Abstract
In the laboratory setting, typical endocrine and targeted behavioral tests are limited in their ability to provide a direct assessment of stress in animals housed in undisturbed conditions. We hypothesized that an automated phenotyping system would allow the detection of subtle stress-related behavioral changes well beyond the time-frames examined using conventional methods. In this study, we have utilized the TSE PhenoMaster system to continuously record basal behaviors and physiological parameters including activity, body weight, food intake and oxygen consumption in undisturbed and stressed C57Bl/6J male mice (n = 12/group), with a pharmacological intervention using the conventional anxiolytic, diazepam (5 mg kg−1 i.p.; n = 8/group). We observed significant 20–30% reductions in locomotor activity in the dark phase, with subtle reductions in light phase activity for up to 96 h following a single 2 h episode of restraint stress. A single administration of diazepam reduced plasma corticosterone concentrations by 30–35% during stress exposure when compared to mice treated with vehicle. This treatment did not result in significantly different locomotor activity compared to vehicle within the first 48 h following restraint stress. However, diazepam treatment facilitated restoration of locomotor activity at 72 and 96 h after restraint stress exposure in comparison to vehicle-treated mice. Hence, the use of an automated phenotyping system allows a real time assessment of basal behaviors and empirical metabolism following exposure to restraint stress and demonstrates major and subtle changes in activity persist for several days after stress exposure.
Introduction
Induction of psychological stress in the laboratory setting is a useful and highly studied technique known to cause a plethora of quantifiable changes from the behavioral to molecular level. It has been well documented that stress and the consequent responses are associated with many multifactorial diseases including psychiatric disorders, various inflammatory-related and neurodegenerative diseases (Glaser & Kiecolt-Glaser, Citation2005; Machado et al., Citation2014; Wingenfeld & Wolf, Citation2011). To examine the effect of stress and its involvement in different disorders, researchers have employed multiple types of stressors including restraint/immobilization (Koo et al., Citation2010), cold/heat (Djordjevic et al., Citation2003) and lipopolysaccharide challenge (Perez-Nievas et al., Citation2010). Typically, assessment of stress utilizes both physiological and behavioral indicators, with one of the most commonly measured physiological responses being activation of the hypothalamic-pituitary-adrenal (HPA) axis, which ultimately triggers the release of glucocorticoids from the adrenal gland (Engelmann et al., Citation2004). Furthermore, multiple behavioral tests including forced swim, open field, elevated plus maze, sucrose preference and step down avoidance are also used to assess the impact of stress in animals (Beery & Kaufer, Citation2015; Haller & Alicki, Citation2012; Nishimura et al., Citation1989).
Although activation of the HPA axis can be monitored in blood through repeated sampling via catheters and dialysis, these methods are invasive and require surgical intervention. This limits their ability to provide a direct assessment of stress in live animals housed in undisturbed conditions. Several studies have attempted to assess the validity of noninvasive behavioral tests impacted by stress exposure by measuring parameters, such as ambulation, rearing and grooming activity (Brodkin et al., Citation2014; Zimprich et al., Citation2014). In addition to these tests, high-throughput automated systems, such as the TSE PhenoMaster have been developed to examine continuous undisturbed home-cage activity of rodents including locomotion and calorimetry (Clemmensen et al., Citation2015; Greenman et al., Citation2013). Given the wealth of knowledge on the effects of diazepam and stress on rodent behaviors (Belzung, Citation2001; Zhao et al., Citation2012), this study aims to test the sensitivity of the automated TSE PhenoMaster system and identify the time course of behavioral changes occurring after a single episode of restraint stress with or without a pharmacological intervention using diazepam. This may provide a platform to test stress-related compounds and their effects using continuously monitored behaviors in undisturbed conditions beyond time-domains that are practically achievable using typical experimental procedures.
Methods
Animals
Wild-type C57Bl/6J male mice aged 10–11 weeks were obtained from the Animal Resources Center in Canning Vale, Australia and group housed 4 per cage on arrival in the University of Queensland animal facility. Mice were housed in a 12-h light: dark phase (on at 06:00 h and off at 18:00 h) with room temperature maintained at 24 ± 2 °C. One week before experimental manipulation, mice were relocated to the housing cages within the TSE PhenoMaster system, where mice were housed individually with free access to food and water with cage temperature tightly regulated at 24.5 ± 0.1 °C. Mice were allowed 7 d to acclimate to the new housing conditions before the commencement of all experiments. All experimental procedures were approved by the University of Queensland Animal Ethics Committee and complied with the policies and regulations regarding animal experimentation. They were conducted in accordance with the Queensland Government Animal Research Act 2001, associated Animal Care and Protection Regulations (2002 and 2008) and the Australian Code of Practice for the Care and Use of Animals for Scientific Purposes, 8th Edition (National Health and Medical Research Council, 2013).
Experimental protocol
Part A
For the first part of the experiment, body weight, food intake, oxygen consumption and activity were examined by monitoring baseline levels of these parameters for 24 h in mice (n = 12) housed within an automated TSE PhenoMaster system prior to the application of restraint stress (termed “Baseline”). All mice were subsequently subjected to a single 2-h restraint stress from 10:00 to 12:00 h using cylindrical metal restrainers (3 × 7 cm) equipped with nasal ventilation holes and a rear aperture clip. Immediately following stress cessation, mice were monitored for 96 h within the automated TSE PhenoMaster system (termed “Post-Stress”; ).
Figure 1. Schematic timeline of experimental procedures. (A) In the first part of the experiment, naïve mice were habituated for a period of 7 d (green) to the home cages of the TSE PhenoMaster system after which 24 h (12 h light and 12 h dark; 10:00–10:00 h) of behavioral recording was collected (Baseline) prior to application of a 2 h restraint stress (10:00–12:00 h; red). Following stress application, mice were returned to the home cages and behavioral recording was continuously collected for 96 h (12:00–12:00 h; blue). (B) In the second part of the experiment, naïve mice were habituated for a period of 7 d (green) followed by a single intraperitoneal injection of diazepam (DZP; 5 mg kg−1) or vehicle (VEH) at 09:30 h. After 30 min, recordings were collected for 2 h for initial response to injection (10:00–12:00 h) and a further 48 consecutive hours (12:00–12:00 h; blue). (C) The third experiment involved a similar experimental procedure to Part A with the inclusion of the single intraperitoneal injection of DZP or VEH 30 min prior (09:30 h; black) to application of a 2 h restraint stress (10:00–12:00 h; red). (D) In the last experiment, naïve mice were habituated for a period of 7 d (green). The baseline blood samples were collected from the tail, followed by a single intraperitoneal injection of DZP or VEH 30 min prior (09:30 h; black) to application of a 2 h restraint stress (10:00–12:00 h; red). Subsequent blood samples were collected at 1 h (11:00 h) and 2 h (12:00 h) time points following restraint stress application.
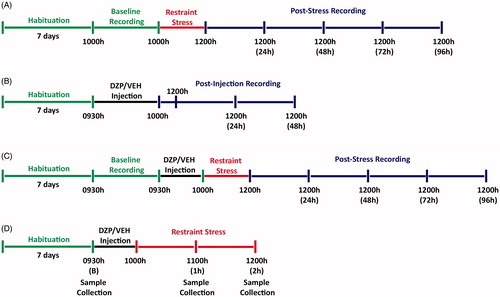
Part B
For the second part of the experiment, the effects of diazepam on body weight, food intake, oxygen consumption and activity were examined over time by monitoring a new cohort of naïve mice in TSE PhenoMaster system for 50 consecutive hours (beginning at 10:00 h) 30 min after mice (n = 8/group) were injected intraperitoneally with diazepam (5 mg kg−1; Hospira Pty. Ltd., Melbourne, Australia) or vehicle (53% propylene glycol, 31% ethanol and 16% water, pH =7.0) at a final volume of 1 mL kg−1 ().
Part C
For the third part of the experiment, the effects of diazepam on restraint stress-induced changes in body weight, food intake and activity were examined over time by monitoring another cohort of naïve mice in the TSE PhenoMaster system for 96 consecutive hours after mice (n = 8/group) were injected intraperitoneally with diazepam (5 mg kg−1) or vehicle 30 min prior to the onset of the 2-h restraint stress at 10:00–12:00 h ().
Part D
In a separate experiment, the effects of diazepam on the restraint stress-induced responses in corticosterone and blood glucose concentrations were examined. Blood from diazepam- and vehicle-treated mice was collected prior to and during restraint stress exposure (). Specifically, the distal 1 mm at the very tip of the tail was cut once only and blood samples were collected into chilled heparinized glass hematocrit tubes (HEMATO-CLAD® Mylar®, Drummond Scientific Company, Broomall, PA) and sealed with hematocrit clay (BD Seal-Ease™). Mice were then intraperitoneally injected with diazepam and vehicle 30 min prior to application of a 2-h restraint stress. Subsequent blood samples were collected at 1 and 2 h, while mice were in the restrainers, by gently removing clotted blood from the tail. Each blood sample was approximately 25–30 μL, with the total volume collected much less than 10% of the calculated total blood volume. A 2.5 μL aliquot of whole blood was also used to measure blood glucose using a standard glucometer (Medisense® Optium glucometer, Abbot Laboratories, Waltham, MA) with an assay range of 1.1–27.8 mmol L−1 (20–500 mg dL−1). Whole blood samples were centrifuged at 1250 × g for 15 min and supernatant plasma was collected and stored at −80 °C for later analysis of corticosterone concentrations.
Assessment of body weight, food intake, activity and metabolism
The TSE PhenoMaster system comprises two environmental chambers each housing up to eight individual cages, allowing the monitoring of 16 mice in parallel with high resolution for activity, calorimetry, body weight and food consumption. Oxygen consumption and carbon dioxide production in cages were measured through an open-circuit indirect calorimetry system and gas-sensing units where oxygen and carbon dioxide sensors were calibrated with calibration gas mixtures (CO2: 0.05 ± 0.0005%, O2: 20.8 ± 0.1% in N2; CO2: 0.95 ± 0.009%, O2: 20 ± 0.1% in N2) and the sample airflow adjusted to 0.25 L min−1. The cages also contain high precision sensor-associated weight and feeding baskets to accurately measure body weight and food intake (grams), with the feeders filled with standard chow and water bottles filled with distilled water. The apparatus is also equipped with a multi-dimensional infrared beam system for real time measurement of locomotor activity, defined as the total number of infrared beam breaks in the X and Y-axis (counts), where the beam break counts are subdivided into: (1) fine movement or grooming resulting in the repeated break of the same light beam and (2) ambulatory movement which results in breaks of consecutive light beams. All measurements were taken hourly for the duration of assessment.
Corticosterone assay
Plasma corticosterone concentrations were determined as described previously (Chen et al., Citation2014). Briefly, a 5 μL aliquot of plasma was extracted in 2 mL of glass-distilled dichloromethane with a mean extraction recovery of 85.2% and assay sensitivity of 10 pg mL−1. Extracts were dried under nitrogen gas and reconstituted in phosphate buffered saline containing 0.1% porcine gelatin (w/v). Unlabeled corticosterone standards and samples were incubated with anti-corticosterone antibody (Sapphire Bioscience Pty Ltd., Redfern, Australia) and [1, 2, 6, 7-3H]-corticosterone tracer. Unbound steroids were removed by addition of a dextran charcoal suspension followed by centrifugation at 1000 × g for 10 min at 4 °C. Radioactivity was quantified in a 100-μL aliquot of the resulting supernatant using a Liquid Scintillation Spectrometer (Tri-Carb 3100TR, Perkin Elmer, Waltham, MA) and samples were determined from the standard curve. Intra-assay and inter-assay coefficients of variation were 4.33 and 3.83%, respectively.
Statistical analysis
All measures were performed using GraphPad Prism version 6.0 (GraphPad Software, Inc., San Diego, CA). For Part A, phenotype measures recorded for 24 h prior to restraint stress were used for baseline data termed “Baseline”, and compared with “Post-Stress” recordings analyzed at 24-h intervals for 96 h following the release of mice from restraints. Data for “Baseline” and “Post-Stress” at 24, 48, 72 and 96 h for measures of body weight, food intake, oxygen consumption, locomotor and fine activity were analyzed using repeated measures one-way ANOVA with Greenhouse-Geisser correction for sphericity and a post-hoc analysis using Dunnett’s test. For Part A and B, fine and locomotor activity were analyzed hourly for 4 h after restraint stress and injection to determine the initial response to either restraint stress or the injection procedure. All 24-h cumulative counts for each measurement throughout the experiment, including Part B, were taken from 2 h after the onset of treatment (i.e. at 12:00 h) to account for the time animals were held in restraint. For Parts B, C and D, the statistical differences between vehicle and diazepam treatment for body weight, food intake, oxygen consumption, locomotor activity, fine activity, corticosterone and blood glucose concentrations were analyzed using repeated measures two-way ANOVA comparing the main effects of drug treatment and time following restraint stress. Where significant main effects were observed in the repeated measures two-way ANOVA, a post-hoc analysis was performed using a Fisher’s least significant difference test for each time point. All data are presented as mean ± SEM and differences were considered significant when p ≤ .05.
Results
Physical restraint stress resulted in a sustained reduction in diurnal activity
Using the automated TSE PhenoMaster system, we monitored circadian locomotor and fine activity continuously for 96 consecutive hours following a single 2-h episode of restraint stress (). Repeated measures one-way ANOVA showed that locomotor activity was significantly reduced [F (2.31, 25.45) = 10.89, p = .0002]. Compared to baseline activity scores, there was an approximate 30% (p < .01) and 20% (p < .05) reduction in locomotor activity during the 24 and 48 h periods immediately following restraint stress exposure, respectively (). Repeated measures one-way ANOVA showed that locomotor activity during the light and dark phase was significantly reduced [F (3.35, 36.79) = 5.94, p = .001; F (2.42, 26.66) = 9.15, p = .0005, respectively]. Interestingly, the post-hoc analysis revealed a decrease in locomotor activity in the light phase at 24 h (p < .01), 48 h (p < .05), 72 h (p < .05) and 96 h (p < .05) after restraint stress exposure, whereas locomotor activity was decreased at 24 h (p < .01) after restraint stress exposure in the dark phase (). A change in total locomotor activity was also reflected by a significant decrease in fine activity [F (2.16, 23.71) = 6.03, p = .007]. Post-hoc analysis showed an approximate 20% (p < .01) and 15% (p < .01) decrease in fine activity at 24 and 48 h following restraint, respectively (). As with locomotor activity, fine activity during the light and dark phases was significantly decreased [F (2.88, 31.64) = 2.98, p = .048; F (2.55, 28.1) = 6.26, p = .003, respectively]. Post-hoc analysis showed a reduction in fine activity at 48 h (p < .05) after restraint stress during light phase and decreases in fine activity during the dark phase were evident at 24 h (p < .05) following restraint (). Interestingly, repeated measures one-way ANOVA showed that 1 h following restraint stress fine activity significantly increased by approximately 280% [F (2.51, 27.64) = 34.33, p < .0001], while no other significant changes were observed at 2, 3 and 4 h after restraint ().
Figure 2. Single episode of two-hour restraint stress induces a prolonged reduction in locomotor and fine activity but no change in metabolic rate. Line graphs of continuous recording of (A) total locomotor activity, (D) fine activity, and (H) oxygen consumption during the dark (grey) and light (white) phase prior to and after acute restraint stress exposure (n = 12). The standard errors of mean (SEM) are indicated by dashed lines. The red vertical line depicts time of restraint. Mean ± SEM cumulative counts per 24 h in (B) total locomotor activity and (F) fine activity were recorded for 96 h following acute restraint stress (blue) when compared to the baseline recording (“B”; green). These were separated into light and dark phase components for up to 96 h following restraint stress (C & G). (E) Fine activity for 4 h following acute restraint stress (blue) was compared to the fine activity prior to restraint stress application (green). (I) Mean ± SEM cumulative 24 h measurements of oxygen consumption prior to and after acute restraint stress exposure were monitored for up to 96 h and (J) shows linear regression of metabolic rates (energy expenditure relative to body weight) at baseline (green) and 24 h (blue) after restraint stress. *p < .05, **p < .01, ***p < .001 when compared to baseline recording; repeated measures one-way ANOVA with Dunnett’s test.
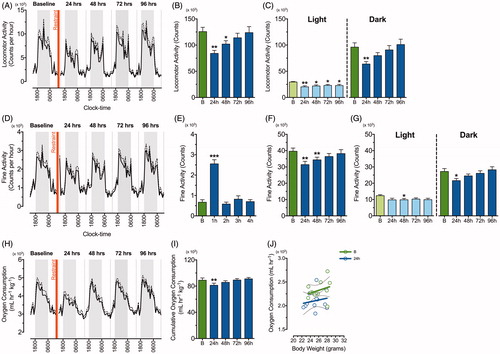
Oxygen consumption was also monitored (), with a repeated measures one-way ANOVA showing that there was a significant reduction over the time-course of the experiment [F (2.21, 24.26) = 5.81, p = .007]. Post-hoc analysis showed that cumulative rate of oxygen consumption was decreased in these mice at 24 h following restraint stress likely reflecting the reduction in locomotor activity (p < .01; ). While mice consumed less oxygen during this time, energy expenditure relative to body weight did not change between baseline and 24 h post-stress measurements, indicating there was no measureable change in metabolic rate due to acute restraint stress (). We also observed a transiently decreased body weight and food intake in mice during the 24-h period following restraint (data not shown).
Diazepam facilitated recovery of restraint-induced reduction in activity and reduced corticosterone output
Initially, the effect of diazepam alone on locomotor and fine activity was examined for 50 consecutive hours following injection (). The repeated measures two-way ANOVA demonstrated reduction in locomotor and fine activity following diazepam treatment [F (1, 14) = 18.28, p = .0008; F (1, 14) = 17.34, p = .001] in the period immediately following injection [F (3, 42) = 28.83, p < .0001; F (3, 42) = 27.81, p < .0001]. There was also a significant interaction between diazepam treatment over time on locomotor and fine activity [F (3, 42) = 26.96, p < .0001 and F (3, 42) = 17.74, p < .0001, respectively]. Specifically, post-hoc analysis showed that locomotor and fine activity significantly differed between treatment groups at 1 h (p < .001) and 2 h (p < .001) post injection (). However, there were no significant differences in cumulative locomotor activity (), cumulative fine activity () and oxygen consumption () for the 24- and 48-h periods following vehicle or diazepam injection. The effects of diazepam on body weight and food intake were also examined post injection. While there was no difference in body weight for diazepam- and vehicle-treated mice, a transient increase in food intake after diazepam was observed 24 h post-injection when compared to the vehicle group (data not shown).
Figure 3. Diazepam alone does not affect oxygen consumption, locomotor and fine activity. A line graph demonstrating continuous recording of (A) total locomotor activity and (D) fine activity during the dark (grey) and light (white) phase after vehicle (green line) and diazepam (blue line) injection (n = 8 per group). The standard errors of mean (SEM) are illustrated as dashed green and blue lines. (B & E) Locomoter and fine activity for 4 h following acute restraint stress was compared between vehicle (green bar) and diazepam (blue bar). Mean ± SEM cumulative 24 h measurements in (C) locomotor activity, (F) fine activity and (G) oxygen consumption were compared in mice treated with vehicle (green bar) and diazepam (blue bar) at 24 and 48 h post injection (n = 8 per group). ***p < .001 when comparing between vehicle and diazepam at each time point; repeated measures two-way ANOVA with Fisher’s LSD test.
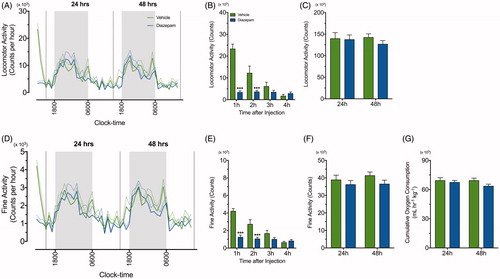
The effect of diazepam on locomotor and fine activity was also monitored for the 96 h post-restraint stress period (). Repeated measures two-way ANOVA showed main effects of diazepam treatment [F (1, 14) =4.43, p = .05] and time [F (4, 56) = 29.83, p < .0001] on locomotor activity following restraint without significant interaction between treatment and time. Mice treated with diazepam showed increased locomotor activity at 72 h (p < .05) and 96 h (p < .05) post-stress when compared to the vehicle group (). The repeated measures two-way ANOVA on locomotor activity data during the light and dark phase after restraint stress exposure revealed significant main effects of time [F (4, 56) = 5.86, p = .0005; F (4, 56) = 37.84, p < .0001] and diazepam treatment [F (1, 14) = 4.42, p = .05; F (1, 14) = 3.86, p = .05] without any significant interaction between the two effects. There was a transient increase in locomotor activity at 72 h (p < .01) post-stress during the light phase with a more prominent increase at 72 h (p < .05) and 96 h (p < .05) hours post-stress during the dark phase for diazepam-treated mice (). Conversely, although fine activity was significantly reduced in the time following restraint [F (4, 56) = 11.99, p < .0001], diazepam treatment did not effectively attenuate these stress-induced reductions [F (1, 14) = 4.36, p = .056] at 24, 48, 72 and 96 h post-stress (). Repeated measures two-way ANOVA showed similar main effects of time and diazepam treatment in the light and dark phases, indicating that diazepam did not attenuate the reduction in fine activity following restraint ().
Figure 4. Diazepam facilitates recovery of locomotor activity following a single episode of two-hour restraint stress. A line graph demonstrating continuous recording of (A) total locomotor activity and (D) fine activity during the dark (grey) and light (white) phase after acute restraint stress exposure in vehicle (green line) and diazepam (blue line) treated mice (n = 8 per group). The standard errors of mean (SEM) was illustrated as dashed green and blue lines. The red vertical line depicts time of restraint. Cumulative 24 h counts in (B) locomotor and (E) fine activity from mice treated with vehicle (green bar) and diazepam (blue bar) were recorded for 96 h following acute restraint stress. Cumulative 24 h measurements for locomotor and fine activity were separated into light and dark phase for 96 h following acute restraint stress (C & F). *p < .05, **p < .01, ***p < .001 when comparing between vehicle and diazepam at each time point; repeated measures two-way ANOVA with Fisher’s LSD test.
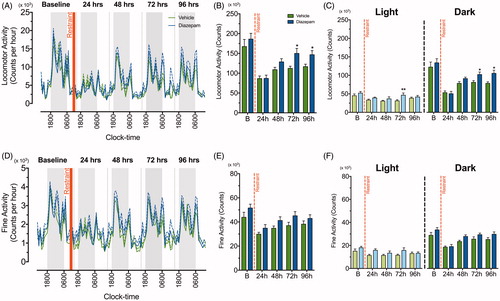
Repeated measures two-way ANOVA revealed significant main effects of diazepam treatment [F (1, 42) = 22.31, p < .0001], time [F (2, 42) = 56.17, p < .0001] and the interaction between these main effects on plasma concentrations of corticosterone [F (2, 42) = 3.32, p = .046]. A post-hoc analysis showed plasma corticosterone concentrations in mice administered diazepam was reduced by approximately 35% (p < .001) and 30% (p < .001) at 1 and 2 h of restraint stress exposure, respectively, compared to the vehicle group (). The repeated measures two-way ANOVA also showed significant main effects of time [F (2, 42) = 105.8, p < .0001] and diazepam treatment [F (1, 42) = 7.18, p = .01], while no significant interaction was observed between time and diazepam treatment on glucose concentrations. Diazepam reduced the acute elevation in blood glucose at 1 h (p < .05), but this was not significantly different by 2 h of stress when compared to vehicle-treated mice (). The effects of diazepam on body weight and food intake were also examined throughout the 96 h post-restraint stress period. While there was no difference in body weight for diazepam- and vehicle-treated mice, facilitation of food intake by diazepam was transiently observed 24 h post-stress when compared to the vehicle-treated mice (data not shown).
Figure 5. Diazepam dampens restraint-evoked plasma corticosterone and blood glucose concentrations following a single episode of two-hour restraint stress. (A) Plasma corticosterone and (B) blood glucose concentrations were determined from serial blood samples collected at baseline, 1 and 2 h of restraint-stress in vehicle (green line) and diazepam (blue line) treated mice. Data are represented as mean ± SEM from eight mice per group; *p < .05, ***p < .001 when comparing between vehicle and diazepam at each time point; repeated measures two-way ANOVA with Fisher’s LSD test.
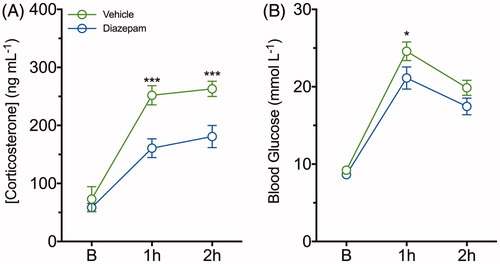
Discussion
In this study, we have demonstrated the ability of an automated phenotyping system to monitor stress-related perturbations in behavior that occur following a single episode of restraint. Using the TSE PhenoMaster, we have identified sustained reductions in basal locomotor activity for up to 96 h following exposure to a single restraint stress. These sustained changes were preferentially observed in the light phase of the light/dark cycle. We also observed delayed facilitation of stress-induced locomotor activity recovery following administration of a single dose of diazepam. Together with the transient changes observed in food and oxygen consumption, this demonstrates that the automated TSE PhenoMaster is capable of detecting subtle behavioral changes in mice beyond the measurable time-frames typically employed in normal experimental procedures.
This study has demonstrated decreased basal locomotor activity up to 96 h following exposure to a single episode of restraint stress. When separated for light/dark phase, transient suppression occurred during the dark phase within the first 48 h, while sustained decreases were observed in the light phase for up to 96 h. This is consistent with previous studies indicating that locomotor activity decreases following exposure to severe stressors, such as restraint (Brodkin et al., Citation2014; Chesworth et al., Citation2012). However, these studies were only limited to 15–30 min of post-stress recording and did not examine persistent changes in activity. Similar to Brodkin et al. (Citation2014), we observed a significant increase in fine activity, which is suggested to represent grooming behavior, in the immediate hour following exposure to restraint (Robinson et al., Citation2013; Urbach et al., Citation2014). However, the cumulative fine activity over the 24–48 h following restraint mirrors the changes observed in total locomotor activity, with the actual counts indicating that both fine and ambulatory activity are reduced. It should be noted that increased fine activity observed in this study could also be representative of relatively immobile risk assessment behaviors. However, video and vibration analysis performed by Brodkin et al. (Citation2014) indicated that many facets of risk assessment behavior including rearing, orienting (little to no body movement with small head movement/sniffing) and immobility were reduced while grooming behavior preferentially increased immediately after stress exposure.
In order to test the sensitivity of the TSE PhenoMaster system to temporal changes in activity we administered a single dose of diazepam and observed an immediate sedative effect of diazepam on locomotor and fine activity. However, this effect was highly transient, differing significantly for only 2 h from mice administered vehicle with no observable difference being recorded in cumulative counts collected over 48 h. It has previously been shown that a single intraperitoneal injection of 1 mg kg−1 diazepam is rapidly absorbed into blood, yielding maximum serum concentrations in approximately 10 min after which decay into active metabolites yields an overall half-life of approximately 22 h in mice. Therefore, it is unlikely that diazepam is unavailable in this immediate 2-h period following administration. When mice were monitored following diazepam administration and subsequent restraint stress, no immediate effects of the drug were observed in the stress-induced reduction of locomotor and fine activity. Interestingly, the time of release from restraint approximately coincides with the duration of time elapsed to produce no significant differences in activity when diazepam was administered in the absence of restraint. Moreover, we observed a facilitated recovery from stress-induced reductions in locomotor activity occurring at 72 and 96 h after stress exposure, an effect that was preferentially observed in the dark phase of the light cycle. It should be noted that the impact of a stressor, such as restraint might initially override the observable effects of diazepam which subsequently become visible as the mouse recovers from stress exposure. Given the complex relationship that exists between benzodiazepines, stress and behavior, further experiments will be required to determine the mechanism underlying this delayed facilitation of locomotor activity recovery.
Underlying behavioral modification in models utilizing stress intervention is the overall increase in HPA axis activity. Using a single exposure to inescapable tail shock, Fleshner et al. (Citation1995) observed an increase in circulating total corticosterone and decreased corticosteroid-binding globulin levels for up to 48 h following shock exposure. Similarly, Tannenbaum et al. (Citation1997) demonstrated that basal HPA axis activity, measured as circulating adrenocorticotropic hormone, free/total corticosterone and corticosteroid binding globulin capacity were altered for up to 48 h and pituitary transcortin binding significantly decreased for up to 72 h following a single 20 min restraint stress. These changes were preferentially observed in the light phase and may relate to the sustained suppression of basal locomotor activity observed during the light phase in this study.
Several studies have also demonstrated that single exposure to stress causes long-lasting changes in HPA axis activity (Armario et al., Citation2004; Dal-Zotto et al., Citation2000, Citation2003; Marti et al., Citation2001; Valles et al., Citation2000). Using different rat models exposed to a single social defeat stressor, Meerlo et al. (Citation1996, Citation1997) demonstrated changes in activity for several days similar to those observed in this study following stress exposure. Calvo et al. (Citation1998) also showed that corticosterone or dexamethasone administration were as effective as a 15-min restraint stress at inducing anxiety-like behavior 24 h later in the elevated plus maze. This effect was attenuated by the corticosterone synthesis inhibitor, metyrapone, indicating the importance of corticosterone in the generation of this behavior.
While diazepam has potent anxiolytic activity, administration alone transiently increases basal corticosterone output. However, subsequent activation of the HPA axis is blunted, resulting in significantly decreased corticosterone responses to stress exposure (Kalman et al., Citation1997; Mikkelsen et al., Citation2005). This is consistent with the results of this study, where corticosterone concentrations were attenuated during restraint stress exposure following pretreatment with diazepam. However, it should be noted that the blunted corticosterone response to restraint might be the result of activated negative feedback on the HPA axis caused by diazepam-induced increases in basal corticosterone. Although Beracochea et al. (Citation2011) have demonstrated diazepam dose-dependently attenuates the stress-induced increase in free corticosterone in the hippocampus, this has not been confirmed at the dose used in this study. We also monitored stress-induced hyperglycemia following administration of diazepam or vehicle as this is a well-documented marker of increased catecholamine and glucocorticoid secretion (Halter et al., Citation1984; Vachon & Moreau, Citation2001). Interestingly, we observed a reduction in the peak glycemic response to restraint following diazepam administration. This is in contrast to Sugimoto et al. (Citation1998) who showed diazepam administered at the same dose as this study had no effect on stress-induced blood glucose concentrations. However, this may be due to their immediate application of stress in place of the 30-min drug equilibration period following administration used in this study.
Following stress exposure there was a transient decrease in food intake and body weight in experimentally naïve animals during the first 24 h. Both Marti et al. (Citation1994) and Valles et al. (Citation2000) have previously observed this decrease in food intake 24 h following restraint or immobilization stress in rats, with the latter study also demonstrating a decrease in body weight gain. However, unlike the transient changes observed in this study, Valles et al. (Citation2000) observed sustained and profound reductions in food intake for up to 8 d and substantial body weight differences lasting the 15 d duration of their experiment. While these differing results may indicate a relative resilience of mice over rats, Marti et al. (Citation2001) also used corticosterone output to demonstrate the severity of immobilization stress in comparison to the restraint method used in this work. In addition to the transient changes in food intake and body weight, the decrease in oxygen consumption observed in this study did not translate to changes in metabolic rate when normalized for body weight and is likely the result of overall decreases in activity during this time period. In contrast, administration of diazepam transiently increased food intake during the first 24 h after stress exposure. Benzodiazepines are known to induce increased food and water intake acutely after administration, an effect considered to be mediated by interaction with the mitochondrial diazepam binding inhibitor receptor responsible for neurosteroidogenesis and consequent GABA-A receptor modulation (Cooper & Estall, Citation1985; Reddy & Kulkarni, Citation1998; Soderpalm & Berridge, Citation2000). However, as the increase in food intake observed in this study was only evident during the first 24 h, it did not translate into lasting changes in body weight.
Conclusions
The automated behavioral monitoring system, TSE PhenoMaster allows the continuous monitoring of basal behavior and physiological parameters concurrently in multiple mice individually housed under undisturbed conditions for a period of weeks. Here, we demonstrated disturbances in basal behaviors for up to 96 h following a single episode of restraint. Moreover, mice administered a single prophylactic dose of a conventional anxiolytic pharmaceutical drug prior to restraint exposure facilitated recovery of stress-induced locomotor reductions, while dampening restraint-evoked increases in plasma corticosterone and blood glucose concentrations. While these systems cannot replace targeted behavioral, endocrine or biochemical testing, automated measures may offer further invaluable insights into the effects of stress on basal behavior and metabolism. Taken together, these results indicate that the use of an automated behavioral monitoring system is capable of identifying mice with irregular behavioral patterns indicative of anxiety and may be a useful tool for screening the effectiveness of pharmaceutical compounds outside of more targeted behavioral testing arenas.
Acknowledgments
We would like to thank Dr. Peter G. Noakes for his support and providing feedback on the manuscript. We would also like to thank the animal technicians from the University of Queensland Biological Resources animal house for animal care and husbandry.
Disclosure statement
The authors declare no competing financial interests. This work was supported by internal funds from The University of Queensland. This funding body had no further role in the study design; in the collection, analysis and interpretation of data; in the writing of the report; and in the decision to submit the article for publication.
Additional information
Funding
References
- Armario A, Marti O, Valles A, Dal-Zotto S, Ons S. (2004). Long-term effects of a single exposure to immobilization on the hypothalamic-pituitary-adrenal axis: neurobiologic mechanisms. Ann N Y Acad Sci 1018:162–72.
- Beery AK, Kaufer D. (2015). Stress, social behavior, and resilience: insights from rodents. Neurobiol Stress 1:116–27.
- Belzung C. (2001). The genetic basis of the pharmacological effects of anxiolytics: a review based on rodent models. Behav Pharmacol 12:451–60.
- Beracochea D, Tronche C, Coutan M, Dorey R, Chauveau F, Pierard C. (2011). Interaction between diazepam and hippocampal corticosterone after acute stress: impact on memory in middle-aged mice. Front Behav Neurosci 5:14.
- Brodkin J, Frank D, Grippo R, Hausfater M, Gulinello M, Achterholt N, Gutzen C. (2014). Validation and implementation of a novel high-throughput behavioral phenotyping instrument for mice. J Neurosci Methods 224:48–57.
- Calvo N, Martijena ID, Molina VA, Volosin M. (1998). Metyrapone pretreatment prevents the behavioral and neurochemical sequelae induced by stress. Brain Res 800:227–35.
- Chen HJC, Spiers JG, Sernia C, Anderson ST, Lavidis NA. (2014). Reactive nitrogen species contribute to the rapid onset of redox changes induced by acute immobilization stress in rats. Stress 17:520–7.
- Chesworth R, Yulyaningsih E, Cappas E, Arnold J, Sainsbury A, Karl T. (2012). The response of neuregulin 1 mutant mice to acute restraint stress. Neurosci Lett 515:82–6.
- Clemmensen C, Finan B, Fischer K, Tom RZ, Legutko B, Sehrer L, Heine D, et al. (2015). Dual melanocortin-4 receptor and GLP-1 receptor agonism amplifies metabolic benefits in diet-induced obese mice. EMBO Mol Med 7:288–98.
- Cooper SJ, Estall LB. (1985). Behavioural pharmacology of food, water and salt intake in relation to drug actions at benzodiazepine receptors. Neurosci Biobehav Rev 9:5–19.
- Dal-Zotto S, Marti O, Armario A. (2000). Influence of single or repeated experience of rats with forced swimming on behavioural and physiological responses to the stressor. Behav Brain Res 114:175–81.
- Dal-Zotto S, Marti O, Armario A. (2003). Glucocorticoids are involved in the long-term effects of a single immobilization stress on the hypothalamic-pituitary-adrenal axis. Psychoneuroendocrinology 28:992–1009.
- Djordjevic J, Cvijic G, Davidovic V. (2003). Different activation of ACTH and corticosterone release in response to various stressors in rats. Physiol Res 52:67–72.
- Engelmann M, Landgraf R, Wotjak CT. (2004). The hypothalamic-neurohypophysial system regulates the hypothalamic-pituitary-adrenal axis under stress: an old concept revisited. Front Neuroendocrinol 25:132–49.
- Fleshner M, Deak T, Spencer RL, Laudenslager ML, Watkins LR, Maier SF. (1995). A long-term increase in basal levels of corticosterone and a decrease in corticosteroid-binding globulin after acute stressor exposure. Endocrinology 136:5336–42.
- Glaser R, Kiecolt-Glaser JK. (2005). Stress-induced immune dysfunction: implications for health. Nat Rev Immunol 5:243–51.
- Greenman Y, Kuperman Y, Drori Y, Asa SL, Navon I, Forkosh O, Gil S, et al. (2013). Postnatal ablation of POMC neurons induces an obese phenotype characterized by decreased food intake and enhanced anxiety-like behavior. Mol Endocrinol 27:1091–102.
- Haller J, Alicki M. (2012). Current animal models of anxiety, anxiety disorders, and anxiolytic drugs. Curr Opin Psychiatry 25:59–64.
- Halter JB, Beard JC, Porte D Jr. (1984). Islet function and stress hyperglycemia: plasma glucose and epinephrine interaction. Am J Physiol 247:E47–52.
- Kalman BA, Kim PJ, Cole MA, Chi MS, Spencer RL. (1997). Diazepam attenuation of restraint stress-induced corticosterone levels is enhanced by prior exposure to repeated restraint. Psychoneuroendocrinology 22:349–60.
- Koo JW, Russo SJ, Ferguson D, Nestler EJ, Duman RS. (2010). Nuclear factor-kappaB is a critical mediator of stress-impaired neurogenesis and depressive behavior. Proc Natl Acad Sci USA 107:2669–74.
- Machado A, Herrera AJ, de Pablos RM, Espinosa-Oliva AM, Sarmiento M, Ayala A, Venero JL, et al. (2014). Chronic stress as a risk factor for Alzheimer’s disease. Rev Neurosci 25:785–804.
- Marti O, Garcia A, Valles A, Harbuz MS, Armario A. (2001). Evidence that a single exposure to aversive stimuli triggers long-lasting effects in the hypothalamus-pituitary-adrenal axis that consolidate with time. Eur J Neurosci 13:129–36.
- Marti O, Marti J, Armario A. (1994). Effects of chronic stress on food intake in rats: influence of stressor intensity and duration of daily exposure. Physiol Behav 55:747–53.
- Meerlo P, Overkamp GJ, Benning MA, Koolhaas JM, Van den Hoofdakker RH. (1996). Long-term changes in open field behaviour following a single social defeat in rats can be reversed by sleep deprivation. Physiol Behav 60:115–19.
- Meerlo P, Overkamp GJ, Koolhaas JM. (1997). Behavioural and physiological consequences of a single social defeat in Roman high- and low-avoidance rats. Psychoneuroendocrinology 22:155–68.
- Mikkelsen JD, Soderman A, Kiss A, Mirza N. (2005). Effects of benzodiazepines receptor agonists on the hypothalamic-pituitary-adrenocortical axis. Eur J Pharmacol 519:223–30.
- Nishimura Y, Hata T, Kawabata A, Itoh E, Kita T. (1989). Impairment of passive avoidance performance in SART-stressed mice and the action of drugs. Jpn J Pharmacol 49:111–17.
- Perez-Nievas BG, Madrigal JL, Garcia-Bueno B, Zoppi S, Leza JC. (2010). Corticosterone basal levels and vulnerability to LPS-induced neuroinflammation in zthe rat brain. Brain Res 1315:159–68.
- Reddy DS, Kulkarni SK. (1998). The role of GABA-A and mitochondrial diazepam-binding inhibitor receptors on the effects of neurosteroids on food intake in mice. Psychopharmacology (Berl) 137:391–400.
- Robinson L, Plano A, Cobb S, Riedel G. (2013). Long-term home cage activity scans reveal lowered exploratory behaviour in symptomatic female Rett mice. Behav Brain Res 250:148–56.
- Soderpalm AH, Berridge KC. (2000). Food intake after diazepam, morphine or muscimol: microinjections in the nucleus accumbens shell. Pharmacol Biochem Behav 66:429–34.
- Sugimoto Y, Yamada J, Noma T. (1998). Effects of anxiolytics, diazepam and tandospirone, on immobilization stress-induced hyperglycemia in mice. Life Sci 63:1221–6.
- Tannenbaum B, Rowe W, Sharma S, Diorio J, Steverman A, Walker M, Meaney MJ. (1997). Dynamic variations in plasma corticosteroid-binding globulin and basal HPA activity following acute stress in adult rats. J Neuroendocrinol 9:163–8.
- Urbach YK, Raber KA, Canneva F, Plank AC, Andreasson T, Ponten H, Kullingsjo J, et al. (2014). Automated phenotyping and advanced data mining exemplified in rats transgenic for Huntington’s disease. J Neurosci Methods 234:38–53.
- Vachon P, Moreau JP. (2001). Serum corticosterone and blood glucose in rats after two jugular vein blood sampling methods: comparison of the stress response. Contemp Top Lab Anim Sci 40:22–4.
- Valles A, Marti O, Garcia A, Armario A. (2000). Single exposure to stressors causes long-lasting, stress-dependent reduction of food intake in rats. Am J Physiol Regul Integr Comp Physiol 279:R1138–44.
- Wingenfeld K, Wolf OT. (2011). HPA axis alterations in mental disorders: impact on memory and its relevance for therapeutic interventions. CNS Neurosci Ther 17:714–22.
- Zhao Y, Wang Z, Dai J, Chen L, Huang Y, Zhan Z. (2012). Beneficial effects of benzodiazepine diazepam on chronic stress-induced impairment of hippocampal structural plasticity and depression-like behavior in mice. Behav Brain Res 228:339–50.
- Zimprich A, Garrett L, Deussing JM, Wotjak CT, Fuchs H, Gailus-Durner V, de Angelis MH, et al. (2014). A robust and reliable non-invasive test for stress responsivity in mice. Front Behav Neurosci 8:125.