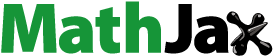
Abstract
The satellite laser geometry calibration method based on terrain matching (terrain matching calibration) has been extensively employed in satellite laser geometry calibration for its simplicity and lack of need for ground probes. In this study, the key factors for the accuracy of the above-mentioned calibration method, i.e. namely the terrain slope and the number of laser points, are examined with the GaoFen-7 (GF-7) satellite as an example. Terrain is classified into six levels in according with the slope classification standards, i.e. Flat (<2°), Micro-slope (2°–5°), Gentle-slope (5°–15°), Moderate-slope (15°–25°), Slope (25°–35°) and Steep-slope (35°–55°). Moreover, a different number of laser points are randomly selected from each the respective terrain slope for calibration. The accuracy of calibration is verified using the true laser pointing obtained based on the ground detector calibration method. As indicated by the experimental results, the terrain matching calibration achieves the optimal experimental conditions when there are over 50 laser points with a terrain slope greater than 15°, or there exist over 20 laser points with a terrain slope greater than 25°. In both cases, the laser pointing accuracy after calibration can exceed 3 arc seconds. This study can provide technical guidance for high-precision terrain matching calibration.
1. Introduction
Satellite laser altimetry, i.e. an active remote sensing technology, refers to an extension of modern radar detection technology from centimetre and millimetre wave detection to optical wave detection. Satellite laser altimetry has high measurement accuracy, temporal, spatial and vertical resolution (Shan and Toth Citation2017). A wide variety of scientific disciplines have been leaping forward with remarkable achievements, which comprise surveying and mapping (Li et al. Citation2022), rivers and lakes research (Guo et al. Citation2021), oceanography investigations (Neumann et al. Citation2022), polar exploration (Zhang et al. Citation2022), as well as planetary exploration (Steinbrugge et al. Citation2022; Xie and Liu Citation2021). However, numerous factors (e.g. the vibration during satellite launch, changes in the space environment after orbiting and vibration during satellite operation) can trigger deviations between the practical pointing parameters of the satellite in orbit and the laboratory design values or previous calibration parameters, such that the accuracy of laser altimetry. Therefore, carrying out geometric calibration of satellite laser is an essential step in obtaining high precision satellite laser altimetry data (Tang and Hu Citation2018).
For laser altimetry satellites, achieving high-frequency on-orbit calibration to eliminate short-term system errors is recognized as a crucial problem that should be addressed. According to whether artificial ground marker is needed, the calibration methods are divided into artificial ground marker calibration method and natural surface calibration method. As depicted in and , artificial ground marker calibration method comprise the Laser Ground Detector (LGD) method (Magruder et al. Citation2001; Xie et al. Citation2018; Tang et al. Citation2021), airborne infrared camera imaging calibration method (Magruder et al. Citation2010), Corner Cube Retroreflector (CCR) calibration method (Magruder et al. Citation2007). The natural surface calibration method includes satellite attitude maneuver calibration method (Luthcke et al. Citation2005; Luthcke et al. Citation2002; Luthcke et al. Citation2000; Rowlands et al. Citation1999), slope terrain calibration method (Liu and Xie Citation2021), as well as terrain matching calibration method (Tang et al. Citation2019). The advantages and disadvantages of various calibration methods are described in detail in and .
Table 1. Artificial ground marker calibration method.
Table 2. Natural Surface calibration method.
Terrain matching calibration method is one of the effective means to achieve high-frequency on-orbit geometry calibration of satellite lasers. Currently, research on the terrain matching calibration method has made certain progress and has been used and validated multiple times on ZiYuan-3 02 (ZY-3 02) satellite, ZY-3 03satellite and GaoFen-7 (GF-7) satellite (Zhang et al. Citation2017). Xie et al. (Citation2018) proposed an on-orbit calibration method based on terrain matching with pyramid-search of spaceborne laser altimeter and Liu et al. (Citation2022) proved that high-precision terrain reference data is conducive to improving terrain matching accuracy through terrain reference data of different precision. Zhao et al. (Citation2022) established a new terrain matching algorithm by matching laser ranging contour with terrain contour. Gao et al. Gao et al. Gao et al. (Citation2023) and other scholars used a random forest model to analyse ICESat-2 satellite data, identifying the factors that influence ICESat-2 data. However, an important problem facing terrain matching calibration method is the selection of the calibration region.
High-precision terrain reference data can improve terrain matching accuracy (Liu et al. Citation2022), but the cost of obtaining high-precision terrain reference data is very expensive, and it is also very difficult to obtain high-precision terrain reference data on a large scale. Therefore, it is necessary to plan the calibration area in advance. The two important factors affecting the selection of calibration area are terrain type and area. Terrain slope is the key factor affecting the terrain types. At the same time, the distance between the laser points of adjacent satellites is relatively fixed, so the required area can be calculated by the number of laser points. Therefore, if the terrain slope range and the number of laser points are determined, the calibration area can be quickly and accurately determined by combining other factors such as the difficulty of obtaining and economic benefits.
The main objectives of this study are to (1) analyse the influence of terrain slope on terrain matching calibration results, (2) analyse the influence of the number of laser points on terrain matching calibration results and (3) find the best terrain slope range and the number of laser points for terrain matching calibration methods. Through statistical analysis of terrain matching results of a large number of GF-7 satellite laser data, the most suitable terrain slope range and the number of laser points are obtained. The results of this study can provide guidance for the selection of terrain-matching calibration area, and promote the laser altimetry satellite to achieve high frequency on-orbit calibration.
The main structure of this study is as follows. The second section introduces the research area, data and methods used, including the satellite laser geometric positioning model, terrain matching model and so on. The third section summarizes and analyses the results of the research. The fourth section is the discussion part, including the preliminary reasoning model of terrain slope and terrain matching accuracy. Section 5 describes the research conclusions and prospects.
2. Materials and methods
2.1. Study area
The experimental area of this study is distributed in Asia and northeast Africa, and it is divided into five regions according to the satellite trajectory and satellite laser data acquisition. As shown in , the selected research area includes a variety of terrain regions, such as the West Siberian Plain, the hills of Kazakhstan, the Mongolian Plateau and the Tibetan Plateau, which meet the selection requirements of large relief terrain and large coverage area. shows the detailed information of each research area.
Table 3. Study area information.
2.2 Data
2.2.1. GF-7 satellite laser data
The GF-7, China’s first civilian submetre stereo mapping satellite, was launched on 3 November 2019. It is equipped with the country’s inaugural laser altimeter system for Earth observation (Xie et al. Citation2023). The GF-7 carries two primary payloads, i.e. two line array stereo mapping cameras and a laser altimeter system. The former presents stereo panchromatic images with a resolution of 0.7 m, while the latter provides high precision ranging data, digital echo waveforms with a 2 GHz sampling rate, as well as laser footprint images with a 3.2 m resolution. The combination of the laser altimeter and the stereo camera is primarily intended for 1:10,000 stereo mapping and large-scale geographic information data updates across China (Xie et al. Citation2020). lists the information regarding the data employed in this study.
Table 4. GF-7 satellite laser data used in this study.
2.2.2. Reference terrain data
The ‘ALOS World 3D-30m’ (AW3D30) refers to a global digital surface model (DSM) dataset released by the Japan Aerospace Exploration Agency (JAXA) in 2016. This dataset originates from the Panchromatic Remote Sensing Instrument for Stereo Mapping (PRISM) carried by the Advanced Land Observing Satellite (ALOS), and it exhibits a horizontal resolution of approximately 30 m (1 arc second) (Takaku et al. Citation2018). With a global elevation accuracy of over 5 m (Takaku et al. Citation2014; Tadono et al. Citation2017), the above-mentioned dataset is a highly reliable resource for researchers and practitioners alike.
The AW3D30 dataset has been widely used in terrain-matching experiments of ZiYuan-3 02 satellite (ZY-3 02), ZiYuan-3 03 satellite (ZY-3 03) and GF-7 satellite. It has achieved good experimental results to meet the required accuracy indicators. At the same time, the DSM dataset as a global coverage can achieve full coverage of the study area. Therefore, the AW3D30 data is an ideal reference terrain dataset for this experiment.
2.2.3. Laser-slope dataset
The slope dataset was obtained by calculating terrain slope data and utilizing the AW3D30 DSM dataset. The slope dataset was then classified according to the slope classification data developed by the Commission on Geomorphic Survey and Cartography of the International Geographical Society. As shown in , this classification classifies slopes into seven categories, i.e. flat (<2°), micro-slope (2°–5°), gentle-slope (5°–15°), moderate-slope (15°–25°), slope (25°–35°), steep-slope (35°–55°) and sharp-slope (>55°). Due to the lack of laser point data in sharp-slope category, this study mainly focuses on the slope in the range of 0°–55°, as there are sufficient laser point data in this range for analysis.
Table 5. Statistical table of laser point quantities under different terrain slopes.
After the slope dataset is generated, the 3D coordinates of the respective laser point are matched in accordance with the laser point data. The satellite laser geometry positioning model is adopted to process the laser point data, which assigns 3D coordinates to each laser point. Using the coordinate position information, the slope dataset is searched to determine the corresponding image element, such that the slope value of the laser point is obtained. In the above-mentioned process, a one-to-one correspondence between the laser point data and its slope value is established. On that basis, the slope-laser dataset is generated, integrating all the data together.
2.3. Methodology
To examine the effect of terrain slope on satellite pointing verification using terrain matching, the following experiments are performed, and the experimental procedure is shown in .
In Step 1, the laser dataset with coordinates is obtained by processing the GF-7 satellite laser dataset using the satellite laser geometric positioning model. Likewise, the terrain slope dataset is obtained by processing the AW3D30 DSM data using the terrain slope calculation method.
In Step 2, the laser point dataset with coordinates and terrain slope dataset are matched and classified using a laser data classification method based on terrain slope. Thus, the slope-laser dataset is generated.
In Step 3, a random sampling method is adopted to extract 10, 20, …, 100 laser points from the slope-laser dataset. The results are grouped based on satellite laser beam type, terrain slope, and laser point number.
In Step 4, calibration experiments are performed for each data group using the terrain matching based satellite laser pointing calibration method. Accordingly, the calibration results for each group of data are obtained.
Lastly, in Step 5, the calibration of each data group is verified using the satellite pointing calibration verification method. This step involves the comparison of the calibration results that are obtained with the real laser pointing obtained based on the LGD calibration results.
2.3.1. Satellite laser geometry positioning model
illustrates the geometric model of the laser altimeter. As depicted in the figure, O-XYZITRF represents the International Terrestrial Reference Frame (ITRF), and O-XYZBODY expresses the coordinate system of the satellite body. L denotes the laser emitter. G denotes the position of the GNSS receiver. represents the vector position of G in the ITRF coordinate system. Ground Point represents the position of the satellite laser point.
expresses the vector position of the Ground Point in the ITRF coordinate system.
represents the spatial offset of the GNSS receiver point in the satellite body coordinate system relative to the satellite centre of mass.
denotes the spatial offset of the laser emission reference point in the coordinate system relative to the satellite centre of mass.
expresses the laser emitted from L to the Ground Point. Based on the geometric model schematic, a satellite laser geometry positioning model can be built (Charlot et al. Citation2020) as follows:
(1)
(1)
where ICRF denotes The International Celestial Reference Frame (Ligas and Banasik Citation2011);
represents the coordinates of the centre of mass of the ground laser spot in the ITRF coordinate system;
expresses the satellite centre of mass coordinate in the ITRF coordinate system;
represents the spatial offset of the GNSS receiver point in the satellite body coordinate system with respect to the satellite centre of mass;
denotes the rotation matrix from the ICRF to the ITRF coordinate system;
is the rotation matrix of the satellite body coordinate system to the ICRF coordinate system;
expresses the spatial offset of the laser emission reference point relative to the satellite center of mass in the satellite body coordinate system.
represents the laser ranging value;
are the vector angle between the laser emission axis and the satellite body coordinate system.
2.3.2. Terrain slope data calculation method
The Earth-Centered Earth-Fixed (ECEF) coordinate system refers to a 3D Cartesian coordinate system with the Earth’s centre serving as its origin. In this system, the X, Y and Z coordinates denote the spatial positions. In this study, terrain slope is calculated by treating the Earth as an ellipsoid and measuring the angle between the terrain surface at the laser point and the surface of the Earth ellipsoid (Blanchard Citation1993; Eberly Citation1999; Yang Citation2009). First, the surface laser point coordinates are converted into X, Y and Z coordinates within the ECEF system for the calculation.
Any terrain surface parallel to the surface of an ellipsoid exhibits a slope of 0. To calculate the slope at the respective location, the least square method (LSM) can be adopted to fit a 3 × 3 neighbourhood plane around each processed pixel, such that a terrain surface of lidar points is formed.
To be specific, the plane is expressed as For the respective image element centre,
denotes the difference between the practical z-value and the fitted z-value. The plane will achieve the best fit when the
minimum is small.
As depicted in , the surface normal and the ellipsoid normal perpendicular to the tangent plane of the ellipsoid surface are calculated at the pixel position after the fitting of the plane. The slope can be determined based on the angle between the ellipsoid normal and the terrain surface normal (. ). The angle presented in
represents the geodesic slope, which is equal to angle
in accordance with the principle of equal geometry.
2.3.3. Terrain matching based satellite laser pointing calibration method
As indicated by the satellite laser geometry positioning model, the satellite will compute varying ground laser points under the constant laser range value and the varying pointing direction. The satellite is subjected to vibrations during launch and operation. This can lead to an erroneous pointing angle of the laser transmitter, compared with the angle measured during launch. Consequently, a spatial disparity arises between the calculated laser point and the practical ground laser point. The pointing angle of the satellite laser transmitter is assumed as and the total number of laser points is assumed as M, Subsequently, the coordinates of all laser points in the pointing direction can be obtained in accordance with the satellite laser geometry positioning model. The set of all laser points is defined as P, and the set of all laser point coordinates is defined as L.
(2)
(2)
(3)
(3)
The function represents the satellite laser geometric positioning model equation.
The value of the DSM at the reference terrain DSM can be obtained as
from the coordinates
in the laser point. Subsequently, the set of all corresponding
ground points is defined G.
(4)
(4)
The sum of the absolute value of the elevation difference between the respective laser point and the corresponding ground point
serves as an indicator to evaluate the pointing
(5)
(5)
denotes the z-value in the 3D coordinate of the
laser point;
represents the z-value of the ground point
corresponding to the
laser point illustrates a schematic representation of the elevation difference calculation between laser points and ground points.
It is assumed that there is a set of N satellites with laser pointing
(6)
(6)
Next, the sum of absolute values of elevation differences can be calculated for the respective point in the set
Subsequently, the sum of absolute values of elevation differences corresponding to the set
can be obtained from the set
(7)
(7)
Afterward, there must exist a set of pointing under which the elevation difference
is the minimum element in the set
such that
is satisfied. Furthermore, the pointing
represents the optimal pointing in the set
of the satellite pointing.
2.3.4. Satellite pointing calibration and verification method
After the completion of calibration, a spatial relationship exists between the calibration pointing and the practical pointing of the satellite (). To facilitate a more intuitive expression, it can be abstracted as , where the laser emitter is taken as the origin of the coordinate system. The vector corresponds to the practical pointing of the satellite, and its corresponding ground point is termed the Real Point. The vector
corresponds to the calibration pointing of the satellite, and its corresponding ground point is termed the Calibration Point. The calibration error
is the spatial angle between the above-mentioned two vectors, which can be expressed as:
(8)
(8)
where
denotes the real pointing laser vector;
represents the calibration pointing laser vector;
expresses the mode lengths of
and
denotes the dot product of the vectors
The value of calibration error
indicates the offset value between calibration pointing and real pointing; the smaller the value of calibration error
the higher the accuracy of the calibration.
3. Results
In this experimental study, the GF-7 satellite laser point data is classified into 120 groups based on two beams, six types of terrain slopes and 10 quantities of satellite laser points. Among the above-mentioned groups, seven groups are deemed invalid due to the insufficient number of satellite laser points, resulting in a total of 113 valid groups. The laser data of each group were calibrated using the following calibration process: Based on EquationEquation (1)(1)
(1) of the satellite laser geometry positioning model, the satellite laser pointing
is represented by
The laboratory calibration pointing
is employed as the initial calibration pointing, and the calibration range is cantered at S= ±1° from this pointing. The calibration step
is determined by the reference terrain DSM grid resolution, resulting in the formation of a pointing set
(9)
(9)
Set for satellite laser pointing is optimized using the terrain matching satellite pointing verification method, such that the optimal pointing for the respective group of verification experiments is determined. For the respective optimal pointing, the calibration error is calculated using the satellite pointing calibration verification method. and list the results of these calculations respectively. ‘Nan’ is used to indicate that the laser point quantity for a specific terrain slope does not conform to the requirement for conducting the corresponding terrain-matching experiment. For instance, in Beam1, there are only 57 Steep-slope laser points. Therefore, terrain-matching experiments requiring 60 or more laser points cannot be conducted, and the value ‘Nan’ is assigned in this case.
Table 6. GF-7 Beam 1 calibration error statistics table.
Table 7. GF-7 Beam 2 calibration error statistics table.
As shown in , RMSE is used as an evaluation index to show the change of calibration errors of Beams 1 and 2 under different conditions. When the number of laser points exceeds 50, the calibration error begins to fluctuate more smoothly. Once the slope reaches or exceeds the Gentle-slope category (i.e. the slope exceeds 15°), the rate of error reduction slows down and tends to stabilize. illustrates the overall variation of the error under a specific condition. The variations of the error under different numbers of laser points and different terrain slope conditions will be discussed in detail later in the text.
Figure 7. Calibration errors for different laser point quantities and calibration errors for different terrain slopes.

3.1. Calibrationing error results of each laser point under different terrain slope conditions
The effect of terrain slope on calibration errors is evaluated under different laser point conditions based on the statistics tables for calibration errors of GF-7 Beam 1 and 2. The terrain slope is categorized into Levels 1–6, representing flat, micro-slope, gentle-slope, moderate-slope, slope, steep-slope and sharp-slope terrain slopes, respectively. As depicted in , Beams 1 and 2 display a similar trend in calibration error transformation with the increase of the laser point number from 10 to 100, suggesting that the calibration error gradually increases with the terrain slope, from flat to very hilly, until it stabilizes. Notably, for micro-slope and gentle slope terrain (slope less than 15°), the calibration error changes dramatically with the increase in terrain slope. However, for moderate-slope and steep-slope (the slope ranging from 15° to 55°), the calibration error remains relatively stable.
3.2. Calibration error results of the respective terrain slope under different conditions of the number of laser points
shows the relationship between the calibration accuracy and the number of laser points under different terrain slopes. As shown in , when the slope is <15°, there is a strong negative correlation between the number of laser points and the calibration error. It can be seen from the figure that the calibration error decreases with the increase of the number of laser points, and the calibration error is in a state of fluctuation after 50 laser points. When the slope is 15°–25°, the Beam 1 calibration error shows a strong correlation with the laser point, and the Beam 2 correlation is not strong. When the slope is >25°, there is a weak relationship between the calibration error and the number of laser points. This shows that when the slope is different, the relationship between the number of laser points and the calibration error is different. When the slope is small, the increase in the number of laser points can reduce the calibration error to a certain extent. However, after the slope >15°, because the calibration error is very small, the number of laser points can reduce the calibration error is very limited. In addition, the slope can be observed in . At <5°, the calibration error is more than 10 s, which is not suitable for terrain-matching experiments. When the slope is 15°–25°, it is necessary to raise the number of laser points more than 50, which can reduce the calibration error to less than 4 s. Beams 1 and 2 have different results at slopes of 25°–35°. The Beam 1 calibration error can be controlled within 3 s after the number of laser points exceeds 50, and the Beam 2 calibration error can be controlled within 3 s after 20 laser points, which may be related to the performance of the two lasers. Therefore, the number of laser points should be increased to 50 or more in this slope range. When the slope is >25°, the calibration error is about 3 s, which can achieve good experimental results. Therefore, 25°–55° is a very ideal slope range.
Figure 9. Influence of the number of laser points on the calibration errors under different terrain slope conditions.

Table 8. Pearson correlation coefficients of Beam1 and Beam2 with the number of laser points under different terrain slopes.
4. Discussion
4.1. The effect of terrain slope on the results of the calibration
As indicated by the calibration results of various laser points under different terrain slope conditions, slope significantly affects the satellite laser pointing calibration based on terrain matching. The calibration results of each laser point quantity suggest that with the increase of the slope, the overall calibration error is decreased. Based on the calibration error results and trends of terrain slope, terrain slope can fall into three categories, i.e. Unsuitable Terrain Slope, Transitional Terrain Slope and Suitable Terrain Slope. The calibration errors of each laser point quantity on flat and micro-slope terrain (slope < 5°) are high, while the accuracy is low. Accordingly, the above-mentioned two types of terrain slope can be referred to as unsuitable terrain matching calibration terrain slope. The calibration error of the gentle-slope and moderate-slope terrain (slope 2°–25°) trends to be decreased, and there are transitional terrain slopes between unsuitable and suitable terrain slopes. The calibration errors of each laser point quantity on slope and steep-slope terrain (slope 25°–55°) are low, while the accuracy is high, and the change is stable, suggesting high stability. Thus, the above-described two types of terrain slope can be termed suitable terrain matching calibration terrain slope.
4.2. Effect of the number of laser points on the results of calibration
The effect of the number of laser points varies with the terrain slope conditions. As revealed by the calibration results, for flat and micro-slope (slope <5°), the calibration error is decreased with the increase of the laser point numbers till reaching a smooth fluctuation. However, the calibration error in the smoothly fluctuating terrain slope area still shows a considerable difference of over 10 s compared with other terrain slope areas. On the other hand, for gentle-slope and moderate-slope (slope 5°–25°), the calibration error is decreased till reaching smooth fluctuation with the increase of the number of laser points. Unlike flat and slightly sloping terrain slopes, the calibration error in this slope area can still fall into 5 s when fluctuating smoothly. Moreover, the calibration error is even lower, falling into 3 s under the sufficient laser points. However, under the low number of laser, the calibration error in this area tends to be increased. For hilly and very hilly (slope >25°), the calibration error is generally lower in this region, and the number of laser points is less affected. The maximum value of calibration error for slopes and steep slopes is less than 3.5 s, and it can go down to less than 3 s under the sufficient laser points. Consequently, the number of laser points significantly influences terrain slopes with a slope <25°, and the overall trend indicates that the calibration error decreases and accuracy increases with an increase in the number of laser points.
4.3. Comprehensive analysis of terrain matching calibration results by terrain slope and number of laser points
The effects of terrain slope on calibration results and the effects of the number of laser points on calibration results are analysed. As indicated by the result of the above analysis, the overall calibration results of Beams 1 and 2 are similar and can be discussed together. In accordance with the calibration results of terrain slopes, the terrain slopes can be categorized into three main classes, i.e. unsuitable terrain slopes, transitional terrain slopes, and suitable terrain slopes ().
Table 9. Accuracy table for different terrain slope types.
The calibration error can be reduced by increasing the slope. However, as indicated by the statistical table for laser points at different terrain slopes, only 9.31% of the laser points apply to terrain matching calibration. Even when the moderate-slope terrain slope is included, the number of laser points only accounts for 18.7%. Besides, when considerable laser points can be obtained for global or large regional terrain matching calibrations, high-precision short-period satellite laser on-orbit geometry calibrations require high-precision DSM reference terrain data, which are difficult to obtain for such large scales.
To address the above-mentioned challenge, an asymptotic strategy is proposed in this study based on the analysis and discussion of terrain slope and laser points. The laser points suitable for terrain matching calibration should be selected as much as possible under the target number of laser points of ≥50. Laser points from Moderate-slope terrain can be added under the insufficient number. If the number remains insufficient, some laser points with a higher slope in gentle slopes can be introduced to create a laser point dataset for terrain matching.
4.4. The relationship between terrain slope and calibration error
As shown in , the geometric relationship between the laser altimeter satellite and the terrain slope is established. represents the real pointing laser,
represents the calibration pointing laser,
represents the inclination angle of the laser emitter,
represents the angle between the calibration pointing and the real pointing and
represents the terrain slope. Real Point indicates the laser Point after the Calibration; Calibration Point indicates the laser point after the calibration; Ground Point indicates the ground point corresponding to the calibration laser point. The horizontal plane at the 'Real Point’ is considered as the base plane. The height from the ‘Ground Point’ to the base plane is denoted by
the height from the 'Calibration Point’ to the base plane is denoted by
and the height difference between the two is represented by
In the terrain matching calibration process, the laser ranging value is constant, so
is equal to
in terms of the ranging value
The following formula can be obtained according to the terrain matching model diagram
():
(10)
(10)
The value in the formula is closely related to the installation of the laser, and the satellite generally does not change greatly after orbit operation. In addition, based on the elevation accuracy
of the reference terrain data,
can not accurately describe the position relationship between the laser point and the real terrain when
theoretically. Therefore, the angle between the calibration direction and the real direction when
is selected as the theoretical calibration accuracy.
As shown in , the trend of the actual calibration results is completely consistent with the theoretical results. However, because the theoretical accuracy model is only a preliminary reasoning model, it does not consider many factors, such as laser ranging error, terrain roughness and so on. Therefore, the theoretical model results should be better than the actual results. However, in gentle-slope, moderate-slope and slope terrain categories, the actual accuracy surpasses the theoretical accuracy. This discrepancy indicates that the model is not perfect and requires further refinement. Unfortunately, although the calibration error and the number of laser points have been shown to be correlated experimentally, we have not derived a functional relationship between them. The theoretical accuracy model of terrain matching and the relationship between the number of laser points and the calibration error are the directions we strive to study.
5. Conclusions
In this study, we analyse the effects of terrain slope and number of laser points on satellite laser pointing calibration based on terrain matching. The results show that the slope has a great influence on the geometric calibration of the satellite laser based on terrain matching, and the number of laser points affects the calibration results to some extent. The terrain slope suitable for terrain matching ranges from 25° to 55°, in which only 20 laser points are needed to obtain good calibration results. When the slope ranges from 15° to 25°, at least 50 laser points are needed to obtain better calibration results. Therefore, the recommended calibration area is where the slope is greater than 25°, ensuring that each beam has at least 20 data points. However, if this condition is difficult to achieve, the slope condition can be relaxed to greater than 15°, but it is necessary to ensure that at least 50 laser points are available to achieve more ideal calibration results. At the same time, the theoretical accuracy model of terrain matching is discussed. It can be seen from our model that slope and reference terrain accuracy are very important factors affecting terrain matching results, whereas other factors such as the number of laser points are not included in the model. The further improvement and optimization of the model should be the direction of our further research.
Disclosure statement
All authors declare that they have no known competing financial interests or personal relationships in this paper.
Data availability statement
The AW3D30 DSM data are available via the JAXA Earth Observation Research Center website (https://www.eorc.jaxa.jp/ALOS/en/aw3d30/data/index.htm, accessed on 10 March 2023); the GF-7 satellite laser data are available on the Natural Resources Satellite Remote Sensing Cloud Service Platform (http://sasclouds.com/chinese/normal/, accessed on 15 October 2022).
Additional information
Funding
References
- Blanchard W. 1993. GPS–theory and practice. B. Hofmann-Wellenhof H. Lichtenegger and J. Collins. Springer-Verlag, Vienna. 326 pp. DM 79. J Navigation. 46(3):457–457. doi: 10.1017/S0373463300011966.
- Charlot P, Jacobs CS, Gordon D, Lambert S, de Witt A, Böhm J, Fey AL, Heinkelmann R, Skurikhina E, Titov O, et al. 2020. The third realization of the international celestial reference frame by very long baseline interferometry. A&A. 644: a 159. doi: 10.1051/0004-6361/202038368.
- Eberly DH. 1999. Least squares fitting of data by linear or quadratic structures. Geometric Tools. 55.
- Gao M, Xing S, Zhang GP, Zhang XL, Li PC. 2023. Assessment of ICESat-2’s horizontal accuracy using an iterative matching method based on high-accuracy terrains. Remote Sens. 15(9):2236. doi: 10.3390/rs15092236.
- Guo XZ, Jin SG, Zhang ZJ. 2021. Evaluation of water level estimation in the upper Yangtze River from ICESat-2 data. Paper presented at 2021 Photonics & Electromagnetics Research Symposium (PIERS); Hangzhou, China. IEEE. p. 2260–2264. doi: 10.1109/PIERS53385.2021.9695146.
- Li BB, Xie H, Tong XH, Tang H, Liu SJ, Jin YM, Wang C, Ye Z. 2022. High-accuracy laser altimetry global elevation control point dataset for satellite topographic mapping. IEEE Trans Geosci Remote Sens. 60:1–16. doi: 10.1109/TGRS.2022.3177026.
- Ligas M, Banasik P. 2011. Conversion between Cartesian and geodetic coordinates on a rotational ellipsoid by solving a system of nonlinear equations. Geodesy Cartograph. 60(2):145–159. doi: 10.2478/v10277-012-0013-x.
- Liu R, Xie JF, Xu CP, Zeng JZ, Mo F, Yang XM. 2022. A separate calibration method of laser pointing and ranging for the GF-7 satellite laser that does not require field detectors. Remote Sens. 14(23):5935. doi: 10.3390/rs14235935.
- Liu R, Xie JF. 2021. Calibration of the laser pointing bias of the GaoFen-7 satellite based on simulation waveform matching. Opt Express. 29(14):21844–21858. doi: 10.1364/OE.423679.
- Luthcke SB, Carabajal CC, Rowlands DD. 2002. Enhanced geolocation of spaceborne laser altimeter surface returns: parameter calibration from the simultaneous reduction of altimeter range and navigation tracking data. J Geodyn. 34(3–4):447–475. doi: 10.1016/S0264-3707(02)00047-9.
- Luthcke SB, Rowlands DD, Mccarthy JJ, Pavlis DE, Stoneking E. 2000. Spaceborne laser-altimeter-pointing bias calibration from range residual analysis. J Spacecraft Rock. 37(3):374–384. doi: 10.2514/2.3571.
- Luthcke SB, Rowlands DD, Williams TA, Sirota M. 2005. Reduction of ICESat systematic geolocation errors and the impact on ice sheet elevation change detection. Geophys Res Lett. 32(21):312–321. doi: 10.1029/2005GL023689.
- Magruder LA, Schutz BE, Silverberg EC. 2001. Pointing angle and timing calibration/validation of the Geoscience Laser Altimeter with a ground-based detection system. Paper presented at IGARSS 2001. Scanning the Present and Resolving the Future. Proceedings. IEEE 2001 International Geoscience and Remote Sensing Symposium (Cat. No.01CH37217); Vol. 4. Sydney, NSW, Australia. p. 1584–1587. doi: 10.1109/IGARSS.2001.977001.
- Magruder LA, Webb CE, Urban TJ, Silverberg EC, Schutz BE. 2007. ICESat altimetry data product verification at white sands space harbor. IEEE Trans Geosci Remote Sens. 45(1):147–155. doi: 10.1109/TGRS.2006.885070.
- Magruder LA, Ricklefs RL, Silverberg EC, Horstman MF, Suleman MA, Schutz BE. 2010. ICESat geolocation validation using airborne photography. IEEE Trans Geosci Remote Sens. 48(6):2758–2766. doi: 10.1109/TGRS.2010.2040831.
- Neumann T, Magruder L, Kurtz N. 2022. ICESat-2 mission: contributions of a spaceborne lidar to ocean science. Paper presented at OCEANS 2022; Hampton Roads, VA. p. 1–5. doi: 10.1109/OCEANS47191.2022.9977260.
- Rowlands DD, Pavlis DE, Lemoine FG, Neumann GA, Luthcke SB. 1999. The use of laser altimetry in the orbit and attitude determination of Mars Global Surveyor. Geophys Res Lett. 26(9):1191–1194. doi: 10.1029/1999GL900223.
- Shan J, Toth CK. 2017. Topographic laser ranging and scanning: principles and processing. 2nd ed. Boca Raton (FL): CRC Press. doi: 10.1201/9781315154381.
- Steinbrugge G, Haynes MS, Schroeder DM, Scanlan KM, Stark A, Young DA, Grima C, Kempf S, Ng G, Buhl D, et al. 2022. Altimetry measurements from planetary radar sounders and application to SHARAD on mars. IEEE Trans Geosci Remote Sens. 60:1–14. doi: 10.1109/TGRS.2021.3134638.
- Tadono T, Takaku J, Ohgushi F, Doutsu M, Kobayashi KI. 2017. Updates of ‘AW3D30’ 30 M-MESH global digital surface model dataset. Paper presented at 2017 IEEE International Geoscience and Remote Sensing Symposium (IGARSS); Fort Worth (TX). p. 5656–5657. doi: 10.1109/IGARSS.2017.8128290.
- Takaku J, Tadono T, Tsutsui K. 2014. Generation of high resolution global DSM from ALOS PRISM. Int Arch Photogramm Remote Sens Spatial Inf Sci. XL-4:243–248. doi: 10.5194/isprsarchives-XL-4-243-2014.
- Takaku J, Tadono T, Tsutsui K, Ichikawa M. 2018. Quality improvements of ‘AW3D’ global DSM derived from Alos Prism. Paper presented at IGARSS 2018–2018 IEEE International Geoscience and Remote Sensing Symposium; Valencia, Spain. p. 1612–1615. doi: 10.1109/IGARSS.2018.8518360.
- Tang XM, Hu F. 2018. Development status and trend of satellite mapping. Spacecraft Recover Remote Sens. 39:26–35. doi: 10.3969/j.issn.1009-8518.2018.04.004.
- Tang XM, Xie JF, Mo F, Dou XH, Li X, Li SN, Li S, et al. 2021. GF-7 dual-beam laser altimeter on-orbit geometric calibration and test verification. Acta Geodaetic Cartograph Sin. 50(3):384–395. doi: 10.11947/j.AGCS.2021.20200397.
- Tang XM, Xie JF, Gao XM, Mo F, Feng WW, Liu R. 2019. The in-orbit calibration method based on terrain matching with pyramid-search for the spaceborne laser altimeter. IEEE J Sel Top Appl Earth Observ Remote Sens. 12(3):1053–1062. doi: 10.1109/JSTARS.2018.2890552.
- Xie JF, Liu R. 2021. Sliding window Gaussian fitting algorithm for ranging error suppression of full-waveform spaceborne laser. Acta Geodaetic Cartograph Sin. 50(9):1240–1250. doi: 10.11947/j.AGCS.2021.20200466.
- Xie JF, Mo F, Feng WW, Liu R. 2018. The on-orbit calibration method based on terrain matching with pyramid-search for the spaceborne laser altimeter. Paper presented at IGARSS 2018–2018 IEEE International Geoscience and Remote Sensing Symposium; Valencia, Spain. p. 1978–1981. doi: 10.1109/IGARSS.2018.8518718.
- Xie J, Huang G, Liu R, Zhao C, Dai J, Jin T, Mo F, Zhen Y, Xi S, Tang H, et al. 2020. Design and data processing of China’s first spaceborne laser altimeter system for earth observation: gaoFen-7. IEEE J Sel Top Appl Earth Observ Remote Sens. 13:1034–1044. doi: 10.1109/JSTARS.2020.2977935.
- Xie JF, Liu R, Tang XM, Yang XM, Zeng JZ, Mo F, Mei YK. 2023. A geometric calibration method without a field site of the GF-7 satellite laser relying on a surface mathematical model. IEEE Trans Geosci Remote Sens. 61:1–14. doi: 10.1109/TGRS.2022.3231902.
- Xie J, Tang X, Mo F, Tang H, Wang Z, Wang X, Liu Y, Tian S, Liu R, Xia X, et al. 2018. In-orbit geometric calibration and experimental verification of the ZY3-02 laser altimeter. Photogram Rec. 33(163):341–362. doi: 10.1111/phor.12249.
- Yang B. 2009. The research of mountains ontology and digital classification in the framework of ‘digital mountains’—A case study in Sichuan Province [Ph.D thesis]. Sichuan: Chengdu University of Technology.
- Zhang G, Li SN, Huang WC, Li DR. 2017. Geometric calibration and validation of ZY3-02 satellite laser altimeter system. Geomat Inform Sci Wuhan Univ. 42(11):1589–1596. doi: 10.13203/j.whugis20160514.
- Zhang SK, Tong G, Zhu CH, Li JX, Li X, Zhu BX, Liu LX, Feng X. 2022. Arctic sea ICE freeboard estimation and variations from operation Icebridge. IEEE Trans Geosci Remote Sens. 60:1–10. doi: 10.1109/TGRS.2022.3185230.
- Zhao PF, Li S, Ma Y, Liu XY, Yang J, Yu D. 2022. A new terrain matching method for estimating laser pointing and ranging systematic biases for spaceborne photon-counting laser altimeters. ISPRS J Photogramm Remote Sens. 188:220–236. doi: 10.1016/j.isprsjprs.2022.04.015.