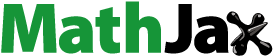
ABSTRACT
Intracranial vascular stent-assisted coil embolization has been widely used for intracranial vascular aneurysm. However, there are serious complication risks, such as thromboembolism, stent migration and spring coil prolapse, due to the inappropriate radial force, poor wall apposition and oversized mesh. Therefore, a locally-reinforced intracranial vascular stent was designed to improve the radial force and wall apposition performance. Based on finite element analysis and substantial measurements, the local radial force has improved 38.9% and thestability of the coil embolism has been enhanced as well. The releasing test in vitro suggests the stent can adhere to the vascular wall very well without the ‘Gator backing’ phenomenon. Furthermore, the implanting test in vivo shows complete endothelialization without restenosis and hyperplasia in 4 weeks. The outcomes of this study provide optimal design of the locally reinforced nitinol stent used for intracranial aneurysm therapy with reduced risks of vascular embolism and haemorrhagic apoplexy after implantation.
Introduction
Intracranial aneurysm is a common cause of haemorrhagic cerebrovascular disease, accounting for 13% of cerebrovascular disease, which mostly occurs in complex and tortuous parts of the blood vessels like the junction of the cerebral arteries with vascular branches, such as the main artery branch point of the circle of Willis [Citation1]. Intracranial vascular stent-assisted coil embolization has become the most effective solution to achieve aneurysm occlusion, especially for intracranial wide-necked, fusiform complex aneurysm lesions (aneurysm neck >4 mm or the ratio of aneurysm sac to aneurysm neck <1.5). Different intravascular stents based on metal materials have been developed to date, such as nanostructured austenitic alloys [Citation2,Citation3], copper-containing austenitic antibacterial stainless steel [Citation4] and nitinols. Among them, nitinols are intensively applied in the manufacturing of vascular stents since they combine high hyperelasticity, good biocompatibility and corrosion resistance. Two kinds of nitinol stents have been in clinical use for spring coil embolism: open-cell stents and closed-cell stents. However, clinical experiences with these stents have confirmed the aneurysm recurrence rate of 12.7% and thrombotic complications rate of 4.5% after stent-assisted coiling treatment [Citation5], which are mainly attributed to the poor administration of the radial force and wall apposition abilities.
Typical closed-cell stents are prone to be oval or crescent, and kinking at the tortuous vessel [Citation6], which results in poor apposition to the vessel wall [Citation7,Citation8] and higher thromboembolic complication rates [Citation9]. Dashti et al. [Citation10] reported that the Enterprise stent with the closed cell structure was displaced after implantation for 4 months, which may also be related to stent malapposition. On the other hand, the open-cell stents have unconnected free ends, which renders better wall apposition to blood vessels; however, the lack of radial force is a critical aspect. In addition, when the stent is located in a tortuous vessel, from a structural point of view, the free ends may cause the mesh to protrude.
For stent-assisted coil embolization in the treatment of intracranial aneurysms, clinical results show that the incidence of in-stent restenosis complications is only 1.28% [Citation11], while the risk of thromboembolism is as high as 4.2% to 17.1% [Citation12], which is attributed to the vascular injury caused by the excessive radial force of the stent. In addition, due to the large mesh or low support force, there is no sufficient supporting to ensure the stents anchoring, resulting in the phenomenon of spring coil prolapse. Therefore, for the treatment of haemorrhagic diseases, the appropriate administration of the radial force from the perspective of structural designing is crucially important.
In order to obtain a proper radial force, Shobayashi et al. designed a closed-cell endovascular stent, which is dominated by a twined strut geometry to reduce the stiffness of the stent, but the wall apposition when contacted with the adjacent vessel wall was not assessed [Citation13]. Li et al. introduced a regular arc structure into the middle of the traditional sinusoidal open-cell stent ring, and the radial strength for the optimised stent increases by approximately 40% [Citation14]. However, the open-cell stent design will inevitably produce problems such as the free ends prolapsing.
As a consequence, a middle-enhanced intracranial vascular stent was designed for assisting coil embolization in the treatment of intracranial aneurysms in this paper. The main body of the stent is composed of an open cell structure to improve the flexibility and wall apposition of the stent. In addition, closed cell structures with different lengths are arranged in the middle part to reduce size of meshes, achieving mechanical enhancement and improving the stability of coil embolism. The local radial force of the stent and its wall apposition ability were evaluated by the finite element analysis (FEA). A series of mechanical properties of the stent were also tested, including three-point bending test, flat plate test, stent crimping test and conveying and releasing test.
Materials and methods
Design of stents
A centrally reinforced hybrid-cell nitinol stent was designed, containing closed cells and open cells (), the former is mainly used to provide better radial load capacity to the stent, and the later is employed to increase the conformability and wall apposition.
Figure 1. (a) Schematic diagram of stent structure, (b) open cell structure, (c) closed cell structure, and (d) the whole stent view.

The strut widths of the open cell and closed cell are 0.03 mm and 0.05 mm (), respectively. The wall thickness of the stent is 0.05 mm. Three closed cell structures were added in different parts of the stent: closed cell 1 (CC1, length-to-width ratio of 1.5) and closed cell 2 (CC2, double diamond structure) were designed to improve the stability of the stent, while closed cell 3 (CC3, length-to-width ratio of 1) was used to reduce the mesh size, achieving mechanical enhancement and improving the stability of spring coil. The open cells account for 30% of the total number of cells, and the distance of the locally reinforced closed cells is 2.25 mm corresponding to the aneurysm length. The stents were fabricated with nitinol tubes (out diameter = 2.8 mm and wall thickness = 100 µm, EUROFLEX GmbH, Pforzheim, Germany) by laser cutting, followed by cleaning, expansion and heat treatment, the stents with the diameter of 4.5 mm and length of 25 mm were fabricated, as shown in .
Material properties for finite element analysis
FEA was used to evaluate the local force in the middle position and wall apposition of intracranial vascular stents, since the built-in user material subroutine is more suitable for modelling of nitinol materials and has good capability to cope with problems involving large nonlinear analysis. In the numerical models, the nitinol stent was assumed to be homogeneous and isotropic. The material properties were obtained from previous work [Citation15] ().
Table 1. Material properties of the nitinol stent (Auricchio model).
Local radial force evaluation
Three stents with different lengths of CC3 structure were designed, abbreviated as CC3A (0 mm), CC3B (2.25 mm) and CC3C (4.75 mm), respectively, as shown in . To verify the mechanical enhancement of different CC3 structure, the three-dimensional models of 4.5 mm diameter stents with different CC3 structures were established by wrapping a 2D sketch of the design around a cylinder. The 3D models of the stents were imported in FEA software. The meshes on all stents are built with C3D8R elements (an 8-node linear brick, reduced integration, hourglass control) of 0.01 mm size.
The local radial force in the middle of the stent was assessed to confirm the effectiveness of the middle reinforcement. In detail, the stent was placed on a flat plate and then the local radial force was measured during compression course. The indenter was withdrawn back to allow the stent recovery after a displacement of 0.7 mm. During the expansion simulation, the indenter was discretized using R3D4 elements (a 4-node 3D bilinear rigid quadrilateral) and the flat plate was discretized by SFM3D4R elements (4-node quadrilateral surface element, reduced integration).
Wall apposition evaluation
Wall apposition describes the ability of implanting stents to remain when contacting closely with the adjacent blood vessel wall. For comparison, a fully closed-cell stent was designed (named Stent-CC) was deployed in a vessel with different diameters, 3.5 mm at one end and 2 mm at the other end. A displacement load was applied to the stent by a rigid clamped shell, pressing the outer diameter to 1.8 mm. The clamped shell was then withdrawn to allow the stent to expand freely and adhere to the wall of the blood vessel. The cross section in the transition segment was obtained to visually observe the apposition of each stent with the vessel wall.
In this model, the mechanical properties of natural blood vessels were simplified to homogeneous and isotropic model, assigning the vessels’ density of 1200 kg/m3, Poisson’s ratio of 0.45 and Young’s modulus of 4 MPa. The meshes on the vessel are built with C3D8R elements. Nlgeom is open during the wall apposition test. A static, large deformation analysis was performed using the default contact algorithm. A ‘surface-to-surface contact’ algorithm was defined between the vessel inter surfaces and the outer surfaces of the stent. The friction in the tangent direction was defined as a penalty function assuming the friction coefficient of 0.5 for all contract surfaces, and ‘Hard contact’ was defined in the normal direction.
Mechanical properties of as-fabricated nitinol stents
Stents fabricated by nitinol tubes were expanded to 4.5 mm. The morphology was observed using scanning electron microscope (SEM, Inspect F50, FEI, Hillsboro, U.S.A), and the chemical compositions were characterized by energy dispersive spectroscopy (EDS).
The radial force of the fabricated stent was analysed using the ‘Flat Plate Test’. The stent was placed between two flat plates, and the radial force was then measured during compression of the two plates. The flat plate was withdrawn back to allow the stent recovery from 50% compression (2.25 mm displacement). The radial force was collected in the period. To establish the relationship between the bending moment and displacement, two lower static supports were displaced in a selected span 20 mm. The upper load applicator sat in the middle point of the span. In detail, the upper load applicator moved down at a speed of 0.05 mm/s then withdrew back to allow recovery of the stent due to the spring-back effect after a displacement of 4.5 mm. The force value was collected in the period, which was multiplied by the length to obtain the bending moment. In addition, in order to further characterize the radial force of the stent, the TTR2 radial force testing machine (Blockwise Engineering LLC, AZ, U.S.A) was used for stent crimping test. During the whole process, the stent was compressed or released along the radial direction, and the radial force of the stent was recorded by the force sensor.
Finally, an in vitro silicone hose model for the evaluation of delivery performance of stents was built, which was composed of hoses with the same inner diameter (3 mm) and different curvature radius (4 mm, 5 mm, 6 mm, respectively). Subsequently, the stent was released into the model through the 2.3 F Prowler® Select Plus microcatheter (0.021 inch inside diameter) and transmission-related properties were investigated, such as delivery, releasing and apposition of the stent.
In vivo stent implantation
Three stents were implanted into the abdominal aorta of three adult male Japanese white rabbits weighing 3–3.5 kg through the delivery device. After 28 days, the test samples were taken and fixed with 10% paraformaldehyde solution at 4°C for 5 days. Then the samples were cut in half. One half was cut longitudinally along the artery direction. After gradient dehydration, the endothelialization degree of the stent was observed by SEM. The other half of stents were embedded in epoxy resin and sliced into 100 μm thin pieces. Haematoxylin-eosin (H&E) staining (Solarbio Science and Technology Co., Ltd., Beijing, China) was performed to observe the new endothelial cell layer. Animal welfare and the experimental design were approved by the Ethics Committee of the Shenyang Research Institute of Chemical Industry, China.
Results
Local radial force of the stent
The local radial force in the middle of the stent is shown in . The reaction force of the indenter subjected to the stent was 0.0162 N (CC3A) < 0.0225 N (CC3B) < 0.0337 N (CC3C), respectively. With the increase of the middle reinforcement length, the force value in the middle of the stent increases, and the enhancement effect is more significant.
Figure 3. Local compression test simulation of stents with different lengths of CC3 structure: A CC3A, B CC3B, CCC3C, D-F enlarged views of the black dashed boxes.

In addition, there are structural differences between the closed cell design and the open cell design. The mesh wires of the closed cell structure are connected, and the force transferring can be realized when compressed, inducing higher resistance to external forces and uniform deformation (). Open cell structures with unconnected mesh, forming units with open ends, provide better flexibility during bending process, but supporting performance is relatively poor. Most importantly, free ends are easy to extend under pressure (red circles in ), thus increasing the risk of thrombosis.
Wall apposition of stents
The closed cell structure Stent-CC and the open cell structure CC3B were simulated to be released into vessels with varying inner diameters, and the FEA results are shown in .
Figure 4. Deployment simulation of stents into vessels with varying inner diameters: (a, b) wall apposition of the stent, (c, d) S11 stress distribution of the stent on the vessel wall, (e, f) S33 stress distribution of the stent on the vessel wall. (a, c, e) Stent-CC, (b, d, f) CC3B stent.

The cross-sectional micrographs of the stent in the transition region of the inner side of the vessel are shown in . It is obvious that the Stent-CC mesh of the closed-cell stent is connected to each other, and the force exerted on the retracted end of the stent is transmitted to the whole stent, and the wall apposition is poor in the transition area of the inner side (, red circle area), forming a horn mouth shape, which may lead to an increased risk of migration. As for the open-cell stent CC3B, typical free ends are attached to the wall at both ends of the transition region because part of the mesh is not connected (indicated by the arrows in ). The stress distribution of different stents acting on the vessel wall in different directions are shown in . It can be seen that the stress on the vessel wall in the S11 direction (radial) of Stent-CC () is significantly higher than that of the open-cell stent CC3B (), mainly concentrated at one end of the small-diameter vessel, indicating that the closed-cell stent at one end of the large-diameter vessel has a weaker interaction with the vessel wall, thus is more likely to migrate. In addition, the stress of the open-cell stent in the S11 direction of the vessel wall is evenly distributed at both ends of the vessel, achieving a good anchoring effect. On the other hand, for the S33 direction (axial), the shear stress on the vessel wall of Stent-CC () is also higher than that of the open-cell stent CC3B (). As such, the difference of the diameter at the position of distal and proximal vessels may exert a compressive force on the stent, driving it to move to a more stable position, resulting in the migration of the stent into the vessel with larger diameter.
Characterization of the as-fabricated stents
Based on the simulation results, the middle-enhanced stent with the length of 25.5 mm was processed, and the size of the CC3 cell is about 2.25 mm (), consists of both open cell and closed cell structures (), which are separated from each other by rounded transitions (), thus reduces stress concentration and avoids damage to vessels due to the sharpness of the stent. Fifteen positions of the stent were selected randomly for measurement and verification, the width of the 12 periodic V-shaped unit mesh was 0.037 ± 0.003 mm, the width of the 8 periodic V-shaped unit mesh was 0.050 ± 0.003 mm, and the wall thickness was 0.054 ± 0.003 mm. EDS analysis confirmed that nitinol alloy scaffold consisted of 50.81 atomic percentages of Ti and 49.19 of Ni ().
Figure 5. Dimension verification and composition of laser-cut stents: (a) the whole stent image, (b, c) structural connecting rod, (d) open cell structure of the stent, (e) closed cell structure of the stent, (f) the chemical composition of the stent measured by EDS.

Finally, the as-fabricated stent was tested to evaluate mechanical properties, as shown in . The local radial force of the stent at a displacement of 0.7 mm is 0.0225 N (), which corresponds well to the simulation results. When compressed to 50% of the stent’s nominal diameter (2.25 mm), this value increase to 0.086 N, much higher than the radial force value (a range of 0.008–0.026 N) of other aneurysm stents as reported [Citation16–18]. Through the three-point bending test (), the final bending moment of the bracket was calculated to be 0.148 N·mm. Meanwhile, the radial force was 0.0161 N/mm by flat plate test (). In addition, the chronic abduction force of the stent obtained from stent crimping test was about 1.03 N (the position of the red dot in ).
Figure 6. Mechanical properties of the as-fabricated stent: (a) the relationship between the local radial force and displacement, (b) the relationship between the bending moment and displacement, (c) the relationship between the radial force per unit length and displacement, (d) the relationship between the chronic outward force and diameters.

The results of stent releasing into different models in vitro are shown in . The stents were released into silicone hoses with a centerline radius of 4 mm, 5 mm, and 6 mm, respectively (). It is obvious that during the releasing processes with different centerline radii, the stents could be successfully delivered and released through the microcatheter, showing good wall apposition and excellent compliance. After that, the stent was further released into a silicone aneurysm vascular model with an inner diameter of 4 mm (). For the tortuous vessel with aneurysm (, the aneurysm sac is about 9.1 mm wide, the aneurysm neck is about 7.5 mm, the ratio of above two is about 1.21, and the diameter of the aneurysm vessel curvature circle is 5 mm), good wall apposition for the stent can be observed, most important, at the neck of the tumour, no ‘Gator backing’ phenomenon at the free end of the open cell appeared.
In vivo animal implantation experiment
The rabbit abdominal artery model was used to evaluate the stent implantation in vivo for 4 weeks. The H&E staining showed that a thin layer of endothelial cells formed and wrapped the whole stent post surgery for 4 weeks, and there was no restenosis and hyperplasia (). Blood vessel tissue covered on the stent was further examined by SEM. It can be seen that the stent struts were wrapped in the middle layer of blood vessels, which has been almost completely healed, as shown in [Citation19–21].
Discussion
Intracranial aneurysms are generally found in complex and tortuous parts of the blood vessels, often accompanied by branching, such as the junction of the cerebral arteries. Many clinical studies have shown that closed-cell stents have a higher risk of spontaneous delayed migration [Citation22–25], especially for the treatment of basilar artery apical aneurysms, where the stent extends from the basilar artery to the posterior cerebral artery (PCA). When stents implanting, the difference in the inner diameter of the vessel is about 1–1.5 mm, which is considered to be the main reason for the spontaneous delayed migration of the stent. Compared with the closed-cell stents, the open-cell stents can provide better adherence when implanted in blood vessels with varying inner diameters owing to the unconnected free ends. Therefore, the open cell structures are preferred for the auxiliary coil embolization stents for intracranial aneurysm.
However, when the open-cell stent is located in a tortuous vessel, its free ends may cause the mesh to protrude, forming a ‘Gator backing’ at the neck of the aneurysm [Citation16], which might cause coil prolapse. Yang et al. established linear theoretical model to estimate mechanical properties of stents with rhombus cells under specific loading conditions [Citation15]. According to their algorithm, the open cell structure resembles a spring with less stability, while the closed cell structure is closer to a truss, where the loading can be distributed evenly and thus providing the higher radial force. Additionally, most intracranial aneurysms range from 2.1 to 9.3 mm in width, and thus the appropriate enhanced length for stents are required [Citation26]. Therefore, setting a 2.25 mm length single diamond cell CC3 structure with a length-to-width ratio of 1 in the middle part of the stent can be expected to improve the local radial force, and with the mesh size decreasing, the stability of the coil embolism is enhanced. In addition, although the open-cell stents showed good adherence and anchoring with little migration, Broadbent et al. [Citation27] found that the open-cell stents may migrate due to less radial force. Therefore, closed cell structures CC1 and CC2 were added to the stents in this study, which can further improve the stability of the stents and avoid migrating risk.
Previous studies have shown that the wire width, wall thickness and mesh size of the stent have great impact on the radial force [Citation14,Citation28–30]. It is well known that too thick the stent wire will lead to restenosis, while too thin the stent wire cannot provide appropriate radial force. Therefore, based on our previous work [Citation31], the strut widths of the open cells and closed cells are set as 0.03 mm and 0.05 mm, respectively. In addition, the cell shape also influences the radial force. Karnessis et al. designed different cellular structures, including inverted hexagonal honeycomb, V-type and chiral auxetic structures, the results demonstrated that the adoption of auxetic tubular structures, through appropriate designing, can contribute to extending considerably the curvature that the tube can reach before undergoing kinking [Citation32]. The open-cell stent with rhombus/diamond structure has good structural stability, but the wall-apposition and bending performance are slightly poor. Therefore, an uneven design with different functions for the stent is adopted in this work, that is to say the main body is composed of an open cell structure, and a closed cell structure with different lengths arranged in the middle parts, as such, a middle-enhanced intracranial vascular stent for assisting coil embolization in the treatment of intracranial aneurysms is obtained.
The local radial force test shows that the closed cell structure indeed improves the local compression resistance of the stent, and increases the stability of the coil. According to the feedback force value of the three-point bending test, the final bending moment of the stent after processing is 0.148 N mm, which is superior to other commercial intracranial vascular stents. The radial force obtained from the flat plate test was 0.0161 N/mm, which was much higher than the performance of commercially available stents (0.0065–0.0116 N/mm [Citation16]). Therefore, the newly designed stent has good local compression resistance at the neck of the aneurysm and appropriate wall apposition as well.
Conclusion
Based on the FEA, a locally reinforced intracranial vascular stent for assisted coil embolization in the treatment of intracranial aneurysms was designed with the appropriate radial force and good wall apposition. The main body of the stent was composed of an open cell structure, and CC3 closed cell structures with different lengths were set in the middle part to reduce the mesh size. The local radial force increased from 0.0162 N (CC3A) to 0.0225 N (CC3B), and the stability of the coil embolism was enhanced as well. The releasing test in vitro suggested that the stent can adhere to the vascular wall very well, without the ‘Gator backing’ phenomenon at the free end of the open cell. Complete endothelialization of the stent was observed after in vivo implantation of the stent for 4 weeks.
Acknowledgments
This work was supported by the National Key Research and Development Program (2018YFC1105503). We also thank Chen Liu and Nina An in Shenyang Research Institute of Chemical Industry for helping complete animal experiments. The FEA using Abaqus (SIMULIA Inc., U.S.A.) were provided by National Supercomputing Center in Shen Zhen, China.
Disclosure statement
No potential conflict of interest was reported by the authors.
Additional information
Funding
References
- Macdonald RL, Schweizer TA. Spontaneous subarachnoid haemorrhage. Lancet. 2017;389(10069):655–10.
- Misra RDK, Challa VSA, Injeti VSY. Phase reversion-induced nanostructured austenitic alloys: an overview. Mater Technol. 2022;37(7):437–449.
- Nune KC, Misra RDK. Biological activity of nanostructured metallic materials for biomedical applications. Mater Technol. 2016;31(13):772–781.
- Luo F, Tang Z, Xiao S, et al. Study on properties of copper-containing austenitic antibacterial stainless steel. Mater Technol. 2019;34(9):525–533.
- Phan K, Huo YR, Jia F, et al. Meta-analysis of stent-assisted coiling versus coiling-only for the treatment of intracranial aneurysms. J Clin Neurosci. 2016;31:15–22.
- Heller RS, Miele WR, Do-Dai DD, et al. Crescent sign on magnetic resonance angiography revealing incomplete stent apposition: correlation with diffusion-weighted changes in stent-mediated coil embolization of aneurysms. J Neurosurg. 2011;115(3):624–632. DOI:10.3171/2011.4.JNS102050
- Heller RS, Malek AM. Parent vessel size and curvature strongly influence risk of incomplete stent apposition in enterprise intracranial aneurysm stent coiling. Am J Neuroradiol. 2011;32(9):1714–1720.
- Ebrahimi N, Claus B, Lee CY, et al. Stent conformity in curved vascular models with simulated aneurysm necks using flat-panel CT: an in vitro study. Am J Neuroradiol. 2007;28(5):823–829.
- Kadkhodayan Y, Rhodes N, Blackburn S, et al. Comparison of enterprise with neuroform stent-assisted coiling of intracranial aneurysms. Am J Roentgenol. 2013;200(4):872–878. DOI:10.2214/AJR.12.8954
- Dashti SR, Fiorella D, Toledo MM, et al. Proximal migration and compaction of an enterprise stent into a coiled basilar apex aneurysm: a posterior circulation phenomenon? J Neurointerv Surg. 2010;2(4):356–358. DOI:10.1136/jnis.2010.002444
- Goyal RK, Kato Y, Kawase T, et al. Comparative outcome analysis of enterprise and neuroform stent-assisted coiling of cerebral aneurysms: a review of the literature. Asian J Neurosurg. 2020;15(1):4–9. DOI:10.4103/ajns.AJNS_284_19
- Kocur D, Slusarczyk W, Przybylko N, et al. Stent-assisted endovascular treatment of anterior communicating artery aneurysms. Literature Review, Pol J Radiol. 2016;81:374–379.
- Shobayashi Y, Tateshima S, Tanishita K. Load-dispersing design with twined-spring geometry of a distensible intracranial stent for cerebral aneurysms. J Biorheology. 2012;26(1–2):29–37.
- Li Y, Wang Y, Shen Z, et al. A biodegradable magnesium alloy vascular stent structure: design, optimisation and evaluation. Acta Biomater. 2022;142:402–412.
- Yang H, Fortier A, Horne K, et al. Investigation of stent implant mechanics using linear analytical and computational approach. Cardiovasc Eng Techn. 2017;8(1):81–90. DOI:10.1007/s13239-017-0295-0
- Krischek O, Miloslavski E, Fischer S, et al. A comparison of functional and physical properties of self-expanding intracranial stents [Neuroform3, Wingspan, Solitaire, Leo+, Enterprise. Minim Invasive Neurosurg. 2011;54(1):21–28. DOI:10.1055/s-0031-1271681
- Cho SH, Jo WI, Jo YE, et al. Bench-top comparison of physical properties of 4 commercially-available self-expanding intracranial stents. J Neurointerv Surg. 2017;12(1):31–39. DOI:10.5469/neuroint.2017.12.1.31
- Arokiaraj MC, De Santis G, De Beule M, et al. Finite element modeling of a novel self-expanding endovascular stent method in treatment of aortic aneurysms. Sci Rep. 2014;4(1):3630. DOI:10.1038/srep03630
- Ishiwata S, Tukada T, Nakanishi S, et al. Postangioplasty restenosis: platelet activation and the coagulation-fibrinolysis system as possible factors in the pathogenesis of restenosis. Am Heart J. 1997;133(4):387–392. DOI:10.1016/S0002-8703(97)70178-9
- Liu T, Liu S, Zhang K, et al. Endothelialization of implanted cardiovascular biomaterial surfaces: the development from in vitro to in vivo. J Biomed Mater Res Part A. 2014;102(10):3754–3772. DOI:10.1002/jbm.a.35025
- He Y, Chang Y, Hower JC, et al. Origin of repulsive force and structure/dynamics of interfacial water in OEG-protein interactions: a molecular simulation study. Phys Chem Chem Phys. 2008;10(36):5539–5544. DOI:10.1039/b807129b
- Lobotesis K, Gholkar A, Jayakrishnan V. Early migration of a self expanding intracranial stent: case report. Neurosurgery. 2010;67(2):E516–517.
- Kelly ME, Turner RDT, Moskowitz SI, et al. Delayed migration of a self-expanding intracranial microstent. Am J Neuroradiol. 2008;29(10):1959–1960. DOI:10.3174/ajnr.A1224
- Lavine SD, Meyers PM, Connolly ES, et al. Spontaneous delayed proximal migration of enterprise stent after staged treatment of wide-necked basilar aneurysm: technical case report. Neurosurgery. 2009;64(5):E1012. DOI:10.1227/01.NEU.0000343745.18753.D6
- Khatri R, Rodriguez GJ, Siddiq F, et al. Early migration of a self-expanding intracranial stent after the treatment of a basilar trunk aneurysm: report of a second case. Neurosurgery. 2011;69(2):E513–515. DOI:10.1227/NEU.0b013e3182242bfd
- Beck J, Rohde S, Berkefeld J, et al. Size and location of ruptured and unruptured intracranial aneurysms measured by 3-dimensional rotational angiography. Surg Neurol. 2006;65(1):18–25. DOI:10.1016/j.surneu.2005.05.019
- Broadbent LP, Moran CJ, Cross DT, et al. Management of neuroform stent dislodgement and misplacement. Am J Neuroradiol. 2003;24(9):1819–1822.
- Garcia A, Pena E, Martinez MA. Influence of geometrical parameters on radial force during self-expanding stent deployment. Application for a variable radial stiffness stent. J Mech Behav Biomed. 2012;10:166–175.
- Kim DB, Choi H, Joo SM, et al. A comparative reliability and performance study of different stent designs in terms of mechanical properties: foreshortening, recoil, radial force, and flexibility. Artif Organs. 2013;37(4):368–379. DOI:10.1111/aor.12001
- Kumar GV, Mathew L. Effects of design parameters on the radial force of percutaneous aortic valve stents. Cardiovasc Revascula. 2010;11(2):101–104.
- Yan Y, Li N, Guo F, et al. Fabrication of intracranial vascular nitinol alloy stents with improved mechanical property and endothelialization function. Acta Metall Sin-EngL. 2022;35(12):2069–2081. DOI:10.1007/s40195-022-01435-1
- Karnessis N, Burriesci G. Uniaxial and buckling mechanical response of auxetic cellular tubes. Smart Mater Struct. 2013;22(8):084008.