent studies in rodent malarial infections suggest that GPI-anchored proteins may provide a receptor for entry, but there is no corresponding evidence in human malarias Citation[29]
The uptake of the seven remaining internalized erythrocyte DRM proteins and the importance of each for malarial growth have not been examined thoroughly. However, a critical connection has been established between malarial invasion and signaling via the host β2-adrenoreceptor (β2AR) and the heterotrimeric G protein Gs (Citation[12]; see below). Studies are needed to further understand this connection as well as the role of the flotillins, aquaporin-1, the Duffy chemokine receptor and scramblase in malarial infection. A recent report showed that once inside the erythrocyte, the malarial parasite alters the DRM association of raft-associated proteins such as flotillin-1 and -2, an outcome that may reflect a novel parasite mechanism for remodeling the infected erythrocyte membrane Citation[30].
Erythrocyte signaling and malarial infection
The recent explosive interest in DRM rafts has been due to findings that they contain heterotrimeric G proteins and tyrosine kinases on their cytoplasmic face; these proteins associate with rafts because they tend to be acylated Citation[31], Citation[32]. Although erythrocytes are not highly active in signaling, they do possess signaling molecules Citation[33], including heterotrimeric G alpha proteins, Gs, Gi/oCitation[34], GqCitation[35], GzCitation[36] and G11Citation[37], Citation[38]. Gs is recruited to the malarial vacuole, and peptides that disrupt its interaction with associated receptors block malarial infection Citation[10], Citation[12], Citation[23]. Further, agonists of the β2AR stimulate production of cyclic adenosine monophosphate (cAMP) and increase malarial infection; both effects are blocked by antagonists. Thus, erythrocyte signaling via adrenergic receptors and Gs plays an important role in regulating malarial infection.
Adrenergic receptors are expressed on virtually every cell type. It is well established that expression and function of receptors and physiological responses to agonists and antagonists display marked inter-individual variation within human populations. There are nine human adrenergic receptor types: α1A,1B,1D; α2A-C and β1-3, with polymorphisms in many of these sub-types Citation[39]. Since β2ARs exist on erythroid cells, including mature erythrocytes Citation[40], at a minimum, it is possible that polymorphisms in the β2AR, especially those functionally linked to signaling, may play a significant role in modulating malarial infection. However, reduced β2AR-mediated signaling may be compensated for by other GPCRs, such as the adenosine receptor that also activates Gs and regulates P. falciparum entry into erythrocytes Citation[12].
In addition to malarial invasion, Gs signaling modulates other properties of erythrocytes as well. Activation of the pathway through the β2AR alters erythrocyte membrane rigidity Citation[40–46]. Exposure to epinephrine increases red cell filterability Citation[41] and increases cell membrane flexibility Citation[40]. In addition to flexibility, Gs signaling influences the adhesive properties of erythrocytes in sickle cell disease. Treatment of erythrocytes with forskolin or epinephrine caused increased adhesion of sickle erythrocytes (but not of normal erythrocytes) to immobilized laminin via erythrocyte BCAM/LU Citation[47] and to endothelial α5β3 integrin via erythrocyte ICAM-4/LW Citation[48]. Such adhesive events could possibly contribute to the vaso-occlusive crises observed in sickle cell anemia patients. The increased adhesion between BCAM/LU and laminin has since been shown to occur through Epac, a cAMP-dependent exchange factor that activates Rap1, a small GTPase in red cells Citation[49]. Thus, in addition to a role for the Gs signaling pathway in malarial invasion, this pathway can modulate membrane flexibility and adhesiveness in the erythrocyte. The dependence of these functions on erythrocyte rafts is unknown. Nonetheless, as in signaling at the ‘immunological synapse’ Citation[50], Citation[51], erythrocyte rafts may provide critical foci for rapid, transient changes in the organization of critical host molecules. Given their importance, raft proteins may be exploited in infections as well as genetic disorders.
Parasite DRM rafts: Coming together with the host during malarial invasion?
Although catecholamines (released during a fever) activate the β-adrenoreceptor to stimulate an increase in cAMP in erythrocytes, this alone does not induce endovacuolar uptake or clustering of rafts in the erythrocyte membrane (Murphy and Haldar, unpublished). Vacuole formation requires a stimulus from the parasite, and our working model proposes that parasite proteins couple directly to erythrocyte Gs or to Gs-containing host raft complexes that are internalized into the vacuole (see below). In support of this model, we characterized a P. falciparum orthologue of stomatin (Pfstomatin), an important raft family protein in eukaryotes Citation[52]. Stomatins are members of a protein superfamily that contains flotillin-1, whose ability to associate with DRM rafts and oligomerize, is thought to induce raft nucleation and formation of DRM-based membrane invaginations Citation[53], Citation[54]. Several lines of evidence suggest that Pfstomatin and RhopH (another parasite-encoded protein) are involved in raft-based processes that could affect invasion. These proteins reside in parasite rhoptries (apical organelles that discharge their contents during erythrocyte invasion), are parasite DRM raft proteins, form oligomers, adhere to erythrocytes, insert into the newly forming Gs (and host raft)-enriched vacuole and end up on the cytoplasmic face of the vacuolar membrane Citation[52]. Thus, they have the potential to nucleate both parasite and host rafts, as well as DRM-based membrane invaginations. Since rafts show self-associative properties and form lipid shells (or platforms) that signal Citation[55], we think it is possible that interactions of parasite rafts and host rafts (containing Gs) influence signaling, at least to the extent of concentrating signaling at the invagination junction, where the nascent vacuole is forming (see model in ). As described above, catecholamines (which are high during malaria fever periods, the time of parasite emergence and reinvasion into red cells) stimulate cAMP production and alter red cell rigidity, filterability and deformation Citation[40], Citation[41], Citation[56]. In vivo, adrenergic signaling processes are thought to be rapidly attenuated. Thus, parasite proteins released during early infection may activate, prolong and/or alter host signaling pathways, and in doing so, render the erythrocyte more permissive for malarial infection.
Figure 4. Proposed model for recruitment of parasite and host DRM rafts during PVM formation. The PVM contains proteins originating from erythrocyte and parasite DRMs. The model shows a nascent vacuole (blue circle) where proteins from parasite DRM rafts (colored dots) nucleate host DRM rafts that contain Gs (blue bar). These host-parasite complexes are sequestered in the PVM, where they are located on the cytoplasmic face of the vacuolar membrane. These complexes may be involved in the raft-induced invagination of the host plasma membrane required for PVM formation. PPM indicates parasite plasma membrane. Reproduced from Hiller and colleagues Citation[52].
![Figure 4. Proposed model for recruitment of parasite and host DRM rafts during PVM formation. The PVM contains proteins originating from erythrocyte and parasite DRMs. The model shows a nascent vacuole (blue circle) where proteins from parasite DRM rafts (colored dots) nucleate host DRM rafts that contain Gs (blue bar). These host-parasite complexes are sequestered in the PVM, where they are located on the cytoplasmic face of the vacuolar membrane. These complexes may be involved in the raft-induced invagination of the host plasma membrane required for PVM formation. PPM indicates parasite plasma membrane. Reproduced from Hiller and colleagues Citation[52].](/cms/asset/4f004a00-17c9-48dd-ae0d-7eab81512752/imbc_a_147327_f0004_b.jpg)
Future directions
That erythrocyte GPCRs and G proteins regulate plasmodial infection was an unexpected finding. It has led to development of concepts of how G protein signaling and parasite ligands regulate dynamic changes in the erythrocyte to modulate malarial entry and host membrane changes during blood stage infection. However, comprehensive studies of raft signaling pathways in the erythrocyte still need to be undertaken. G protein signaling has been shown to regulate reorganization of the cytoskeleton in a variety of cells Citation[57], Citation[58]. Of particular interest will be determining how signaling in the erythrocyte is linked to reorganization of the erythrocyte cytoskeleton – this could affect many erythrocyte membrane processes, including deformation and invagination to form the vacuole during parasite entry, as well as erythrocyte adhesion. Identification of additional Gs-coupled receptors and other raft proteins that regulate signaling and erythrocyte infection by P. falciparum may provide targets to develop new strategies to dampen host signaling and thereby protect against malarial infection. In addition, parasite ligands that interact with host raft signaling pathways may also provide new targets for drug and vaccine development.
This paper was first published online on prEview on 24 January 2006.
This work was supported by American Heart Association Pre-doctoral Fellowships (0315210Z to S.M., 0215246Z to N.L.H.), a Northwestern University Presidential Fellowship (S.M.) and N.I.H. grants (P01 HL078826 to K.H., J.W.L and N.M.; AI39071 and HL079397 to K.H.; HL55591 and HL03961 to J.W.L.; DK32094 to N.M.).
References
- Miller LH, Baruch DI, Marsh K, Doumbo OK. The pathogenic basis of malaria. Nature 2002; 415: 673–679
- Weatherall, DJ, Miller, LH, Baruch, DI, Marsh, K, Doumbo, OK, Casals-Pascual, C, Roberts, DJ. Malaria and the red cell, Hematology, (Am Soc Hematol Educ Program). 2002;35–57.
- Dvorak JA, Miller LH, Whitehouse WC, Shiroshi T. Invasion of erythrocytes by malaria merozoites. Science 1975; 187: 748–750
- Bannister LH, Hopkins JM, Fowler RE, Krishna S, Mitchell GH. A brief illustrated guide to the ultrastructure of Plasmodium falciparum asexual blood stages. Parasitol Today 2000; 16: 427–433
- Holder AA, Blackman MJ, Borre M, Burghaus PA, Chappel JA, Keen JK, Ling IT, Ogun SA, Owen CA, Sinha KA. Malaria parasites and erythrocyte invasion. Biochem Soc Trans 1994; 22: 291–295
- Cowman AF, Crabb B. The Plasmodium falciparum genome: a blueprint for erythrocyte adhesion. Science 2002; 298: 126–128
- Chitnis CE. Molecular insights into receptors used by malaria parasites for erythrocyte invasion. Curr Opin Hematol 2001; 8: 85–91
- Sim BK, Chitnis CE, Wasniowska K, Hadley TJ, Miller LH. Receptor and ligand domains for invasion of erythrocytes by Plasmodium falciparum. Science 1994; 264: 1941–1944
- Lauer SA, Chatterjee S, Haldar K. Uptake and hydrolysis of sphingomyelin analogues in Plasmodium falciparum-infected red cells. Mol Biochem Parasitol 2001; 115: 275–281
- Samuel BU, Mohandas N, Harrison T, McManus H, Rosse W, Reid M, Haldar K. The role of cholesterol and glycosylphosphatidylinositol-anchored proteins of erythrocyte rafts in regulating raft protein content and malarial infection. J Biol Chem 2001; 276: 29319–29329
- Murphy SC, Samuel BU, Harrison T, Speicher KD, Speicher DW, Reid ME, Prohaska R, Low PS, Tanner MJ, Mohandas N, Haldar K. Erythrocyte detergent-resistant membrane proteins: their characterization and selective uptake during malarial infection. Blood 2004; 103: 1920–1928
- Harrison T, Samuel BU, Akompong T, Hamm H, Narla M, Lomasney JW, Haldar K. Erythrocyte G protein coupled receptor signaling in malaria infection. Science 2003; 301: 1734–1736
- Chasis JA, Prenant M, Leung A, Mohandas N. Membrane assembly and remodelling during reticulocyte maturation. Blood 1989; 74: 1112–1120
- Schrier SL. Red cell membrane biology – introduction. Clin Haematol 1985; 14: 1–12
- Brown D, London E. Functions of lipid rafts in biological membranes. Ann Rev Cell Dev Biol 1998; 14: 111–136
- Friedrichson T, Kurzchalia TV. Microdomains of GPI-anchored proteins in living cells revealed by crosslinking. Nature 1998; 394: 802–804
- Simons K, Ikonen E. Functional rafts in cell membranes. Nature 1997; 387: 569–572
- Varma R, Mayor S. GPI-anchored proteins are organized in sub micron domains at the cell surface. Nature 1998; 394: 798–801
- Brown DA, London E. Structure and origin of ordered lipid domains in biological membranes. J Membr Biol 1998; 164: 103–114
- Ahmed SN, Brown DA, London E. On the origin of sphingolipid/cholesterol-rich detergent-insoluble cell membranes: physiological concentrations of cholesterol and sphingolipid induce formation of a detergent-insoluble, liquid-ordered lipid phase in model membranes. Biochemistry 1997; 36: 10944–10953
- Salzer U, Hinterdorfer P, Hunger U, Borken C, Prohaska R. Ca(+ + )-dependent vesicle release from erythrocytes involves stomatin-specific lipid rafts, synexin (annexin VII), and sorcin. Blood 2002; 99: 2569–2577
- Salzer U, Prohaska R. Stomatin, flotillin-1, and flotillin-2 are major integral proteins of erythrocyte lipid rafts. Blood 2001; 97: 1141–1143
- Lauer S, VanWye J, Harrison T, McManus H, Samuel BU, Hiller NL, Mohandas N, Haldar K. Vacuolar uptake of host components, and a role for cholesterol and sphingomyelin in malarial infection. Embo J 2000; 19: 3556–3564
- Civenni G, Test ST, Brodbeck U, Butikofer P. In vitro incorporation of GPI-anchored proteins into human erythrocytes and their fate in the membrane. Blood 1998; 91: 1784–1792
- Minetti, G, Ciana, A. New and old integral proteins of the human erythrocyte membrane. Blood 2003;101: 3751; author reply, 3751–3753.
- Ciana A, Balduini C, Minetti G. Detergent-resistant membranes in human erythrocytes and their connection to the membrane-skeleton. J Biosci 2005; 30: 317–328
- Rivas MG, Gennaro AM. Detergent resistant domains in erythrocyte membranes survive after cell cholesterol depletion: an EPR spin label study. Chem Phys Lipids 2003; 122: 165–169
- Rosse WF. Paroxysmal nocturnal hemoglobinuria as a molecular disease. Medicine 1997; 76: 63–93
- Rungruang T, Kaneko O, Murakami Y, Tsuboi T, Hamamoto H, Akimitsu N, Sekimizu K, Kinoshita T, Torii M. Erythrocyte surface glycosylphosphatidyl inositol anchored receptor for the malaria parasite. Mol Biochem Parasitol 2005; 140: 13–21
- Nagao E, Seydel KB, Dvorak JA. Detergent-resistant erythrocyte membrane rafts are modified by a Plasmodium falciparum infection. Exp Parasitol 2002; 102: 57–59
- Moffett S, Brown DA, Linder ME. Lipid-dependent targeting of G proteins into rafts. J Biol Chem 2000; 275: 2191–2198
- Sargiacomo M, Sudol M, Tang Z, Lisanti MP. Signal transducing molecules and glycosyl-phosphatidylinositol-linded proteins form a caveolin-rich insoluble complex in MDCK cells. J Cell Biol 1993; 122: 789–807
- Minetti G, Low PS. Erythrocyte signal transduction pathways and their possible functions. Curr Opin Hematol 1997; 4: 116–121
- De Flora A, Damonte G, Sdraffa A, Franco L, Benatti U. Heterogeneity of guanine nucleotide binding proteins in human red blood cell membranes. Adv Exp Med Biol 1991; 307: 161–171
- Johnson GJ, Leis LA, Dunlop PC. Specificity of G alpha q and G alpha 11 gene expression in platelets and erythrocytes. Expressions of cellular differentiation and species differences. Biochem J 1996; 318 (Pt 3): 1023–1031
- Premont RT, Buku A, Iyengar R. The G alpha z gene product in human erythrocytes. Identification as a 41-kilodalton protein. J Biol Chem 1989; 264: 14960–14964
- Maurice DH, Waldo GL, Morris AJ, Nicholas RA, Harden TK. Identification of G alpha 11 as the phospholipase C-activating G-protein of turkey erythrocytes. Biochem J 1993; 290 (Pt 3): 765–770
- James SR, Vaziri C, Walker TR, Milligan G, Downes CP. The turkey erythrocyte beta-adrenergic receptor couples to both adenylate cyclase and phospholipase C via distinct G-protein alpha subunits. Biochem J 1994; 304 (Pt 2): 359–364
- Small KM, McGraw DW, Liggett SB. Pharmacology and physiology of human adrenergic receptor polymorphisms. Annu Rev Pharmacol Toxicol 2003; 43: 381–411
- Tuvia S, Moses A, Gulayev N, Levin S, Korenstein R. Beta-adrenergic agonists regulate cell membrane fluctuations of human erythrocytes. J Physiol 1999; 516: 781–792
- Oonishi T, Sakashita K, Uyesaka N. Regulation of red blood cell filterability by Ca2 + influx and cAMP-mediated signaling pathways. Am J Physiol 1997; 273: C1828–1834
- Rasmussen H, Lake W, Allen JE. The effect of catecholamines and prostaglandins upon human and rat erythrocytes. Biochim Biophys Acta 1975; 411: 63–73
- Allen JE, Rasmussen H. Human red blood cells: prostaglandin E2, epinephrine, and isoproterenol alter deformability. Science 1971; 174: 512–514
- Valensi P, Gaudey F, Parries J, Attali JR. Glucagon and noradrenaline reduce erythrocyte deformability. Metabolism 1993; 42: 1169–1172
- Bogin E, Earon Y, Blum M. Effect of parathyroid hormone and uremia on erythrocyte deformability. Clin Chim Acta 1986; 161: 293–299
- Huestis WH, McConnell HM. A functional acetylcholine receptor in the human erythrocyte. Biochem Biophys Res Commun 1974; 57: 726–732
- Hines PC, Zen Q, Burney SN, Shea DA, Ataga KI, Orringer EP, Telen MJ, Parise LV. Novel epinephrine and cAMP- mediated activation of BCAM/Lu-dependent sickle (SS) RBC adhesion. Blood 2003; 101: 3281–3287
- Zennadi R, Hines PC, De Castro LM, Cartron JP, Parise LV, Telen MJ. Epinephrine acts through erythroid signaling pathways to activate sickle cell adhesion to endothelium via LW-alphavbeta3 interactions. Blood 2004; 104: 3774–3781
- Murphy MM, Zayed MA, Evans A, Parker CE, Ataga KI, Telen MJ, Parise LV. Role of Rap1 in promoting sickle red blood cell adhesion to laminin via BCAM/LU. Blood 2005; 105: 3322–3329
- Miceli MC, Moran M, Chung CD, Patel VP, Low T, Zinnanti W. Co-stimulation and counter-stimulation: lipid raft clustering controls TCR signaling and functional outcomes. Semin Immunol 2001; 13: 115–128
- Taner SB, Onfelt B, Pirinen NJ, McCann FE, Magee AI, Davis DM. Control of immune responses by trafficking cell surface proteins, vesicles and lipid rafts to and from the immunological synapse. Traffic 2004; 5: 651–661
- Hiller NL, Akompong T, Morrow JS, Holder AA, Haldar K. Identification of a stomatin orthologue in vacuoles induced in human erythrocytes by malaria parasites, A role for microbial raft proteins in apicomplexan vacuole biogenesis. J Biol Chem 2003; 278: 48413–48421
- Volonte D, Galbiati F, Li S, Nishiyama K, Okamoto T, Lisanti MP. Flotillins/cavatellins are differentially expressed in cells and tissues and form a hetero-oligomeric complex with caveolins in vivo. Characterization and epitope-mapping of a novel flotillin-1 monoclonal antibody probe. J Biol Chem 1999; 274: 12702–12709
- Morrow IC, Parton RG. Flotillins and the PHB domain protein family: rafts, worms and anaesthetics. Traffic 2005; 6: 725–740
- Anderson RG, Jacobson K. A role for lipid shells in targeting proteins to caveolae, rafts, and other lipid domains. Science 2002; 296: 1821–1825
- Tuvia S, Levin S, Bitler A, Korenstein R. Mechanical fluctuations of the membrane-skeleton are dependent on F-actin ATPase in human erythrocytes. J Cell Biol 1998; 141: 1551–1561
- Etienne-Manneville S, Hall A. Rho GTPAses in cell biology. Nature 2002; 240: 629–635
- Vanhauwe JF, Thomas TO, Minshall RD, Tiruppathi C, Li A, Gilchrist A, Yoon EJ, Malik AB, Hamm HE. Thrombin receptors activate G(o) proteins in endothelial cells to regulate intracellular calcium and cell shape changes. J Biol Chem 2002; 277: 34143–34149